Abstract
The bacteria of the genus Streptomyces are the most valuable source of natural products of industrial and medical importance. A recent explosion of Streptomyces genome sequence data has revealed the enormous genetic potential of new biologically active compounds, although many of them are silent under laboratory conditions. Efficient and stable manipulation of the genome is necessary to induce their production. Comprehensive studies in the past have led to a large and versatile collection of molecular biology tools for gene manipulation of Streptomyces, including various replicative plasmids. However, biotechnological applications of these bacteria require stable genome alterations/mutations. To accomplish such stable genome editing, two major strategies for streptomycetes have been developed: (1) integration into the chromosome through Att/Int site-specific integration systems based on Streptomyces actinophages (ΦC31, ΦBT1, VWB, TG1, SV1, R4, ΦJoe, μ1/6) or pSAM2 integrative plasmid; (2) integration by homologous recombination using suicidal non-replicating vectors. The present review is an attempt to provide a comprehensive summary of both approaches for stable genomic engineering and to outline recent advances in these strategies, such as CRISPR/Cas9, which have successfully manipulated Streptomyces strains to improve their biotechnological properties and increase production of natural or new gene-manipulated biologically active compounds.
Similar content being viewed by others
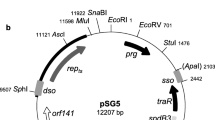
Avoid common mistakes on your manuscript.
Introduction
The genus Streptomyces belongs to one of the largest bacterial phylum Actinobacteria, which are represented by Gram-positive bacteria with high G + C DNA content. In general, Streptomyces species are saprophytic soil-dwelling aerobic filamentous bacteria that play a key role in soil ecology by effectively hydrolyzing a wide range of polysaccharides (cellulose, chitin, xylan, and agar) and other natural macromolecules. This biodegradation efficiency is based on the secretion of potent enzymes such as cellulases, xylanases, and chitinases. Streptomycetes undergo a complex process of morphological differentiation, somewhat resembling that of eukaryotic fungi. In the presence of water and nutrients, their life cycle begins with germination of the spores to form hyphae that grow by tip extension and branching to form a vegetative substrate mycelium. In response to a variety of signals, special reproductive aerial hyphae, similarly growing by tip extension, emerge from the mycelium. Upon cessation of their growth, the aerial mycelium undergoes septation into unigenomic prespore compartments, which finally mature into ovoid spore chains that allow survival and dispersal. This process of morphological differentiation is accompanied and associated with the so-called physiological differentiation, which represents the production of biologically active secondary metabolites, many of which have antibacterial, antifungal, herbicidal, immunosuppresive, antihelminthic, and antitumor properties. Actinobacteria produce about two-thirds of all known antibiotics and other biologically active secondary metabolites, most of which are produced by the Streptomyces genus (Barka et al. 2016; Dyson 2011). However, these bacteria with versatile metabolism are also effective suppliers of many enzymes for biotechnological applications (Spasic et al. 2018). In addition, some Streptomyces strains, such as S. lividans, have been used to efficiently secrete heterologous proteins directly into growth media and are considered valuable hosts for the production of heterologous proteins (Anne et al. 2012; Hamed et al. 2018). So, the bacteria of the genus Streptomyces are clearly of great importance in the field of biotechnology and medicine.
Secondary metabolites are synthesized by complex biosynthetic pathways of subsequent chemical reactions, resulting in a final biologically active product that is specifically exported outside the cell. Biosynthetic genes of secondary metabolites are physically clustered together with other genes encoding regulatory and resistance proteins. This clustering facilitates cloning of entire gene clusters for secondary metabolites into various vectors for production in heterologous hosts and genetic manipulations. Previously, most Streptomyces strains were thought to produce one or only a few different bioactive secondary metabolites. However, robust genomic sequencing of Streptomyces strains revealed a wealth of biosynthetic gene clusters (from 20 to 50) for unknown secondary metabolites. However, only a small proportion of those metabolites are produced under laboratory controlled conditions. The remaining “silent” gene clusters represent a pool of novel metabolites that await “awakening.” Streptomyces strains therefore possess a great potential for synthesis of new compounds. In order to awaken these silent clusters or to increase the production of secondary metabolites, efficient and stable gene manipulation techniques are required for deleting specific genes/gene clusters or for inserting heterologous genes/gene clusters into Streptomyces chromosomes (Baltz 2016; Gross 2007; Chen et al. 2016; Li et al. 2017b). Similarly, heterologous high-level production of recombinant proteins in streptomycetes requires stable genetic manipulations of biotechnologically productive strains (Hamed et al. 2018).
The aim of this review is to summarize approaches for preparing stable genome alterations/mutations in Streptomyces species. However, in many cases, they could also be applied to other actinomycetes. Although a number of reviews have already been published on parts of this subject (Baltz 2012; Deng et al. 2017; Myronovskyi and Luzhetskyy 2013; Siegl and Luzhetskyy 2012), we have described stable genome alterations/deletions with emphasis on new approaches, including our recently published effective system for stable markerless genome alterations (Rezuchova et al. 2018), to create stable Streptomyces strains useful for biotechnology applications.
Genetic manipulations of Streptomyces
The enormous biotechnological potential of Streptomyces strains, as mentioned above, logically provoked great interest in the genetic manipulations of these bacteria. More than fifty years of genetic and molecular biology studies in Streptomyces have brought a comprehensive and versatile collection of molecular biology tools for the gene manipulations of these bacteria, many of which are also useful for biotechnology applications (Hopwood 2007; Kieser et al. 2000).
Unlike efficient transformation systems in Gram-negative bacteria such as Escherichia coli, incorporation of DNA into streptomycetes is difficult due to strong cell wall and restriction modification systems. Nevertheless, several procedures have been developed to introduce DNA to these bacteria. The first used is protoplast transformation. This method, originally developed by Okanishi et al. (1974), was later modified to enhance the efficiency of transformation by the addition of polyethylene glycol (Bibb et al. 1978). Although this procedure has been adapted to a variety of Streptomyces species, this procedure is rather laborious and needs to develop specific conditions for the formation and regeneration of protoplasts in various Streptomyces strains (Kieser et al. 2000; Kormanec et al. 1993). Later, electroporation was developed to introduce plasmid DNA directly into mycelial fragments of some Streptomyces species without the need for protoplast preparation (Pigac and Schrempf 1995). Another and currently the most widely used strategy is the introduction of DNA into Streptomyces strains by easy and efficient conjugation from Escherichia coli. This method allows for the transfer of plasmids containing cis-acting origin of transfer oriT of a broad-host-range IncP-group plasmid RK2 from E. coli donor strains containing the functions of transfer RP4 (IncP) delivered in trans into the Streptomyces strains as a recipient. Conjugation is usually much more effective than protoplast transformation and is applicable to many Streptomyces strains. In addition, it allows easy cloning in E. coli and transfer of finished constructs transferred to Streptomyces only by mixing the donor E. coli with spores of the Streptomyces acceptor strain (Bierman et al. 1992; Mazodier et al. 1989). The main limitation of these methods is that many streptomycetes possess restriction-modification systems that can drastically reduce transformation efficiency. In some strains, they can be circumvented by the use of methylation-deficient E. coli hosts for conjugation and for DNA isolation for transformation, or by heat treatment of protoplasts prior to transformation. The frequency of transformation can also be increased by using single-stranded plasmid DNA. In this regard, conjugation is more efficient because restriction barriers can be circumvented by the transfer of single-stranded concatemers of plasmid DNA during the process (Kieser et al. 2000).
Numerous vector systems are available for genetic manipulation of Streptomyces, including replicative vectors containing the replication origin of Streptomyces, integrating vectors that site-specifically integrate into the genome using bacteriophage or plasmid integrase systems, and suicidal non-replicating vectors that can integrate into the Streptomyces genome by homologous recombination (Kieser et al. 2000). The replicative vectors used for genetic manipulations in streptomycetes were mainly constructed on the basis of four native Streptomyces plasmids: pIJ101, pJV1, pSG5, and SCP2. The high-copy number plasmid pIJ101 with 8830 bp from S. lividans ISP5434 occurs at approximately 300 copies per chromosome (Kieser et al. 1982). It was used for the construction of the most popular non-transmissible replicative cloning vectors pIJ486 and pIJ702 (Kieser et al. 2000). However, the major limitation of these Streptomyces replicative plasmids is that they do not contain E. coli replication origin. Thus, gene manipulations must be performed in S. lividans, which is rather complicated. In addition, they can only be transferred to streptomycetes by laborious protoplast transformation. Although several pIJ101-E. coli shuttle plasmid vectors have been constructed, these are structurally unstable (Kieser et al. 2000; J. Kormanec, unpublished data). Another high-copy number plasmid pJV1 of 11,143 bp from S. phaeochromogenes occurs at approximately 150 copies per chromosome and was also used to construct several Streptomyces replicative cloning vectors (Bailey et al. 1986). In addition, it was used to construct stable and versatile Streptomyces–E. coli shuttle cosmid vector pFD666 (Denis and Brzezinski 1992). The medium copy number (20–50 copies per chromosome) plasmid pSG5 of 12,208 bp from S. ghanaensis 5/1B has temperature-sensitive replication (stops above 34 °C) and was used to construct temperature-sensitive plasmid vectors for gene disruptions (Muth et al. 1989). The low-copy number plasmid SCP2 of 31 kb from S. coelicolor (Bibb et al. 1977) was used to construct several stable low-copy number vectors (e.g., pIJ916, pIJ940), including stable Streptomyces–E. coli cloning shuttle vectors pIJ698 (Kieser et al. 2000), pKC1218, and pOJ446 (Bierman et al. 1992). Based on many years of experience, there are several most useful antibiotic resistance marker genes used for selection of the plasmids in Streptomyces. One of the first used is the thiostrepton resistance tsr gene of S. azureus, but thiostrepton partially inhibits morphological differentiation and interferes also with antibiotic production. One of the most useful resistance markers is the aac(3)IV gene of Klebsiella pneumoniae, encoding aminoglycoside acetyltransferase inactivating apramycin, which has the advantage of selecting plasmids in both E. coli and Streptomyces. The same applies to the neo gene encoding aminoglycoside phosphotransferase inactivating neomycin, kanamycin, and gentamicin from the E. coli transposon Tn5 and the hyg gene of S. hygroscopicus, encoding hygromycin phosphotransferase (Kieser et al. 2000). Detailed genetic manipulation protocols of Streptomyces are described in Kieser et al. (2000) and Rebets et al. (2017).
Streptomyces stable genome alterations/mutations
A major drawback of the Streptomyces replicative plasmids is that their host range is usually restricted to native Streptomyces strains and their closest relatives. In addition, they need a permanent antibiotic selection for stable maintenance in streptomycetes (Kieser et al. 2000). This complicates their use in biotechnology applications. Despite these disadvantages, several replicative plasmids have been successfully used, especially for their high-copy number, for overproduction of many biotechnologically relevant heterologous proteins in streptomycetes (Anne et al. 2012). However, these stability and selection problems can be overcome by using two other types of vectors: (i) integrating vectors containing a DNA segment encoding attachment/integration functions (Att/Int) for site-specific integration into specific sites of the Streptomyces genome (an attB site for specific integrase), (ii) suicidal non-replicating vectors that integrate into any neutral genome region by homologous recombination. In addition, the non-replicating vectors can also be used for stable deletion of genes or whole gene clusters. The main advantage of these vectors is their stability, especially in the introduction of large DNA fragments covering whole gene clusters for secondary metabolites that range from several kb to more than 100 kb. They can be used to awaken “silent” gene clusters for new secondary metabolites, by deleting cluster-situated negative regulatory genes or by inserting strong promoters upstream of cluster-situated positive regulatory genes. They may also facilitate the heterologous production of new “silent” biologically active secondary metabolites or allow increased yields of natural compounds originally produced in low amount in their natural hosts. In addition, they can also functionally decipher huge metagenome data related to gene clusters for new secondary metabolites found by genome mining. Since antibiotic production could also be limited by the availability of precursors, the deletion of relevant biosynthetic genes or their genetically manipulated overexpression could also help in this regard (Bachmann et al. 2014; Baltz 2016; Bekiesch et al. 2016; Huo et al. 2019; Li et al. 2017b; Nah et al. 2017). Similarly, they have great potential for stable heterologous high-level production of recombinant proteins in streptomycetes (Hamed et al. 2018). Another advantage of these two types of vectors is that they are bifunctional and facilitate the engineering of genes/gene clusters in E. coli, followed by conjugation to streptomycetes. Both types of vectors, their advantages, their disadvantages, and their use in several applications are described in the following part of the paper.
Att/Int site-specific integrative vectors
To date, at least eight site-specific integration systems have been characterized and used to construct integration vectors in streptomycetes. Most of them are based on various actinomycete temperate bacteriophages (actinophages) identified in Streptomyces species, including ΦC31 (Lomovskaya et al. 1972, 1980), ΦBT1 (Gregory et al. 2003), VWB (Van Mellaert et al. 1998), TG1 (Morita et al. 2009), SV1 (Fayed et al. 2014), R4 (Chater and Carter 1979), ΦJoe (Fogg et al. 2017), and Φ1/6 (Farkasovska and Godany 2012). Another is based on the natural integrative plasmid pSAM2 found in S. ambofaciens (Boccard et al. 1989; Kuhstoss et al. 1989). Temperate bacteriophages (and integration vectors prepared using their functional elements) are integrated into the host chromosome at a specific site by a recombination process that requires specialized attachment sites, attP in the phage (or in the integration vector) and attB in the host chromosome. Both have 40 to 50 bp in size with different inverted repeat sequences flanking the 2- to 12-bp complementary core sequence that includes the recombination crossover point. This single DNA crossover between these two attachment sites is catalyzed by phage-encoded integrase and generates two junction sites, attL and attR, consisting of hybrid attP and attB sequences (Fig. 1). There are two evolutionary distinct families of site-specific recombinases distinguishable by sequence similarity and mechanism of action that contain a specific catalytic amino acid residue: tyrosine and serine recombinases (Fogg et al. 2014). Interestingly, actinophage integrases belong to both families. The natural integration plasmid pSAM2 has a similar recombination system but with a 26-bp-long attB site and a large 360-bp attP site (Raynal et al. 2002). Most of these integrases are unidirectional and no additional factors are needed for the integration process. Since the excision reaction in which attL and attR are recombined to regenerate attB and attP can occur only in the presence of recombination directionality factor (RDF) or Xis, integration vectors containing only the attP site together with the respective integrase gene remain stably integrated (Khaleel et al. 2011). Therefore, these bacteriophage (or pSAM2-based) attachment/integration (Att/Int) systems (the attP site together with the respective integrase gene) are widely used to develop various integration vectors for stable genetic engineering in Streptomyces. Some of these Att/Int systems have already been reviewed (Baltz 2012; Myronovskyi and Luzhetskyy 2013). The following sections briefly describe individual Att/Int systems and their use for stable Streptomyces gene manipulations and update new information for these systems.
Scheme of site-specific integration of the integration plasmid containing the biosynthetic gene cluster (pink arrows as an example) into the Streptomyces chromosome. int, integrase gene (dark blue arrow); oriT, origin of conjugal transfer; aac(3)IV, the apramycin resistance marker gene used for selection as an example (green arrow)
ΦC31 Att/Int system
Integration plasmids derived from bacteriophage ΦC31, isolated from S. coelicolor (Lomovskaya et al. 1972, 1980), represent the most common integration vectors used in Streptomyces. As mentioned above, only the attP and int gene encoding serine family recombinase are essential for unidirectional site-specific integration. ΦC31-based vectors integrate into the attB site (Table 1) located in the pirin-like gene (SCO3798 in S. coelicolor) encoding a pirin homolog that is highly conserved in prokaryotes and eukaryotes. However, after a deletion of the main attB site, the vectors can also integrate with dramatically reduced efficacy into pseudo-attB sites (Baltz 2012; Combes et al. 2002).
The att/int ΦC31 locus was used to construct several convenient Att/Int integration vectors for use in a broad range of Streptomyces species. One of the first developed and widely used were the conjugative integration plasmid pSET152 and the cosmid vectors pOJ436 and pOJ444, each containing an RK2 replicon oriT for easy conjugal transfer from E. coli to Streptomyces species (Bierman et al. 1992). Both plasmid and cosmid vectors have a cloning capacity of about 15 and 45 kb, respectively. Many ΦC31-based Att/Int cosmid libraries from various Streptomyces strains have been successfully constructed using these cosmid vectors or derivatives thereof to clone biosynthetic gene clusters (e.g., for daptomycin, nikkomycin, moenomycin A, chlorobiocin, novobiocin, coumermycin A1, caprazamycins) and to express them in heterologous Streptomyces strains (Myronovskyi and Luzhetskyy 2013). However, the size of many gene clusters for bioactive secondary metabolites is too large (greater than 100 kb) to fit within the cloning capacity of these cosmid vectors. To solve this problem, two ΦC31-based Att/Int vectors were constructed: bacterial artificial chromosome (BAC) vector pStreptoBAC V based on E. coli F factor and pEASAV13 vector derived from P1 artificial chromosome (PAC). Both can carry DNA inserts of 100–300 kb. Both contain the RK2 oriT replicon for conjugal transfer from E. coli to Streptomyces and have been successfully used to construct libraries from various Streptomyces strains and for subsequent heterologous expression of biosynthetic gene clusters for daptomycin, iso-migrastatin, roseoflavin, FK506, salinomycin, anthracinomycin, chaxamycin, symocyclinone, azalomycin, and vancoresmycin (Tocchetti et al. 2018). Another system for cloning large gene clusters is represented by the ΦC31-based Att/Int integration vector pTARa. It utilizes a useful transformation-associated recombination (TAR) system of the yeast Saccharomyces cerevisiae for cloning and reassembling overlapping DNA fragments to combine the whole biosynthetic gene clusters (Kim et al. 2010). This system has recently been used for cloning and efficient heterologous expression of the metagenomic biosynthetic gene cluster for new calcium-dependent antibiotic malacidins, which acts against multidrug-resistant Gram-positive pathogens (Hover et al. 2018). Two other TAR-based ΦC31 Att/Int integration vectors, pCAP01 and pCAP03, have also been successfully used for cloning and heterologous expression of biosynthetic gene clusters for antibiotics marinopyrrole, taromycin A, enterocin, nataxozole, ammosamides A–C, thiolactomycin, and tiotetroamide (Tocchetti et al. 2018).
The insertion of Att/Int integrative vectors into the ΦC31 attB site of several Streptomyces strains has been shown to be neutral in most cases in terms of individual secondary metabolite production. However, the insertion of large inserts significantly reduced this production. Similarly, insertion of the tylF gene encoding a macrocin 0-methyltransferase into S. fradiue using ΦC31-based Att/Int vectors to increase tylosin production resulted in its decreased yield (Baltz 1998). The reported reductions in antibiotic synthesis could be due to insertional mutagenesis into a pirin-like gene. Indeed, a recent report revealed that a significant decrease in spiramycin production following insertion inactivation of the pirin-like pirA gene using the ΦC31 Att/Int system-based vector in S. ambofaciens ATCC 23877 was due to the marked effects of this pirA inactivation on central carbon and energy metabolism, high sensitivity to oxidative damage, and repression of production of polyketide antibiotics. PirA has been shown to be a novel redox-sensitive negative modulator of very long-chain acyl-CoA dehydrogenase that catalyzes the first committed step of the beta-oxidation pathway. Most of these effects could therefore be traced to the inability of the pirA-defective strain to modulate beta-oxidation pathway, leading to an unbalanced supply of precursors for polyketide biosynthesis (Tala et al. 2018). These results suggest that although insertion into the PhiC31 attB site may in many cases be almost neutral, sufficient for some applications, insertional inactivation of pirA homologs may have substantial metabolic defects that may not be adequate for the construction of producing strains.
ΦBT1 Att/Int system
A suitable alternative to the ΦC31-Att/Int system are vectors derived from ΦBT1 phage that is related to ΦC31 (Gregory et al. 2003). Despite extensive similarities between the genomes of both phages, their attP/int loci are poorly conserved, although ΦBT1 integrase also belongs to the serine recombinase family. ΦBT1 integrates into a different attachment site than ΦC31 (Table 1). The ΦBT1 attB site is located within the SCO4848 gene encoding a conserved integral membrane protein in the genome of S. coelicolor or its ortholog in other streptomycetes (Gregory et al. 2003; Zhang et al. 2008). Three integration plasmids, pRT801, pRT802, and pMS82, were constructed with different resistance marker genes (for apramycin, kanamycin, and hygromycin, respectively), all containing oriT for E. coli–Streptomyces conjugation. These ΦBT1 Att/Int integrative vectors have been shown to have a wide host range and are fully compatible with those based on the ΦC31 Att/Int system (Gregory et al. 2003).
The ΦBT1 Att/Int system was also used to construct the conjugative BAC vector pSBAC which was used to clone the 90-kb meridamycin gene cluster and its ermEp*-driven heterologous expression in S. lividans (Liu et al. 2009). This BAC vector was also successfully used for cloning and efficient heterologous expression of two other large gene clusters for tautomycetin and pikromycin (Tocchetti et al. 2018). In addition, it has been modified for cloning and heterologous expression of whole gene cluster for calcium-dependent lipopetide antibiotic A54145 (Alexander et al. 2010).
Although ΦBT1 Att/Int-derived vectors have good stability and broad utility in Streptomyces (Baltz 2012), there are minor drawbacks using this system. The integration process between ΦBT1 attP and attB is very efficient, but was not completely unidirectional as the one for ΦC31. It can also partially act as excisionase. A low but significant frequency of excision recombination between attL and attR was observed in the presence of the ΦBT1 integrase alone (Zhang et al. 2008). Initially, the insertion of ΦBT1 Att/Int integrative vectors was thought to be neutral for Streptomyces strains (Gregory et al. 2003). However, later work showed that disruption of SCO4848 in S. coelicolor caused a delay in spore germination. This disruption affected the expression of SCO4849, which is cotranscribed with SCO4848 and also encoded a putative transmembrane protein. The detected phenotype was complemented with SCO4849. To overcome the problem, four plasmids were generated, pNG1, pNG2, pNG3, and pNG4, which modified the ΦBT1 pMS82 integrative vector to restore SCO4849 expression. They all produced a neutral phenotype when integrated into Streptomyces (Gonzalez-Quiñonez et al. 2016).
VWB Att/Int system
Another alternative to ΦC31 integration system are the vectors based on phage VWB identified in S. venezuelae. In contrast to both previous Att/Int systems, the VWB integrase belongs to the tyrosine recombinase family and the VWB attB site is located in the putative tRNAArg (AGG) gene conserved in Streptomyces species (Table 1). Phage integration restores the gene at the attL site, but lacks the CCA terminus, typical of prokaryotic tRNAs. However, most Streptomyces tRNA terminals also do not have this terminal CCA. Using the VWB Att/Int system, the integrative plasmid pKT02 was constructed but lacked the oriT origin of conjugation transfer. It has been successfully introduced into strains of S. lividans and S. venezuelae by protoplast transformation, but with less efficacy than the ΦC31-derived integration vectors (Van Mellaert et al. 1998). To improve the properties of this vector, its variant pSOK804 containing oriT was constructed. It is integrated into single site in S. noursei with an efficiency of approximately 2 orders of magnitude greater than pSET152 (Sekurova et al. 2004).
The VWB Att/Int system was used to construct the conjugative TAR vectors pCLY10 and pCLY11 based on p15a and F-factor replicons, respectively, for cloning large gene clusters of secondary metabolites. The vectors were successfully used to assemble a grecocycline biosynthetic gene cluster from Streptomyces sp. Acta 1362 and its heterologous expression in S. albus J1074 (Bilyk et al. 2016). Recently, we used the pCLY10 vector for cloning and efficient heterologous expression of the biosynthetic gene cluster for antitumor polyketide mithramycin A in engineered S. lividans RedStrep strains. The yield of mithramycin A was up to 3 g/L of medium, which is sixfold higher than the amount of the overproducing mutant strain for production of mitramycin A, S. argillaceus (Novakova et al. 2018).
TG1 Att/Int system
Another Att/Int system is based on the bacteriophage TG1 isolated from S. cattleya. Its integrase belongs to the serine recombinase family and the TG1 attB site is located within the SCO3658 gene encoding conserved aminotransferase in the S. coelicolor genome or its ortholog in other streptomycetes (Table 1). Using the TG1 Att/Int system, the integration plasmid pKU462 was constructed, which also contains oriT for E. coli–Streptomyces conjugation and was successfully integrated into S. avermitilis (Morita et al. 2009). SCO3658 orthologs and TG1 attB sites have been found in various Streptomyces strains (Baltz 2012; Morita et al. 2009). Compatibility with other phage-based integration systems has been demonstrated by constructing another TG1 Att/Int conjugative integration plasmid pRF10 that was efficiently introduced to several Streptomyces strains. Individual parts of the erythromycin biosynthetic gene cluster were cloned into four compatible integration plasmids, including pFF10. The other three Att/Int integration systems were derived from ΦC31, ΦBT1, and SV1. All four integration plasmids with individual parts of the erythromycin biosynthetic gene cluster were integrated into two heterologous host strains, S. coelicolor and S. lividans, and robust production of various erythromycin intermediates was achieved. This multiplex integrative approach has provided a useful platform for polyketide synthase enzyme engineering for combinatorial biosynthesis of novel compounds (Fayed et al. 2015).
R4 Att/Int system
This system is based on a broad-host-range R4 bacteriophage isolated from soil on S. albus J1074 (Chater and Carter 1979). Its unidirectional integrase belongs to the serine recombinase family and its attB site is located within the SCO6196 gene encoding the acyl-CoA synthetase homolog in S. coelicolor or its ortholog in other Streptomyces species (Table 1) (Baltz 2012; Matsuura et al. 1996; Miura et al. 2011). Despite its well-characterized nature, the R4 Att/Int system has not been widely used in Streptomyces integration systems, although it has been successfully used to integrate into E. coli, cyanobacteria, and human cells (Miura et al. 2011, 2014; Olivares et al. 2001). However, the R4 Att/Int system has recently been used to construct the conjugative integration vector pLR4 and efficiently integrated into three Streptomyces strains. This vector was a part of five compatible integration systems (derived from ΦC31, ΦBT1, R4, SV1, and TG1) for the multiplex site-specific genome engineering system for single-step, multi-locus integration of biosynthetic gene clusters for bioactive secondary metabolites into streptomycetes (Li et al. 2019).
SV1 Att/Int system
This system is based on bacteriophage SV1 isolated from S. venezuelae. Its unidirectional integrase belongs to the serine recombinase family and its attB site is located within the SCO4383 gene encoding a 4-coumarate:CoA ligase homolog in S. coelicolor or its ortholog in other Streptomyces species (Table 1). The SV1 Att/Int system was used to construct a conjugal pBF3 vector that integrates efficiently into several Streptomyces species; however, it does not integrate into its native host, S. venezuelae (Fayed et al. 2014). As described above, the SV1 Att/Int-derived pBF3 plasmid was successfully used together with other three compatible Att/Int integration systems (derived from ΦC31, ΦBT1, and TG1) for cloning and heterologous expression of individual parts of the erythromycin gene cluster (Fayed et al. 2015). Similarly, the SV1 Att/Int system was also used to construct the conjugative integration vector pLSV1 as part of the five compatible integration systems (ΦC31, ΦBT1, R4, SV1, TG1 Att/Int-derived) for the multiplex site-specific genome engineering system (Li et al. 2019).
ΦJoe Att/Int system
This system is based on recently described bacteriophage ΦJoe isolated from soil on S. coelicolor (Fogg et al. 2017). Its integrase belongs to the serine recombinase family. The ΦJoe Att/Int system was used to construct the conjugal vector pCMF92, which integrated with very low potency into S. coelicolor and S. lividans, but with dramatically higher potency in S. venezuelae (160-fold greater than S. coelicolor and 1600-fold greater than S. lividans). This difference was solved after examining the attB integration site for the ΦJoe Att/Int system. Two integration sites were identified in S. coelicolor, separated by a mobile genetic element containing SCO2603, encoding putative serine integrase with 68% identity to ΦJoe integrase. Plasmid integration was ineffective because the ancestral optimal attB site is occupied by the SCO2603 encoding element. These two integration sites in S. coelicolor were called attLsc and attRsc to reflect the origin of the sites containing the mobile element. However, a single attB site (Table 1), very similar to the reconstituted attB site from two halves attLsc and attRsc, was identified in S. venezuelae. Bioinformatic analysis showed that the attB site for ΦJoe integrase is located at the end of the conserved SCO2606 gene in S. coelicolor (or SVEN_2383 in S. venezuelae) encoding the predicted B12 binding domain-containing radical S-adenosylmethionine protein. In several Streptomyces species, this site was occupied, similarly to S. coelicolor, by a mobile element that differs in gene content and size. Unlike previous serine phage integrases, ΦJoe integrase showed significant excision in the absence of its RDF. This bidirectional property of this system could be applied in several new ways in genome engineering (Fogg et al. 2017).
μ1/6 Att/Int system
The last phage-derived Att/Int system under investigation is based on the bacteriophage μ1/6 isolated from S. aureofaciens. Its integrase belongs to family of tyrosine recombinases. The μ1/6 attB site is located at the 3′-end of the tRNAThr (ACA) gene conserved in Streptomyces species, but the tRNA gene was completely restored after phage-integration (Table 1). The μ1/6 Att/Int system was used to construct the pCTint integration vector, but lacked the oriT origin of conjugal transfer. The plasmid was effectively integrated into three Streptomyces strains (Farkasovska and Godany 2012).
pSAM2 Att/Int system
The last characterized Att/Int system is based on the 11-kb natural integration plasmid pSAM2 identified in S. ambofaciens and able to site-specifically integrate into the genome of various Streptomyces species (Boccard et al. 1989; Kuhstoss et al. 1989). Its integrase belongs to the tyrosine recombinase family and is encoded by the int gene translationally coupled with the upstream xis gene encoding excisionase. Both constitute an operon (Sezonov et al. 1998). The pSAM2 attB site is located at the 3′-end of the tRNAPro (CCG) gene conserved in Streptomyces species and the tRNA gene was restored upon integration. Both the attP and attB regions share an identical 58-bp segment, but the minimum attB site length is 26 bp (Table 1). Interestingly, a relatively large 360-bp attP site is required for integration (Raynal et al. 1998, 2002). The pSAM2 Att/Int system was used to construct two vectors, the integration plasmid pKC824 and the cosmid pKC767, both of which lacked oriT for conjugation. Both vectors were able to integrate into various Streptomyces strains, but their integration efficiency was significantly lower (60-fold and 600-fold) than their ΦC31 Att/Int-derived counterparts (Kuhstoss et al. 1991). Another pPM927 conjugative vector, based on the pSAM2 Att/Int system, was efficiently and stably integrated into one site in S. lividans (Smokvina et al. 1990). Its successful integration has been reported in several other Streptomyces species (Myronovskyi and Luzhetskyy 2013), indicating its broad host specificity and use for stable site-specific integration in Streptomyces. For example, the pSAM2 Att/Int-derived vector was successfully used to introduce the gene cluster fkbG-K for the methoxymalonyl-ACP biosynthetic pathways into S. fradiae to dramatically increase (more than 1 g/l) heterologous production of macrolide polyketide midecamycin (encoded by the mdm gene cluster), compared with S. fradiae with the mdm gene cluster integrated using the ΦC31-based Att/Int system (Rodriguez et al. 2004).
As described above, these Att/Int systems have found widespread use in gene manipulations in streptomycetes and, in particular, in the stable integration of biosynthetic gene clusters for many biologically active secondary metabolites into heterologous hosts. Many vectors based on these integration systems have been prepared and successfully used for efficient heterologous expression of various bacterial biosynthetic pathways producing new biologically active natural products or known products with increased yield compared with the original hosts. More than 100 different biologically active agents, including 21 completely new compounds, prepared by this approach are summarized in a recent review article (Huo et al. (2019). The great advantage of these Att/Int systems, particularly in connection with the conjugative oriT origin, is high efficiency of their integration into the chromosome of Streptomyces strains (Baltz 2012). Another useful feature of these integration systems is that each has a unique attB site located at a different position in the particular Streptomyces genome. These Att/Int systems with their attB sites in the S. coelicolor genome are shown in Table 1. Another great advantage of the Att/Int integration vectors is that they can be multiplexed without interference during recombination or conjugation and without loss of stability. Thus, more copies of a gene cluster or more genetic elements can be stably inserted into individual attB sites (Fayed et al. 2015; Gregory et al. 2003; Li et al. 2019).
Despite all the benefits of these Att/Int systems, they also have some limitations. As mentioned above, some results suggested that insertion into the attB site may result in a substantial reduction of secondary metabolite production (Baltz 1998; Tala et al. 2018). Although Att/Int-derived vectors have good stability and broad applicability in Streptomyces, not all Streptomyces strains contain attB integration sites for these vectors (Baltz 2012; Myronovskyi and Luzhetskyy 2013). For example, our model strain S. lavendulae subsp. lavendulae CCM 3239 (Busche et al. 2018) can only efficiently integrate ΦC31 and pSAM2 Att/Int-derived integrative plasmids (Novakova et al. 2011; J. Kormanec, unpublished results). However, an artificial attB site could be inserted into such strains to allow efficient integration, as demonstrated in several cases (Li et al. 2017a, 2019; Manderscheid et al. 2016). The other disadvantage of these systems is that they integrate the entire integration plasmid with the E. coli replicon, the integrase gene and the resistance marker gene (Fig. 1). It is a significant biotechnological obstacle, as biotechnological production strains should avoid the antibiotic resistance genes. Similarly, the expression of the integrase gene and the E. coli plasmid replication gene can affect the stability of the constructs. In fact, the instability of several Att/Int systems has been reported in several Streptomyces strains (Baltz 2012; Fayed et al. 2014; Myronovskyi and Luzhetskyy 2013; Siegl et al. 2010; Siegl and Luzhetskyy 2012). We also previously found that an E. coli origin of replication caused dramatic instability after integration in some Streptomyces chromosomes (Kormanec et al. 1993). Although the utility of these Att/Int systems is evident for many gene manipulations and applications in streptomycetes, this integration method may not be suitable for the construction of stable biotechnological production strains. These problems can be overcome by another method of stable integration into the Streptomyces chromosomes by homologous recombination, as will be described in the following section of this article.
Genome editing toolkit based on homologous recombination
Homologous recombination (HR) is a highly conserved process common to both prokaryotic and eukaryotic cells, which is essential to maintain genome stability and plays an important role in genome evolution. It is also an effective mechanism of DNA repair necessary for cell survival when DNA suffers from various types of damage, including double-strand breaks. The HR process requires energy and assistance from conserved mediator and recombinase proteins and involves aligning similar DNA sequences to form mobile forks between four DNA strands called Holliday junctions, and ultimately complete exchange of two complementary strands (Costes and Lambert 2013; Wright et al. 2018).
HR-based methodology is a powerful tool for stable genome engineering, including deletion, replacement, and insertion of genomic sequences. HR gene modifications in Streptomyces are usually based on suicidal non-replicating vectors that carry DNA fragments surrounding the target gene(s) to be deleted with the antibiotic resistance marker gene. Such a mutant allele on the vector is used to replace the chromosomal copy of the target gene(s) via two crossovers, on both sides of the antibiotic resistance marker gene, resulting in a stable mutation. These two crossovers may occur simultaneously, resulting in the replacement of the target gene(s) with the antibiotic resistance marker gene, or may occur sequentially via a single crossover intermediate in which the entire vector is integrated into the chromosome through one of the homologous regions. The vectors therefore also carry a counterselectable marker (a second different antibiotic resistance marker gene). The final replacement of the desired gene(s) in the Streptomyces chromosome is accomplished by a double crossover event, usually after two rounds of sporulation, by selecting only for the antibiotic resistance marker inserted into the target gene(s) prior to spore plating for the individual colonies. The double crossover is subsequently identified by replica plating to select a loss of the counterselectable marker (second antibiotic resistance marker gene). However, these techniques are time consuming and require screening of thousands of clones by replica plating (Kieser et al. 2000) (Fig. 2).
Scheme of deletion of a target gene (green arrow) with a conventional inactivation system using a suicidal non-replicating plasmid. UP, upstream homology arm; DOWN, downstream homology arm; aac(3)IV, the apramycin resistance marker gene used to replace the target gene as an example (red arrow); neo, the Tn5 counterselectable kanamycin resistance marker gene as an example (dark blue arrow)
Unlike the site-specific Att/Int-based integrations, HR is much less effective and depends on the size of crossover homologous regions. Generally, the lower limit is about 500 bp, but for some strains, much larger (several kb) homologous DNA fragments are needed (Kieser et al. 2000). To increase the efficacy of HR, a PCR-targeted system (REDIRECT) has been developed for efficient gene deletions in Streptomyces. The strategy used μ-Red-mediated recombination in E. coli (Datsenko and Wanner 2000) between PCR-amplified antibiotic resistance cassette flanked by 39-nt homologous arms and the large Streptomyces DNA (about 40 kb) on cosmid where the target gene(s) are replaced with this cassette. The amplified cassette contains the origin of transfer oriT for E. coli–Streptomyces conjugation and is flanked by yeast Flp recombinase target sequences FRT for the removal of the antibiotic resistance marker gene. Such a mutated cosmid is subsequently used to be introduced into Streptomyces to replace the target gene(s) with the cassette. Due to the large homologous DNA fragments flanking the cassette, the recombination process between the cosmid and Streptomyces chromosome is more effective (Gust et al. 2003). In addition to gene deletions, this REDIRECT system has also been successfully used for insertions into the Streptomyces genome, such as the insertion of a strong ermEp* promoter upstream a “silent” gene cluster for a secondary metabolite allowing its activation (Novakova et al. 2011; Zheng et al. 2007). However, this system requires an ordered cosmid library that may be a hindrance to Streptomyces strains that have not been genetically well characterized, and also does not overwhelm the screening of large number colonies by replica plating to distinguish between single and double crossover.
In order to avoid these laborious and time-consuming steps, several gene inactivation systems with positive selection of double crossovers have been developed. One of these is a system based on the glkA counterselectable gene encoding glucose kinase which confers a sensitivity to the glucose analog 2-deoxyglucose. Thus, after selection on plates containing this toxic compound, only double crossovers lacking glkA can grow. However, this system requires a Streptomyces strain that carries a glkA deletion to allow counterselection of glkA in the vector. If the glkA mutation interferes with further analysis, the final gene disruption can always be crossed into a glkA+ genetic background (van Wezel and Bibb 1996). The second counterselectable gene used for positive selection of double crossovers is rpsL, which encodes S12 ribosomal protein. It confers streptomycin sensitivity in selected spontaneous streptomycin-resistant Streptomyces strain. The rpsL gene was cloned into a plasmid vector containing a Streptomyces pSG5-based temperature-sensitive replication origin and a temperature shift from 29 to 39 °C was used to select double crossovers. However, this system also requires a mutated Streptomyces strain that carries a rpsL mutation to allow counterselection of rpsL+ on the vector (Hosted and Baltz 1997). Another counterselectable system utilizes the synthetic codA gene encoding cytosine deaminase. CodA enzyme converts 5-fluorocytosine into a highly toxic 5-fluorouracyl. After selection in 5-fluorocytosine-containing plates, only double crossovers lacking codA can grow (Dubeau et al. 2009). The use of a suitable chromogenic selectable marker gene to distinguish between single crossover and double crossover mutants by producing colonies of different colors could simplify the procedure and significantly reduce selection time. The first such gene was gusA encoding β-glucuronidase that is absent in Streptomyces strains. The GusA enzyme hydrolyzes a wide variety of β-glucuronides, including commercially available chromogenic substrate 5-bromo-4-chloro-3-indolyl-β-D-glucuronide, which is converted to blue products. This system enables to distinguish between blue single crossover and white double crossover clones. It has been successfully used for deletion of several secondary metabolite gene clusters in S. albus. However, the disadvantage of this system is the relatively high cost of this substrate (Myronovskyi et al. 2014). Thus, all the above counterselectable systems require either mutated Streptomyces host strains or external supply of substrates. Therefore, we have developed a novel efficient gene deletion system based on the positive selection of double crossovers using a counterselectable bpsA gene from S. aureofaciens CCM 3239, which encodes a protein for the synthesis of blue pigment indigoidine. The suicidal non-replicative deletion plasmid pAMR4 contains the apramycin resistance marker gene with oriT for easy E. coli–Streptomyces conjugation. The resistance marker gene was flanked by yeast Flp recombinase target sequences FRT for removal of the antibiotic resistance marker gene. In addition, this apramycin resistance marker cassette was flanked by two polylinkers with several unique sites for rare restriction enzymes in streptomycetes to clone two homologous regions for crossovers. The plasmid contains an additional counterselectable kanamycin resistance gene which also directs the expression of the bpsA gene (Knirschova et al. 2015). After conjugation of the recombinant plasmid containing the two PCR-amplified DNA fragments with the homologous regions flanking the gene(s) to be deleted into the Streptomyces strain, blue colonies (presence of blue-pigmented indigoidine produced by BpsA) and the uncoloured colonies (absence of BpsA) allow easy detection of single and double crossover recombination, respectively (Fig. 3). The system was successfully tested by the deletion of the 21.2 kb act gene cluster responsible for the biosynthesis of polyketide antibiotic actinorhodin in S. lividans TK24 (Knirschova et al. 2015). A similar blue-white deletion system was independently developed using the homologous indigoidine biosynthetic idgS gene from S. lavendulae CGMCC 4.1386 (Li et al. 2015).
Scheme of deletion of a target gene (green arrow) with the gene deletion system based on the positive selection of double crossovers using the counterselectable bpsA gene that encodes a protein for the synthesis of blue pigment indigoidine (Knirschova et al. 2015). The suicidal non-replicating plasmid pAMR4 contains two homologous arms surrounding the gene (UP and DOWN) to be deleted, the promoterless indigoidine biosynthetic gene (bpsA) and the Tn5 kanamycin resistance marker gene (neo) directing bpsA expression. The yellow box indicates the cassette which contains the aac3(IV) apramycin resistance marker gene, the oriT origin of conjugal transfer and is flanked by yeast Flp recombinase target sequences FRT (Gust et al. 2003). To remove the antibiotic resistance marker gene, a plasmid pUWLFLP containing the Flp recombinase gene (Fedoryshyn et al. 2008a) was introduced into the double crossover mutant. A solid Bennet medium plate with colonies after two rounds of non-selective sporulation is shown to distinguish between double-crossover AprR clones (white colonies) and single-crossover AprS clones (blue colonies)
Marker-free genome editing tools
However, all these gene deletion strategies lead to the replacement of the target gene(s) with a selectable antibiotic resistance marker gene that may have a polar effect on the expression of downstream genes. In addition, multiple gene deletions in the same Streptomyces strain are restricted to a limited number of antibiotic resistance marker genes. Therefore, several strategies were introduced to remove this resistance marker gene. In the REDIRECT PCR-targeting method, the Flp/FRT recombination system is used as an assistant tool to remove resistance markers. First, a temperature-sensitive replication plasmid pCP20 containing the thermoinducible Flp recombinase gene is introduced into E. coli, which contains the cosmid with the replaced target gene(s) by the antibiotic resistance marker gene cassette flanked by FRT sites. Expression of the FLP recombinase removes the central part of the FRT-flanked disruption cassette from the disrupted gene leaving an 81-bp “scar” sequence that contains a single FRT site. Subsequently, the resulting cosmid containing the markerless deletion is introduced into the S. coelicolor mutant carrying the marked deletion in the target gene. Replacement of the marked deletion in the Streptomyces chromosome with the markerless deletion in the cosmid is screened by replica plating for the loss of antibiotic resistance marker gene and the counterselectable antibiotic resistance marker gene (kanamycin resistance in the case of SuperCos 1 cosmid) (Gust et al. 2003). The drawback of this REDIRECT system is that Flp/FRT recombination must be performed in E. coli and the selection procedure in Streptomyces with unmarked cosmid, which is a very laborious and time-consuming process. To overcome these limitations, two Streptomyces replicative plasmids, pUWLFLP and pALFLP, were constructed with pIJ101-based and the temperature-sensitive pGS5-based replicons, respectively. Both contain a synthetic gene encoding Flp recombinase with GC content optimized for expression in Streptomyces under the control of a strong ermEp* promoter and the origin of transfer oriT. After conjugation of these replicative plasmids, the apramycin resistance marker gene flanked by FRT sites was removed from Streptomyces chromosome with 40% efficiency (Fedoryshyn et al. 2008a) (Fig. 3). The other two site-specific recombination systems, Cre/loxP from the phage P1 and Dre/rox from the phage D6, were also successfully used to remove the resistance marker gene in a similar manner using pIJ101-based or pSG5-based Streptomyces replicative plasmids pUWLCRE/pUWLDRE and pNLCRE/pALDRE, respectively. Similarly, they contain synthetic genes encoding Cre or Dre recombinases with codon usage optimized for expression in Streptomyces. After conjugation of these plasmids, the apramycin resistance marker gene flanked by loxP or rox sites was removed from Streptomyces chromosome with 100% efficiency. Because all three site-specific recombination systems are strictly specific to their target sites, they could be used sequentially in multiple mutations in a single Streptomyces strain (Fedoryshyn et al. 2008b; Hermann et al. 2012).
All three site-specific recombination systems described above have one limitation, they cannot be used iteratively because they leave an active recognition site in the Streptomyces genome. However, the stable manipulation of Streptomyces genomes requires the manipulation of dozens of loci that can improve their biotechnological properties, e.g., in the preparation of minimal Streptomyces genomes for efficient chassis for the discovery and overproduction of natural product or for the integration of multiple genes (Liu et al. 2018). Thus, there is a need for a site-specific recombination system that can be iteratively used for such multiple genome editing purposes. Such a system, called iterative marker-free excision system (IMES), has been developed. It uses ΦC31 integrase and its mutated att sites B-CC and P-GG. These mutated sites are derivatives of the ΦC31 attB and attP sites by substituting the central core TT dinucleotide with CC and GG. IMES can be used to effectively remove antibiotic resistance marker gene flanked by inversely oriented B-CC and P-GG. The resulting RR site, which evolves as a result of the deletion, is the joining product of the right arms of B-CC and P-GG and is unable to recombine with P-GG and B-CC. Several cassettes carrying synthetic genes of the apramycin, spectinomycin, or hygromycin resistance markers, flanked by the P-GG and B-CC sites, have been generated for this strategy. They were efficiently excised from the Streptomyces chromosome containing the replaced target genes by expression of the ΦC31 integrase from the pIJ101-based Streptomyces replicative pUWLint31 plasmid. Thus, IMES can be used for multiple genomic engineering without the risk of unwanted DNA recombination (Myronovskyi et al. 2014). IMES has recently been used for the successful deletion of all 15 secondary metabolite gene clusters from S. albus J1074 chromosome, resulting in a cluster-free S. albus Del14 chassis strain that was subsequently used to improve heterologous expression of several secondary metabolite gene clusters (Myronovskyi et al. 2018).
Using this INES strategy, we have modified our previous effective deletion system based on the positive double crossovers selection with the bpsA gene responsible for the blue pigment indigoidine production. To reduce unwanted recombination in successive multi-step genomic deletions, new non-replicative plasmid vectors, pAMR12 and pSPR12, were prepared by replacing the apramycin resistance marker gene in pAMR4 with synthetic apramycin and spectinomycin resistance genes, respectively. Both cassettes were flanked by recombination sites P-GG and G-CC for ΦC31 recombinase and contain the conjugation origin oriT of transfer. Similarly, the two new cassettes were flanked by two polylinkers with several unique sites for rare restriction enzymes in streptomycetes to clone two homologous regions for crossovers. Using this new modified system, we prepared a collection of S. lividans RedStrep strains with sequentially deleted gene clusters for five interfering secondary metabolites: act for actinorhodin, red for undecylprodigiosin, cdc for calcium-dependent antibiotic, cpk for coelimycin, mel for brown pigment melanin. In addition, the hrdD gene for the stationary phase sigma factor and the scbAR genes for the γ-butyrolactone system were deleted. In these strains, we demonstrated a dramatic increase in the heterologous production of the antitumoral polyketide mitramycin A, encoded by the mtm gene cluster (up to 3 g/L medium), compared with S. lividans TK24 with the mtm gene cluster integrated using the VWB-based Att/Int system. It demonstrated the biotechnological impact of S. lividans RedStrep strains on heterologous production of biologically active substances (Novakova et al. 2018).
All the markerless systems described above have one disadvantage. In order to remove the antibiotic resistance marker gene, the site-specific recombinase gene must be delivered to the Streptomyces strain in the replicative plasmid (pIJ101-based or temperature sensitive pGM5-based). In addition to this additional time-consuming step, it is also possible that some Streptomyces strains may not accept these replicative plasmids. Although pIJ101 derivatives have a wide host range within the genus Streptomyces, only 13 out of the 18 strains have been successfully transformed (Kieser et al. 1982). Similarly, we were unable to introduce these pIJ101 derivative plasmids into our model S. lavendulae subsp. lavendulae CCM 3239 (Busche et al. 2018; Kormanec et al. 1993). In addition, the scar sequence left after removal of the resistance marker gene contains an integration site (e.g., an 81-bp FRT site for Flp recombinase) with a longer inverted repeat that can affect adjacent genes. To simplify the markerless deletions and to reduce the time needed, we prepared a new efficient markerless deletion system based on the positive selection for double crossovers with the blue pigment indigoidine bpsA gene. A new suicidal non-replicative plasmid vector pAMR18 containing a large polylinker with many unique restriction sites flanked by the apramycin and kanamycin resistance genes (used for single crossover selection) and the bpsA gene for double crossover selection were created. To delete the target gene(s), two PCR-amplified DNA fragments from its flanking regions can be cloned into the large polylinker of pAMR18A, leaving just one restriction site between them. Since pAMR18A does not have the replication origin of Streptomyces, it will be integrated into the Streptomyces genome by HR of one of the cloned Streptomyces DNA fragments. After two rounds of non-selective sporulation, it is possible to readily select double crossover mutant or wild-type reverse colonies based on colony color (blue or white) and then examined by PCR to distinguish between these two events (Fig. 4). This system was successfully tested by the deletion of the 21.2-kb act gene cluster for polyketide antibiotic actinorhodin in S. lividans TK24 with 30% efficacy (3 out of 10 selected white colonies contained correctly deleted act) or by effective deletion of the matAB operon for mycelial aggregation in selected S. lividans RedStrep strains with 40% efficiency. In addition, the system was used for the markerless insertion of the mCherry gene under the control of the subtilisin inhibitor protein secretory signal sequence into the chromosome of S. lividans TK24 (with 30% efficacy), resulting in efficient secretion of mCherry into the culture medium. Thus, this markerless system can also be used to stably insert foreign genes or gene clusters into any neutral position of the Streptomyces chromosome (Rezuchova et al. 2018).
Scheme of deletion of a target gene (green arrow) using the markerless gene deletion system with the bpsA indigoidine biosynthetic gene (Rezuchova et al. 2018). The suicidal non-replicating plasmid pAMR18A contains two homologous arms flanking the deleted gene (UP and DOWN), the promoterless indigoidine biosynthetic gene (bpsA) and the Tn5 kanamycin resistance marker gene (neo) driving bpsA expression. The yellow box indicates the cassette which contains the aac3(IV) apramycin resistance marker gene, the oriT origin of conjugal transfer and is flanked by yeast Flp recombinase target sequences FRT (Gust et al. 2003). A solid Bennet medium plate with colonies after two rounds of non-selective sporulation is shown to distinguish between double-crossover clones or wild-type revertant clones (white colonies) and single-crossover clones (blue colonies)
Compared with the site-specific integrative Att/Int systems, HR-based genome editing systems have several advantages. They are more stable because they leave only the deleted or inserted gene(s) with a short scar sequence (50 to 80 bp) or a single restriction site (6 bp) as opposed to the Att/Int systems that integrate the entire integrative plasmid together with the E. coli replicon, integrase gene, and the resistance marker gene. In addition, they can stably integrate foreign DNA into any neutral chromosome position. However, the main disadvantage of HR-based genome editing systems is their low integration efficiency, depending on the size of the homology arms. In addition, it differs from Streptomyces strain to strain. Two systems have been developed to increase HR efficiency.
I-SceI endonuclease-based genome editing system
The first is based on the yeast mitochondrial homing endonuclease I-SceI, which specifically recognizes and cleaves the 18-bp DNA sequence TAGGGATAACAGGGTAAT. The efficacy of HR could be improved by introducing double-strand DNA breaks (DSBs) into the desired DNA region. In the presence of a homology template, these breaks can be effectively repaired by HR. This strategy is used in this I-SceI-based system to perform markerless gene mutations. Theoretically, the I-SceI recognition sequence appears once in 70 billion bases of a random sequence, and Streptomyces genomes analyzed to date are free of I-SceI recognition sites. To perform a markerless mutation, the mutant gene is cloned into a suicidal non-replicative plasmid that contains the antibiotic resistance marker gene and the I-SceI recognition site. The plasmid is introduced into the Streptomyces strain and integrated into the chromosome by HR. Subsequently, the I-SceI endonuclease gene is expressed in the resulting mutant upon conjugation with one of the Streptomyces replicative plasmids, pALSCE or pUWLHSCE, containing pIJ101 or the temperature sensitive pGS5 replicons, respectively. Both contain a synthetic gene encoding I-SceI recombinase using codons optimized for Streptomyces under the control of a strong tipAp or ermEp* promoter, respectively. The DSB introduced by I-SceI stimulates intramolecular recombination between wild-type and mutant gene. Chromosome repair and viability can only lead to HR between duplicated regions of the genome, providing selection for a second crossover event that provides a mixture of both the original and the mutant gene. They can be easily identified by PCR analysis. Finally, the replicative plasmids containing the I-SceI endonuclease gene are removed from the mutant strain after several rounds of non-selective sporulation (pUWLHSCE) or increased temperature (pALSCE). This procedure significantly increased efficacy of HR leading to markerless mutation and could be used for various manipulations of genome. In addition, the marker-free nature of the mutations facilitates the generation of multiple gene deletions in one genomic background (Siegl et al. 2010). A similar I-SceI-based system was used to efficiently delete the red gene cluster for the antibiotic undecylprodigiosin in S. coelicolor (Fernandez-Martinez and Bibb 2014). However, as in the previous cases described above, the use of Streptomyces replicative plasmids to introduce I-SceI expression into Streptomyces is a disadvantage of the I-SceI endonuclease recombination system, since some Streptomyces strains may not accept these replicative plasmids.
CRISPR/Cas-based genome editing systems
Another system to increase HR efficacy in the markerless genome manipulations in Streptomyces is a CRISPR/Cas-based genome editing tool that has revolutionized genomic engineering in almost every organism over the past six years, including Streptomyces species (Tao et al. 2018; Tong et al. 2018). Clustered Regularly Interspaced Short Palindromic Repeats (CRISPR)/Cas (CRISPR-associated proteins) is an adaptive immune system to defend against exogenous DNA or RNA. Three different CRISPR/Cas systems have been identified. Type I and type III require multiple Cas proteins to induce cleavage of their target DNA. However, type II systems require a single Cas9 endonuclease. The best studied example of type II systems is Streptococcus pyogenes CRISPR/Cas9, which has allowed its widespread use in genome editing applications. This system consists of three elements: Cas9 endonuclease, short crRNA that confers target site specificity, and short tracrRNA facilitating crRNA processing and recruitment. The crRNA–tracrRNA duplex forms a complex with Cas9 and scans the target genome for the presence of PAM (Protospacer adjacent motif 5′-NGG-3′), in which 5′ sequence is complementary to the spacer sequence in the crRNA-tracrRNA duplex. Once the complex binds this specific target sequence, Cas9 specifically cleaves DNA resulting in a lethal DSB. These two small RNAs can be artificially fused into a single synthetic guide RNA (sgRNA) that retains full functionality (Fig. 5). The DSB must be repaired by rejoining the DNA ends. In the absence of a homologous template DNA, this can be achieved by activation of a non-homologous end joining (NHEJ) pathway. In the presence of a homologous template DNA, the ends can be efficiently joined by HR. In eukaryotes, NHEJ is the dominant pathway for DSB repair. However, NHEJ does not widely occur in bacteria, including Streptomyces. Therefore, the repair of bacterial DSB relies mainly on HR (Selle and Barrangou 2015; Tao et al. 2018; Tong et al. 2018).
Scheme of the class II Streptococcus pyogenes CRISPR/Cas9 system. The crRNA (blue) and tracrRNA (green) are fused by a short loop (orange) to create a sgRNA. The 20 nucleotides programmable sequence in the sgRNA to recognize the target sequence (dark blue) in genome is underlined. PAM sequence is in red font. The CRISPR-associated protein 9 (Cas9 nuclease) is indicated by yellow oval. The positions of the cleavage of the target DNA strands by Cas9 nuclease are indicated by red arrows
To date, four S. pyogenes-based CRISPR/Cas9 systems have been developed and applied to rationally edit the Streptomyces genome, including the following delivery plasmid vectors: pCRISPomyces-2 (Cobb et al. 2015); pCRISPR-Cas9 (Tong et al. 2015); pKCcas9dO (Huang et al. 2015); and pWHU2059 (Zeng et al. 2015). All these four systems share some common designs and applications. They use a one-vector strategy that combines the synthetic S. pyogenes cas9 gene with optimum codon usage for Streptomyces and the sgRNA expression cassette, both under the control of the promoters active in these bacteria, and the homology repair template for HR on a single delivery plasmid. Systems specifically generates DSB at a target site using Cas9 under the guidance of a sgRNA harboring a custom-designed spacer, and then repairs it by HR repair in the presence of a homology repairing template, resulting in an accurate markerless deletion of the gene or large gene cluster with efficacy ranging from 50 to 100% in several Streptomyces strains. Using these systems, the multiplex genome editing has also been achieved by equipping multiplex sgRNA cassettes and corresponding homology repairing templates (Cobb et al. 2015; Huang et al. 2015). Besides, CRISPR/Cas9 was also used to introduce nucleotide point mutations into the rpsL gene to generate a specific streptomycin resistant mutation (Huang et al. 2015). The first three systems are based on the Streptomyces temperature-sensitive pSG5 replicon to facilitate multiple rounds of editing (Cobb et al. 2015; Tong et al. 2015; Huang et al. 2015). The latter (pWHU2059), is based on a segregation-unstable sti- pIJ101-derived E. coli–Streptomyces high-copy number shuttle vector producing large amounts of single-stranded plasmid DNA and hence the amount of template DNA. The result was a substantially higher HR efficiency and target mutant frequency. In addition, this CRISPR/Cas9 system utilizes the D314A cytosine deaminase mutant CodA(sm), which converts 5-fluorocytosine to highly toxic 5-fluorouracil, as a counterselection approach to select delivery plasmid loss that significantly accelerates the screening process (Zeng et al. 2015). These studies have facilitated rapid progress in the modifications of the Streptomyces genome. For example, the CRISPR/Cas strategy using the pCRISPomyces-2 vector was used to efficiently insert strong promoters into several unknown silent biosynthetic gene clusters to activate their production in five Streptomyces species, resulting in the production of unique metabolites (Zhang et al. 2017). As noted above, cloning of large clusters (> 100 kb) is still an obstacle to heterologous expression of antibiotic gene clusters. However, this CRISPR/Cas9 approach in combination with Gibson assembly and TAR cloning allowed cloning of such large gene clusters (Jiang et al. 2015; Wang et al. 2015; Lee et al. 2015). Therefore, the CRISP/Cas9-based methodology appears to be a very promising tool for discovering novel bioactive secondary metabolites in Streptomyces and other Actinobacteria. All other applications of these CRISPR/Cas systems in the modification of the Streptomyces genome are described in two recent reviews (Tao et al. 2018; Tong et al. 2018).
The results of these studies show that CRISPR-Cas9 is more efficient and faster than other methods for markerless genome editing in Streptomyces species. However, it has some bottlenecks. Similarly to previous methods, it also uses Streptomyces replicative plasmids to deliver the system to streptomycetes, which may again cause similar replication problems for these plasmids in some strains, especially those that are less studied. Another concern with this CRISPR/Cas9 system is the occurrence of non-specific off-target effects that introduce DSBs in undesired regions of the genome at sites that resemble the 20-nt guide sequence flanking the NGG sequence (PAM). In fact, non-specific loss of huge portions of the genome has been observed in some Streptomyces strains after engineering experiments with CRISPR/Cas9. Therefore, for all CRISPR/Cas9 applications involving DBS generation, it is recommended to check the integrity of genomes (Tong et al. 2018).
Conclusion
The bacteria of the genus Streptomyces are excellent sources for many bioactive compounds with antibacterial, antifungal, herbicidal, immunosuppresive, antihelminthic, and antitumor properties. In addition, some Streptomyces strains, such as S. lividans, are perfect hosts for the effective secretion of heterologous biotechnologically and clinically relevant proteins.
These bacteria are therefore of great importance in the field of biotechnology and medicine (Barka et al. 2016; Dyson 2011). It is now clear that, based on genome analysis, their potential to produce new biologically active metabolites is enormous. However, many of them are not produced under laboratory conditions. Thus, efficient and stable genome manipulations are necessary to induce their production (Baltz 2016; Chen et al. 2016). Comprehensive studies in the past have led to an extensive and versatile collection of molecular biology tools for gene manipulation of Streptomyces, mainly based on their replicative plasmids, which require sustained antibiotic selection to maintain them (Kieser et al. 2000). However, biotechnological applications of these bacteria require stable genome alterations/mutation.
Two major strategies in the Streptomyces species have been developed to accomplish such a stable genome modification; integration into the chromosome through Att/Int site-specific integration systems based on Streptomyces actinophages or the integrative plasmid pSAM2, and integration through HR using suicidal non-replicating vectors. Each strategy has its advantages and limitations. Although Att/Int site-specific integration strategy is much more effective than the HR-based strategy, its biotechnology main concern is the parallel integration of the entire delivery vector, including the replication origin and the antibiotic resistance marker gene. Another limitation is the integration into a single specific attB site on the Streptomyces chromosome, which may not be present in some Streptomyces strains (Baltz 2012; Myronovskyi and Luzhetskyy 2013). However, both disadvantages can be overcome by using other genome editing tools (Li et al. 2017a, 2019; Siegl and Luzhetskyy 2012). Therefore, although not so effective, the HR-based genome editing system is more beneficial for biotechnological applications. Several systems for effective marker-free HR-based genome modification have also been developed (Myronovskyi et al. 2014; Rezuchova et al. 2018). Efficiency issues in HR-based methodology have been dramatically improved by introducing a revolutionary DBS-generating CRISPR/Cas9 methodology in accurate and programmable Streptomyces chromosome positions that HR can correct, resulting in different editing events (deletions, insertions). Therefore, this CRISPR/Cas9-based methodology appears to be a very promising tool for various stable biotechnological applications. All the HR-based genome editing systems are summarized in Table 2.
References
Alexander DC, Rock J, He X, Miao V, Brian P, Baltz RH (2010) Development of a genetic system for combinatorial biosynthesis of lipopeptides in Streptomyces fradiae and heterologous expression of the A54145 biosynthetic gene cluster. Appl Environ Microbiol 76:6877–6887
Anne J, Maldonado B, van Impe J, Mellart v, Bernaerts K (2012) Recombinant protein production and streptomycetes. J Biotech 158:159–167
Bachmann BO, van Lanen SG, Baltz RH (2014) Microbial genome mining for accelerated natural products discovery: is a renaissance in the making? J Ind Microbiol Biotechnol 41:175–184
Bailey CR, Bruton CJ, Butler MJ, Chater KF, Harris JE, Hopwood DA (1986) properties of in vitro recombinant derivatives of pJV1, a multi-copy plasmid from Streptomyces phaeochromogenes. J Gen Microbiol 132:2071–2078
Baltz RH (1998) Genetic manipulation of antibiotic-producing Streptomyces. Trends Microbiol 6:76–83
Baltz RH (2012) Streptomyces temperate bacteriophage integration systems for stable genetic engineering of actinomycetes (and other organisms). J Ind Microbiol Biotechnol 39:661–672
Baltz RH (2016) Genetic manipulation of secondary metabolite biosynthesis for improved production in Streptomyces and other actinomycetes. J Ind Microbiol Biotechnol 43:343–370
Barka EA, Vatsa P, Sanchez L, Gaveau-Vaillant N, Jacquard C, Klenk H-P, Clément C, Ouhdouch Y, van Wezel GP (2016) Taxonomy, physiology, and natural products of Actinobacteria. Microbiol Mol Biol Rev 80:1–43
Bekiesch P, Basitta P, Apel AK (2016) Challenges in the heterologous production of antibiotics in Streptomyces. Arch Pharm Chem Life Sci 349:594–601
Bibb MJ, Freeman RF, Hopwood DA (1977) Physical and genetical characterization of a second sex factor, SCP2, for Streptomyces coelicolor A3(2). Mol Gen Genet 154:155–166
Bibb MJ, Ward JM, Hopwood DA (1978) Transformation of plasmid DNA into Streptomyces at high frequency. Nature 274:398–400
Bierman M, Logan R, O’Brien K, Seno ET, Rao RN, Schoner BE (1992) Plasmid cloning vectors for conjugal transfer of DNA from Escherichia coli to Streptomyces spp. Gene 116:43–49
Bilyk O, Sekurova O, Zotchev SB, Luzhetskyy A (2016) Cloning and heterologous expression of the grecocycline biosynthetic gene cluster. PLoS One 11(7):e0158682
Boccard F, Smokvina T, Pernodet JL, Friedmann A, Guérineau M (1989) The integrated conjugative plasmid pSAM2 of Streptomyces ambofaciens is related to temperate bacteriophages. EMBO J 8:973–980
Busche T, Novakova R, Al'Dilaimi A, Homerova D, Feckova L, Rezuchova B, Mingyar E, Csolleiova D, Bekeova C, Winkler A, Sevcikova B, Kalinowski J, Kormanec J, Rückert C (2018) Complete genome sequence of Streptomyces lavendulae subsp. lavendulae CCM 3239 (formerly “Streptomyces aureofaciens CCM 3239”), a producer of the angucycline-type antibiotic auricin. Genome Announc 6:e00103–e00118
Chater KF, Carter AT (1979) A new, broad host-range, temperate bacteriophage (R4) of Streptomyces and its interaction with some restriction-modification systems. J Gen Microbiol 115:431–442
Chen J, Wu Q, Hawas UW, Wang H (2016) Genetic regulation and manipulation for natural product discovery. Appl Microbiol Biotechnol 100:2953–2965
Cobb RE, Wang Y, Zhao H (2015) High-efficiency multiplex genome editing of Streptomyces species using an engineered CRISPR/Cas system. ACS Synth Biol 4:723–728
Combes P, Till R, Bee S, Smith MCM (2002) The Streptomyces genome contains multiple pseudo-attB sites for the ΦC31-encoded site-specific recombination system. J Bacteriol 184:5746–5752
Costes A, Lambert SAE (2013) Homologous recombination as a replication fork escort: fork-protection and recovery. Biomolecules 3:39–71
Datsenko KA, Wanner BL (2000) One-step inactivation of chromosomal genes in Escherichia coli K-12 using PCR products. Proc Natl Acad Sci U S A 97:6640–6645
Deng Y, Zhang X, Zhang Y (2017) Recent advances in genetic modification systems for Actinobacteria. Appl Microbiol Biotechnol 101:2217–2226
Denis F, Brzezinski R (1992) A versatile shuttle cosmid vector for use in Escherichia coli and actinomycetes. Gene 111:115–118
Dubeau MP, Ghinet MG, Jacques PE, Clermont N, Beaulieu C, Brzezinski R (2009) Cytosine deaminase as a negative selection marker for gene disruption and replacement in the genus Streptomyces and other Actinobacteria. Appl Environ Microbiol 75:1211–1214
Dyson PJ (2011) Streptomyces: molecular biology and biotechnology. Caister Academic Press, Norfolk
Farkasovska J, Godany A (2012) Analysis of the site-specific integration system of the Streptomyces aureofaciens phage μ1/6. Curr Microbiol 64:226–233
Fayed B, Younger E, Taylor G, Smith MCM (2014) A novel Streptomyces spp. integration vector derived from the S. venezuelae phage, SV1. BMC Biotechnol 14:51
Fayed B, Ashford DA, Hashem AM, Amin MA, El Gazayerly ON, Gregory MA, Smith MCM (2015) Multiplexed integrating plasmids for engineering of the erythromycin gene cluster for expression in Streptomyces spp. and combinatorial biosynthesis. Appl Environ Microbiol 81:8402–9413
Fedoryshyn M, Petzke L, Welle E, Bechthold A, Luzhetskyy A (2008a) Marker removal from actinomycetes genome using Flp recombinase. Gene 419:43–47
Fedoryshyn M, Welle E, Bechthold A, Luzhetskyy A (2008b) Functional expression of the Cre recombinase in actinomycetes. Appl Microbiol Biotechnol 78:1065–1070
Fernandez-Martinez LT, Bibb MJ (2014) Use of the Meganuclease I-SceI of Saccharomyces cerevisiae to select for gene deletions in actinomycetes. Sci Rep 4:7100
Fogg PCM, Colloms S, Rosser S, Stark WM, Smith MCM (2014) New applications for phage integrases. J Mol Biol 426:2703–2716
Fogg PCM, Haley JA, Stark WM, Smith MCM (2017) Genome integration and excision by a new Streptomyces bacteriophage, ΦJoe. Appl Environ Microbiol 83:e02767–e02716
Gonzalez-Quiñonez N, López-García MT, Yagüe P, Rioseras B, Pisciotta A, Alduina R, Manteca A (2016) New ΦBT1 site-specific integrative vectors with neutral phenotype in Streptomyces. Appl Microbiol Biotechnol 100:2797–2808
Gregory MA, Till R, Smith MCM (2003) Integration site for Streptomyces phage FBT1 and development of site-speciWc integrating vectors. J Bacteriol 185:5320–5323
Gross H (2007) Strategies to unravel the function of orphan biosynthesis pathways: recent examples and future prospects. Appl Microbiol Biotechnol 75:267–277
Gust B, Challis GL, Fowler K, Kieser T, Chater KF (2003) PCR-targeted Streptomyces gene replacement identifies a protein domain needed for biosynthesis of the esquiterpene soil odor geosmin. Proc Natl Acad Sci U S A 18:1541–1548
Hamed MB, Anne J, Karamanou S, Economou A (2018) Streptomyces protein secretion and its application in biotechnology. FEMS Microbiol Lett 265:fny250
Hermann S, Siegl T, Luzhetska M, Petzke L, Jilg C, Welle E, Erb A, Leadlay PF Bechthold A, Luzhetskyy A (2012) Site-specific recombination strategies for engineering actinomycete genomes. Appl Environ Microbiol 78:1804–1812
Hopwood DA (2007) Streptomyces in nature and mecicine. The antibiotic makers. Oxford University Press, New York
Hosted TJ, Baltz RH (1997) Use of rpsL for dominance selection and gene replacement in Streptomyces roseosporus. J Bacteriol 179:180–186
Hover BM, Kim S-H, Katz M, Charlop-Powers Z, Owen JG, Ternei MA, Maniko J, Estrela A, Molina H, Park S, Perlin DS, Brady SF (2018) Culture-independent discovery of the malacidins as calcium-dependent antibiotics with activity against multidrug-resistant Gram-positive pathogens. Nat Microbiol 3:415–422
Huang H, Zheng G, Jiang W, Hu H, Lu Y (2015) One-step high-efficiency CRISPR/Cas9-mediated genome editing in Streptomyces. Acta Biochim Biophys Sin 47:231–243
Huo L, Hug JJ, Fu C, Bian X, Zhang Y, Muller R (2019) Heterologous expression of bacterial natural product biosynthetic pathways. Nat Prod Rep. https://doi.org/10.1039/c8np00091c
Jiang W, Zhao X, Gabrieli T, Lou C, Ebenstein Y, Zhu TF (2015) Cas9-assisted targeting of chromosome segments CATCH enables one-step targeted cloning of large gene clusters. Nat Commun 6:8101
Khaleel T, Younger E, McEwan AR, Varghese AS, Smith MCM (2011) A phage protein that binds ΦC31 integrase to switch its directionality. Mol Microbiol 80:1450–1463
Kieser T, Hopwood DA, Wright HM, Thompson CJ (1982) pIJ101, a multi-copy broad host-range Streptomyces plasmid: functional analysis and development of DNA cloning vectors. Mol Gen Genet 186:223–228
Kieser T, Bibb MJ, Buttner MJ, Chater KF, Hopwood DA (2000) Practical Streptomyces genetics. The John Innes Foundation, Norwich
Kim JH, Feng Z, Bauer JD, Kallifidas D, Cale PY, Brady SF (2010) Cloning large natural product gene clusters from the environment: piecing environmental DNA gene clusters back together with TAR. Biopolymers 93:833–844
Knirschova R, Novakova R, Mingyar E, Bekeova C, Homerova D, Kormanec J (2015) Utilization of a reporter system based on the blue pigment indigoidine biosynthetic gene bpsA for detection of promoter activity and deletion of genes in Streptomyces. J Microbiol Methods 113:1–3
Kormanec J, Rezuchova B, Farkasovsky M (1993) Optimization of Streptomyces aureofaciens transformation and disruption of the hrdA gene encoding a homologue of the principal sigma factor. J Gen Microbiol 139:2525–2529
Kuhstoss S, Richardson MA, Rao RN (1989) Site-specific integration in Streptomyces ambofaciens: localization of integration functions in S. ambofaciens plasmid pSAM2. J Bacteriol 171:16–23
Kuhstoss S, Richardson MA, Rao RN (1991) Plasmid cloning vectors that integrate site-specifically in Streptomyces spp. Gene 97:143–146
Lee NC, Larionov V, Kouprina N (2015) Highly efficient CRISPR/Cas9-mediated TAR cloning of genes and chromosomal loci from complex genomes in yeast. Nucleic Acids Res 43:e55
Li P, Li J, Guo Z, Tang W, han J, meng X, Hao T, Zhu Y, Zhang L, Chen Y (2015) An efficient blue-white screening based gene inactivation system for Streptomyces. Appl Microbiol Biotechol 99:1923–1933
Li L, Zheng G, Chen J, Ge M, Jiang W, Lu Y (2017a) Multiplexed site-specific genome engineering for overproducing bioactive secondary metabolites in actinomycetes. Metab Eng 40:80–92
Li L, Jiang W, Lu Y (2017b) New strategies and approaches for engineering biosynthetic gene clusters of microbial natural products. Biotechnol Adv 35:936–949
Li L, Wei K, Liu X, Wu Y, Zheng G, Chen S, Jiang W, Lu Y (2019) aMSGE: advanced multiplex site-specific genome engineering with orthogonal modular recombinases in actinomycetes. Metab Eng 52:153–167
Liu H, Jiang H, Haltli B, Kulowski K, Muszynska E, Feng X, Summers M, Young M, Graziani E, Koehn F, Carter GT, He M (2009) Rapid cloning and heterologous expression of the meridamycin biosynthetic gene cluster using a versatile Escherichia coli-Streptomyces artificial chromosome vector, pSBAC. J Nat Prod 72:389–395
Liu R, Deng Z, Liu T (2018) Streptomyces species: ideal chassis for natural product discovery and overproduction. Metab Eng 50:74–84
Lomovskaya ND, Mkrtumian N, Gostimskaya NL, Danilenko VN (1972) Characterization of temperate actinophage ΦC31 isolated from Streptomyces coelicolor A3(2). J Virol 9:258–262
Lomovskaya ND, Chater KF, Mkrtumian N (1980) Genetics and molecular biology of Streptomyces bacteriophages. Microbiol Rev 44:206–229
Manderscheid N, Bilyk B, Busche T, Kalinowski J, Paululat T, Bechthold A, Petzke L, Luzhetskyy A (2016) An influence of the copy number of biosynthetic gene clusters on the production level of antibiotics in a heterologous host. J Biotech 323:110–117
Matsuura M, Noguchi T, Yamaguchi D, Aida T, Asayama M, Takahashi H, Shirai M (1996) The sre gene (ORF469) encodes a site-specific recombinase responsible for integration of the R4 phage genome. J Bacteriol 178:3374–3376
Mazodier P, Petter R, Thompson C (1989) Intergenic conjugation between Escherichia coli and Streptomyces species. J Bacteriol 171:3583–3585
Miura T, Hosaka Y, Yan-Zhuo Y, Nishizawa T, Asayama M, Takahashi H, Shirai M (2011) In vivo and in vitro characterization of site specific recombination of actinophage R4 integrase. J Gen Appl Microbiol 57:45–57
Miura T, Nishizawa A, Nishizawa T, Asayama M, Takahashi H, Shirai M (2014) Construction of a stepwise gene integration system by transient expression of actinophage R4 integrase in cyanobacterium Synechocystis sp. PCC 6803. Mol Gen Genomics 289:615–623
Morita K, Yamamoto T, Fusada N, Komatsu M, Ikeda H, Hirano N, Takahashi H (2009) The site-specific recombination system of actinophage TG1. FEMS Microbiol Lett 297:234–240
Muth G, Nussbaumer B, Wohlleben W, Puhler A (1989) A vector system with temperature-sensitive replication for gene disruption and mutational cloning in streptomycetes. Mol Gen Genet 219:341–348
Myronovskyi M, Luzhetskyy A (2013) Genome engineering in actinomycetes using site-specific recombinases. Appl Microbiol Biotechnol 97:4701–4712
Myronovskyi M, Rosenkranzer B, Luzhetskyy A (2014) Iterative marker excision system. Appl Microbiol Biotechnol 98:4557–4570
Myronovskyi M, Rosenkranzer B, Nadmid S, Pujic P, Normand P, Luzhetskyy A (2018) Generation of a cluster-free Streptomyces albus chassis strains for improved heterologous expression of secondary metabolite clusters. Metab Eng 49:316–324
Nah H-J, Pyeon H-R, Kang S-H, Choi S-S, Kim E-S (2017) Cloning and heterologous expression of a large-sized natural product biosynthetic gene cluster in Streptomyces species. Front Microbiol 8:394
Novakova R, Rehakova A, Feckova L, Kutas P, Knirschova R, Kormanec J (2011) Genetic manipulation of pathway regulation for overproduction of angucycline-like antibiotic auricin in Streptomyces aureofaciens CCM 3239. Folia Microbiol 56:276–282
Novakova R, Núñez LE, Homerova D, Knirschova R, Feckova L, Rezuchova B, Sevcikova B, Menéndez N, Morís F, Cortés J, Kormanec J (2018) Increased heterologous production of the antitumoral polyketide mithramycin A by Engineered Streptomyces lividans TK24 Strains. Appl Microbiol Biotechnol 102:857–869
Okanishi M, Suzuki K, Umezawa H (1974) Formation and reversion of streptomycete protoplasts: cultural conditions and morphological study. J Gen Microbiol 80:389–400
Olivares EC, Hollis RP, Calos MP (2001) Phage R4 integrase mediates site-specific integration in human cells. Gene 278:167–176
Pigac J, Schrempf H (1995) A simple and rapid method of transformation of Streptomyces rimosus R6 and other streptomycetes by electroporation. Appl Environ Microbiol 61:352–356
Raynal A, Tuphile K, Gerbaud C, Luther T, Guerineau M, Pernodet JL (1998) Structure of the chromosomal insertion site for pSAM2: functional analysis in Escherichia coli. Mol Microbiol 28:333–342
Raynal A, Friedmann A, Tuphile K, Guerineau M, Pernodet JL (2002) Characterisation of the attP site of the integrative element pSAM2 from Streptomyces ambofaciens. Microbiology 148:61–67
Rebets Y, Kormanec J, Lutzhetskyy A, Bernaerts K, Anné J (2017) Cloning and expression of metagenomic DNA in Streptomyces lividans and subsequent fermentation for optimized production. In: Streit W, Daniel R (eds) Metagenomics. Methods and protocols. Methods in Molecular Biology, vol 1539. Springer, New York, pp 99–144
Rezuchova B, Homerova D, Sevcikova B, Núñez LE, Novakova R, Feckova L, Skultety L, Cortés J, Kormanec J (2018) An efficient blue-white screening system for markerless deletions and stable integrations in Streptomyces chromosomes based on the blue pigment indigoidine biosynthetic gene bpsA. Appl Microbiol Biotechnol 102:10231–10244
Rodriguez E, Ward S, Fu H, Revill WP, McDaniel R, Katz L (2004) Engineered biosynthesis of 16-membered macrolides that require methoxymalonyl-ACP precursors in Streptomyces fradiae. Appl Microbiol Biotechnol 66:85–91
Sekurova ON, Brautaset T, Sletta H, Borgos SE, Jakobsen MØM, Ellingsen TE, Strøm AR, Valla S, Zotchev SB (2004) In vivo analysis of the regulatory genes in the nystatin biosynthetic gene cluster of Streptomyces noursei ATCC 11455 reveals their differential control over antibiotic biosynthesis. J Bacteriol 186:1345–1354
Selle K, Barrangou R (2015) Harnessing CRISPR–Cas systems for bacterial genome editing. Trends Microbiol 23:225–232
Sezonov G, Duchêne AM, Friedmann A, Guérineau M, Pernodet JL (1998) Replicase, excisionase, and integrase genes of the Streptomyces element pSAM2 constitute an operon positively regulated by the pra gene. J Bacteriol 180:3056–3061
Siegl T, Luzhetskyy A (2012) Actinomycetes genome engineering approaches. Antonie Van Leeuwenhoek 102:503–516
Siegl T, Petzke L, Welle E, Luzhetskyy A (2010) I-SceI endonuclease: a new tool for DNA repair studies and genetic manipulations in streptomycetes. App Microbiol Biotechnol 87:1525–1532
Smokvina T, Mazodier P, Boccard F, Thompson CJ, Guérineau M (1990) Construction of a series of pSAM2-based integrative vectors for use in actinomycetes. Gene 94(1):53–59
Spasic J, Mandic M, Djokic L, Nikodinovic-Runic J (2018) Streptomyces spp. in the biocatalytic toolbox. Appl Microbiol Biotechnol 102:3513–3536
Tala A, Damianoa F, Gallo G, Pinatel E, Calcagnile M, Testini M, Fico D, Rizzo D, Sutera A, Renzone G, Scaloni A, De Bellis G, Siculella L, De Benedetto GE, Puglia AM, Peano C, Alifano P (2018) Pirin: a novel redox-sensitive modulator of primary and secondary metabolism in Streptomyces. Metab Eng 48:254–268
Tao W, Yang A, Deng Z, Sun Y (2018) CRISPR/Cas9-based editing of Streptomyces for discovery, characterization, and production of natural products. Front Microbiol 9:1660
Tocchetti A, Donadio S, Sossio M (2018) Large inserts for big data: artificial chromosomes in the genomic era. FEMS Microbiol Lett 365:fny064
Tong Y, Charusanti P, Zhang L, Weber T, Lee SY (2015) CRISPR/Cas-based engineering of actinomycetal genomes. ACS Synth Biol 4:1020–1029
Tong Y, Weber T, Lee SY (2018) CRISPR/Cas-based genome engineering in natural product discovery. Nat Prod Rep. https://doi.org/10.1039/c8np00089a
Van Mellaert L, Mei L, Lammertyn E, Schacht S, Anne J (1998) Site-speciWc integration of bacteriophage VWB genome into Streptomyces venezuelae and construction of a VWB-based integrative vector. Microbiology 144:3351–3358
Van Wezel GP, Bibb MJ (1996) A novel plasmid vector that uses the glucose kinase gene (glkA) for the positive selection of stable gene disruptants in Streptomyces. Gene 182:229–230
Wang JW, Wang A, Li K, Wang B, Jin S, Reiser M, Lockey RF (2015) CRISPR/Cas9 nuclease cleavage combined with Gibson assembly for seamless cloning. Biotechniques 58:161–170
Wright WD, Shan SS, Heyer WD (2018) Homologous recombination and the repair of DNA double-strand breaks. J Biol Chem 293:10524–10535
Zeng H, Wen S, Xu W, He Z, Zhai G, Liu Y, Deng Z, Sun Y (2015) Highly efficient editing of the actinorhodin polyketide chain length factor gene in Streptomyces coelicolor M145 using CRISPR/Cas9-CodA(sm) combined system. Appl Microbiol Biotechnol 99:10575–10585
Zhang L, Ou X, Zhao G, Ding X (2008) Highly efficient in vitro site-specific recombination system based on the Streptomyces phage ΦBT1 integrase. J Bacteriol 190:6392–6397
Zhang MM, Wong FT, Wang Y, Luo S, Lim YH, Heng E, Yao WL, Cobb RE, Enghiad B, Ang EL, Zhao H (2017) CRISPR-Cas9 strategy for activation of silent Streptomyces biosynthetic gene clusters. Nat Chem Biol 13:607–609
Zheng JT, Wang SL, Yang KQ (2007) Engineering a regulatory region of jadomycin gene cluster to improve jadomycin B production in Streptomyces venezuelae. Appl Microbiol Biotechnol 76:883–888
Funding
This work was financially supported by the Slovak Research and Development Agency under contract No. APVV-15-0410 and by the VEGA grant 2/0002/16 from the Slovak Academy of Sciences.
Author information
Authors and Affiliations
Corresponding author
Ethics declarations
Conflict of interest
The authors declare that they have no conflict of interest.
Ethical approval
This article does not contain any studies with human participants or animals performed by any of the authors.
Additional information
Publisher’s note
Springer Nature remains neutral with regard to jurisdictional claims in published maps and institutional affiliations.
Rights and permissions
About this article
Cite this article
Kormanec, J., Rezuchova, B., Homerova, D. et al. Recent achievements in the generation of stable genome alterations/mutations in species of the genus Streptomyces. Appl Microbiol Biotechnol 103, 5463–5482 (2019). https://doi.org/10.1007/s00253-019-09901-0
Received:
Revised:
Accepted:
Published:
Issue Date:
DOI: https://doi.org/10.1007/s00253-019-09901-0