Abstract
Parkinson’s disease is the second most common neurodegenerative disorder with selective and progressive decline of nigral dopaminergic neurons. Hypericum perforatum L. (H. perforatum, St. John’s wort) has been traditionally used for management of different disorders, especially mild-to-moderate depression. This study was conducted to evaluate the effect of H. perforatum extract against unilateral striatal 6-hydroxydopamine (6-OHDA) toxicity and to unmask some involved mechanisms. Intrastriatal 6-OHDA-lesioned rats were treated with H. perforatum hydroalcoholic extract at a dose of 200 mg/kg/day started 1 week pre-surgery for 1 week post-surgery. The extract attenuated apomorphine-induced rotational behavior, decreased the latency to initiate and the total time on the narrow beam task, lowered striatal level of malondialdehyde and enhanced striatal catalase activity and reduced glutathione content, normalized striatal expression of glial fibrillary acidic protein, tumor necrosis factor α with no significant effect on mitogen-activated protein kinase, lowered nigral DNA fragmentation, and prevented damage of nigral dopaminergic neurons with a higher striatal tyrosine hydroxylase immunoreactivity. These findings reveal the beneficial effect of H. perforatum via attenuation of DNA fragmentation, astrogliosis, inflammation, and oxidative stress.
Similar content being viewed by others
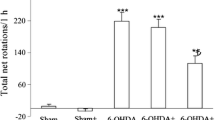
Avoid common mistakes on your manuscript.
Introduction
Parkinson’s disease (PD) is the second most common neurodegenerative disorder which selectively affects mesencephalic dopaminergic neurons of the substantia nigra pars compacta (SNC) with classical symptoms including bradykinesia, postural impairment, resting tremor, and rigidity (Fearnley and Lees 1991; Yacoubian and Standaert 2009). These motor complications are usually difficult to manage, and PD patients also develop a diversity of non-motor symptoms like anosmia, autonomic impairment, sleep disorder, and cognitive impairment which are refractory to most current treatments and usually become the major cause of disability. The complexity of these late-stage complications necessitates the development of new neuroprotective strategies which could be applied in early stages of its development in order to prevent or delay later complications (Yacoubian and Standaert 2009). Several contributing factors including mitochondrial dysfunction, oxidative stress, inflammation, and apoptosis are mainly responsible for PD pathogenesis (Yacoubian and Standaert 2009; Taylor et al. 2013).
Hypericum perforatum L. (H. perforatum, St. John’s wort) has been traditionally used for a wide range of disorders including the treatment of mild-to-moderate depression (Miller 1998; Shelton 2009). In addition, several studies have shown anxiolytic (Ara and Bano 2009) and anti-inflammatory (Hatano et al. 2014) effect of this plant. Enhancing effect of H. perforatum on sodium nitroprusside-mediated neuroprotection under a state of glucose deprivation in PC12 cells has been reported (Munoz et al. 2012). In addition, H. perforatum could minimize oxidative stress, intensify antioxidant defensive system in restraint stress in mice (Kumar et al. 2010), and attenuate amyloid beta-mediated toxicity in microglial cells (Kraus et al. 2007). Meanwhile, it has been shown that H. perforatum is capable to mitigate striatal astrogliosis, restore monoamine oxidase-B (MAO-B) activity in 1-methyl-4-phenyl-1,2,3,6-tetrahydropyridine (MPTP) model of PD in mice (Mohanasundari and Sabesan 2007), and to exert protective effect in different toxic conditions (Sanchez-Reus et al. 2007; Mohanasundari et al. 2006; Benedi et al. 2004; Gomez del Rio et al. 2013). On this foundation, we designed this study to evaluate the neuroprotective potential of H. perforatum extract in 6-OHDA-induced model of PD in the rat and to explore in more detail the involvement of some underlying mechanisms.
Materials and Methods
Plant Material and Extract Preparation
Hypericum perforatum was procured from Isfahan Natural Resource Institute in July and authenticated by Dr. Ghaem-Maghami at Isfahan University Herbarium, and a voucher specimen number 13,648 was assigned. Aerial parts of the plant were dried for 1 week at room temperature under shade and were powdered by an electric grinder. The extract was prepared with 10 g of powder and 100 ml of 70 % ethanol as the solvent. The extraction was done using maceration method for 72 h at room temperature and in a dark place. Afterwards, the solution was filtered three times and dried on a rotary evaporator at 40 °C, yielding 2.14 g (21.4 %) of extract. The final extract was kept at −20 °C and further dilution was prepared in cold normal saline. The major chemical ingredients of the extract were assessed using high-performance liquid chromatography (HPLC) (SPD-10AVP; Shimadzu, Kyoto, Japan) with ODS column and determined as 0.37 % hypericin, 3.1 % hyperforin, 4.3 % flavonoids like isoquercitrin, kaempferol, luteolin, myricetin, quercetin, and rutin, and 10 % tannins in addition to polysaccharides including maltodextrins (Darupakhsh Research Group, Tehran).
Animals
Male adult Wistar rats (205–265 g; n = 48; Pasteur’s Institute, Tehran, Iran) were kept in a temperature-controlled animal house with 12/12 light–dark cycle. All protocols for the use and care of animals were approved by Ethics Committee of Shahed University (Tehran, Iran) in 2013 as stipulated by NIH. Rats were randomly assigned to 4 groups, i.e., sham, H. perforatum-treated sham, lesion group (6-OHDA), and H. perforatum-treated 6-OHDA. For induction of PD, the neurotoxin 6-OHDA was injected into the left striatum of rats (anesthetized with a combination of ketamine 80 mg/kg and xylazine 8 mg/kg, i.p.) fixed in a stereotaxic apparatus (Stoelting, USA) and the coordinates 3 mm lateral and 0.2 mm anterior to bregma and ventrally 5 mm below the dura (Paxinos and Watson 1986). The 6-OHDA group received 5 μl of 0.9 % saline containing 2.5 μg/μl of 6-OHDA HCl (Sigma-Aldrich, USA) and 0.2 % ascorbate. The sham group received only ascorbate-saline solution. The H. perforatum-treated 6-OHDA group received 6-OHDA in addition to H. perforatum extract i.p. at a dose of 200 mg/kg/day started 1 week pre-surgery for 1 week post-surgery. Extract dose was chosen from a previous study on its antidepressant and anxiolytic activity in diabetic rats (Husain et al. 2011).
Behavioral Assessment
All the behavioral experiments were done 1 week post-surgery (n = 11–12 for each group) and conducted between 09 a.m. and 02 p.m.
Rotational Behavior
The rotations were evaluated by a method as described previously (Roghani et al. 2010). Briefly, the animals were allowed to be adapted for 10 min in lab room, and 1 min after the injection of apomorphine hydrochloride (2 mg/kg, i.p.), full clockwise and counterclockwise rotations were obtained in a cylindrical container with a diameter of 33 cm and a height of 35 cm for 1 h. Then, net number of rotations was calculated as positive scores minus negative ones.
Elevated Narrow Beam Test
The protocol for this test has been described before in the literature (Allbutt and Henderson 2007). The narrow beam consisted of a wooden beam with a length of 105 cm, a width of 4 cm, and a height of 3 cm. It was placed at a height of 80 cm above the ground. The wooden supports at the “starting” end of the beam formed a sheer drop, while a platform was located at the other end, next to which the home cage of the rat being tested was placed. Beneath the beam, a soft foam cushion was placed to prevent animal’s injury on falling. At the starting line, a marker was drawn 20 cm from its end. During the test, the rat was placed within the starting zone and upon animal release, time was measured. This time represented the latency to begin the task. The stopwatch was then stopped when all four feet were placed entirely upon the finishing line at the opposite end of the beam. The maximum time allowed for the task was considered 2 min. The starting line was to be crossed within 1 min from the release or the test was canceled, and maximum time was recorded for that trial. A fall was also recorded as a maximum time. A testing session consisted of five trials on the beam.
Oxidative Stress Assessment
One week post-surgery, left and right striatal tissues (n = 7 for each group) were separately dissected out and 5 % homogenate was separately prepared in lysate buffer and in the presence of protease inhibitor cocktail, and the supernatant was aliquoted and stored at −70 °C for the following experiments.
Determination of MDA, Nitrite, and Protein Content
MDA and nitrite concentration in the supernatant was measured as described before (Afshin-Majd et al. 2015). For determination of MDA concentration (thiobarbituric acid-reactive substances, TBARS), trichloroacetic acid and TBARS reagent were added to the supernatant, then mixed, and incubated at boiling water for 90 min. After cooling on ice, samples were centrifuged at 1000×g for 10 min and the absorbance of the supernatant was read at 532 nm. The results were obtained on tetraethoxypropane standard curve. For measurement of nitrite concentration, Griess reagent including sulfanilamide and N-naphthyl ethylenediamine was used, the absorbance was read at 540 nm, and the concentration was obtained on sodium nitrite standard curve. Bradford method was used for determination of protein content using bovine serum albumin as its standard (Bradford 1976).
Assay of Catalase Activity
For this purpose, the Claiborne’s method was used (Claiborne 1985). Briefly, H2O2 was added to a mixture of 50 mM potassium phosphate buffer (pH 7.0) and supernatant, and the rate of H2O2 decomposition was assessed by measuring the absorbance changes at 240 nm.
Reduced Glutathione Measurement (GSH)
GSH was measured as described before (Sedlak and Lindsay 1968; Ellman 1959). Briefly, the supernatant was centrifuged with 5 % trichloroacetic acid. To 0.1 ml of homogenate, 2 ml of phosphate buffer (pH 8.4), 0.5 ml of 5′,5-dithiobis (2-nitrobenzoic acid) (DTNB), and 0.4 ml of distilled water were added, and the absorbance was read at 412 nm.
Determination of Striatal GFAP, MAPK, and TNFα Expression
The GFAP, MAPK, and TNFα levels (n = 6 for each group) in the striatal supernatant were measured using sandwich enzyme-linked immunosorbent assay and commercial kits according to the manufacturer’s instructions (Cloud-Clone Corp., Houston, USA). The absorbance of samples was read at 450 nm by Synergy HT microplate reader (BioTek, USA), and the values were expressed as their final concentration.
Determination of DNA Fragmentation
DNA breakdown into 200-bp nucleosomal fragments and DNA condensation are reliable hallmarks of apoptosis (Cohen 1997). In this series of experiments (n = 6 for each group), 1 week after the lesion, 5 % supernatant of left substantia nigra was prepared in cold normal saline and protease inhibitor cocktail, and DNA fragments were measured using the Cell Death Detection ELISA kit (Roche Diagnostics, Germany) to measure histone-associated DNA fragments (mono- and oligonucleosomes) as an indicator of induced cell death (apoptosis) as described before (Morroni et al. 2013) by a microplate reader (BioTek, USA) to read OD of samples.
Tyrosine Hydroxylase (TH) Immunohistochemistry
Some rats (n = 5 for each group) were deeply anesthetized with ketamine (150 mg/kg) and transcardially perfused with normal saline followed by 4 % paraformaldehyde in 0.1 M phosphate buffer (PB, pH 7.4). After immersion in 30 % sucrose, 30-μm sections were cut on a freezing microtome (Leica, Germany). The sections on gelatin-coated slides were incubated in 0.1 % sodium borohydride in phosphate-buffered saline (PBS, pH 7.4), methanol containing 0.03 % H2O2, then in Triton X100 (0.03 %), and 0.1 % bovine serum albumin in PBS, with rinsing 2–3 times in the PBS between the steps. Then, sections were incubated with primary polyclonal rabbit anti-TH antibody (Sigma-Aldrich, USA, 1/200) in PBS overnight and then with secondary anti-rabbit IgG-peroxidase antibody raised in goat (Sigma-Aldrich, USA) in PBS for 2 h. To reveal the bound peroxidase, the sections were incubated in diaminobenzidine tetrahydrochloride (DAB; 10 mg/20 ml of PBS and 0.03 % H2O2) for 5–8 min, rinsed, dehydrated, cleared, and coverslipped.
Histological Evaluation
Counting of TH-positive neurons within SNC was done according to methods of previous reports (Zare et al. 2015; Healy-Stoffel et al. 2014). Briefly, using rat brain atlas (Paxinos and Watson 1986), the borders of the SNC (interaural 2.9–4.2 mm) were carefully outlined in rostrocaudal sections at low magnification to exclude the pars reticulata (SNR) and the ventral tegmental area. Neurons were counted only when the soma was present and within the focus. Counting was done blind to the treatments. All counting was done two times and its average was obtained.
Intensity of striatal TH immunoreactivity on the left side was measured by a semi-quantitative densitometric analysis method using an image analysis program (ImageJ software, NIH, MD, USA; Version 1.49) and according to a previous report (Shinko et al. 2014) with some modifications. In this regard, at least five sections at 0.2 ± 0.8 mm relative to the bregma (Paxinos and Watson 1986) were randomly selected for quantitative evaluation, and their optical density was calculated.
Statistical Analysis
Data were reported as means ±standard error. For statistical evaluation of data, the parametric one-way ANOVA test followed by Tukey’s post hoc test was used. In all analyses, the null hypothesis was rejected at a level of 0.05.
Results
Regarding the rotational behavior, 6-OHDA-lesioned group exhibited significant contralateral rotations versus sham (p < 0.005), and H. perforatum extract significantly reduced these contralateral rotations versus 6-OHDA group (p < 0.05) (Fig. 1). Evaluation of animal performance in narrow beam test (Fig. 2) showed that the latency and the total crossing time were significantly higher in 6-OHDA group versus sham (p < 0.01–0.005) and H. perforatum significantly reduced this (p < 0.05).
The effect of H. perforatum extract on latency to initiate crossing and on total time to cross the beam in narrow beam task 1 week after intrastriatal 6-hydroxydopamine (6-OHDA) injection. Values are expressed as mean ± S.E.M. n = 11 for each group. *p < 0.05, **p < 0.01, ***p < 0.005 (vs. sham); ǂ p < 0.05 (vs. 6-OHDA)
Measurement of oxidative stress markers (Fig. 3a–d) showed that 6-OHDA-lesioned rats have a significantly elevated level of striatal MDA (p < 0.05) and significantly lower striatal catalase activity (p < 0.05) and GSH content (p < 0.01) versus sham with no significant change of striatal nitrite, and H. perforatum treatment of 6-OHDA group significantly reversed these changes (p < 0.05) relative to 6-OHDA-lesioned group.
The effect of H. perforatum extract on striatal level of malondialdehyde (MDA)(a), catalase activity (b), nitrite (c), and reduced glutathione (d) (GSH) 1 week after intrastriatal 6-hydroxydopamine (6-OHDA) injection. The measurements for each sample were made in duplicate. Data are shown as mean ± S.E.M. n = 7 for each group. *p < 0.05, **p < 0.01 (vs. Sham), ǂ p < 0.05 (vs. 6-OHDA)
Striatal measurement of GFAP as a specific index of astrogliosis and MAPK and TNFα as inflammation-related markers (Fig. 4a–c) revealed that 6-OHDA group has a significantly higher level of GFAP (p < 0.01), MAPK (p < 0.05), and TNFα (p < 0.01) as compared to sham and extract treatment of 6-OHDA group significantly restored these changes (p < 0.05) with an exception for MAPK. Determination of apoptosis with the measurement of nigral DNA fragmentation indicated that 6-OHDA group has a significantly higher level of apoptosis relative to sham (p < 0.01) and extract treatment significantly attenuated this (p < 0.05) (Fig. 4d).
The effect of H. perforatum extract on striatal expression of glial fibrillary acidic protein (a) (GFAP), MAPK (b), and TNFα (c) and on nigral DNA fragmentation as an index of apoptosis in ELISA (d) 1 week after intrastriatal 6-hydroxydopamine (6-OHDA) injection. The measurements for each sample were made in duplicate. Data are shown as mean ± S.E.M. n = 6 for each group. *p < 0.05, **p < 0.01 (vs. Sham), ǂ p < 0.05 (vs. 6-OHDA)
The results of TH immunohistochemistry indicated a significant reduction in TH-positive neurons in SNC (Fig. 5) and a significantly lower TH immunoreactivity in neostriatum (Fig. 6) of 6-OHDA group versus sham (p < 0.01), and extract was able to significantly prevent neuronal loss and caused a significantly higher TH immunoreactivity (p < 0.05) relative to 6-OHDA group.
The effect of H. perforatum extract on the number of tyrosine hydroxylase (TH)-positive neurons on the left side of substantia nigra pars compacta (SNC) and coronal section photomicrographs through the midbrain 1 week after intrastriatal 6-hydroxydopamine (6-OHDA) injection. (SNR substantia nigra pars reticulata). Data are shown as mean ± S.E.M. n = 5 for each group. *p < 0.01 (vs. Sham);ǂ p < 0.05 (vs. 6-OHDA)
Semi-quantitative analysis of tyrosine hydroxylase (TH) immunoreactivity intensity as optical density and representative photomicrographs of coronal sections through neostriatum showing TH immunostaining. Data are shown as mean ± S.E.M. n = 5 for each group. **p < 0.01 (vs. Sham); ǂ p < 0.05 (vs. 6-OHDA)
Discussion
In this study, H. perforatum treatment of 6-OHDA group reduced motor asymmetry and improved animal performance in narrow beam task via protection of SNC neurons with a higher striatal TH immunoreactivity and mitigation of astrogliosis, apoptosis, inflammation, and oxidative stress and by potentiation of antioxidant defensive system.
Although it has been shown that the standardized extract of H. perforatum with antidepressant property could acutely and dose dependently (at doses from 30 to 90 mg/kg) increase locomotor activity and decrease immobility time in forced swimming test (Bukhari and Dar 2013), we did not observe its significant effect in apomorphine-induced rotational and narrow beam tests in extract-treated sham group. This discrepancy with our results may be attributed to its U-shaped dose–response curve and a possible adaptive mechanism due to its longer exposure in this research which itself needs more investigation. The neurotoxin 6-OHDA is routinely used for modeling PD in rodents through selective degeneration of mesencephalic dopaminergic neurons (Schober 2004). The unilateral damage of the nigrostriatal dopaminergic system following intrastriatal injection of 6-OHDA is followed by the reduction of dopamine level in the striatum and ensuing upregulation of post-synaptic dopaminergic receptors. These changes lead to prominent motor asymmetry, as observed in our study by significant contralateral rotations in 6-OHDA group (Schwarting and Huston 1997). A lower rotational behavior in our study may be attributed to the potential of H. perforatum extract in protection of dopaminergic neurons and in this way to keep striatal dopamine level at a level that is not concomitant with marked motor asymmetry in rotational test. Although we did not measure striatal dopamine level in this study, it has been shown that H. perforatum extract low in hyperforin content could inhibit dopamine re-uptake in rat striatal slices via inhibition of respective transporters (Ruedeberg et al. 2010). In addition, H. perforatum extract administration for 15 days at a dose of 100 mg/kg to male rats could have a direct dopaminergic activity and in this way affect prolactin secretion (Di Carlo et al. 2005). Furthermore, hydroalcoholic extract of H. perforatum could preferentially increase extracellular dopamine levels in the rat prefrontal cortex and in this way enhance dopaminergic transmission (Yoshitake et al. 2004). However, these issues need further investigation in future studies to find out its exact mode of action. On the other hand and in consistent with our finding, H. perforatum extract has been found to exhibit neuroprotective property in rat rotenone model of PD through diminishing dopaminergic neuronal death and inhibition of the apoptotic cascade by decreasing level of pro-apoptotic Bax (Gomez del Rio et al. 2013). Moreover, bioactive flavonoid compounds isolated from H. perforatum like hyperoside are capable to protect primary rat cortical neurons against neurotoxicity induced by amyloid β protein through inhibition of apoptosis and attenuation of mitochondrial dysfunction and oxidative stress (Zeng et al. 2011). Other reports also exist on protective potential of this plant and its active constituents (Breyer et al. 2007; Mohanasundari and Sabesan 2007; Munoz et al. 2012; Silva et al. 2008). Assessment of performance of 6-OHDA-lesioned rats in narrow beam test showed an increase of latency and total time on the beam relative to sham group, indicating that existing dopamine depletion in the striatum resulted in both an increased delay in initiating the task and a lower speed in crossing the beam, which would be indicating bradykinesia and/or akinesia (Allbutt and Henderson 2007) and that H. perforatum was also able to attenuate and partly normalize these changes.
Oxidative stress is a critical determinant for the survival and maintenance of dopaminergic neurons in PD. Excessive free radical generation may cause cell death. In addition, auto-oxidation of dopamine may produce dopamine quinine (Lotharius and Brundin 2002). Formation of free radicals like semiquinones could damage cellular constituents (und Halbach et al. 2004). On this basis, some therapeutic approaches are focused on mitigation of oxidative stress. Free radical scavengers may also be of benefit in the maintenance of dopaminergic neurons (Chen and Le 2006). H. perforatum could have attenuated 6-OHDA neuronal insult through counteracting oxidative stress that is consistent with previous reports on its antioxidant ability as well as inhibition of oxidative stress (Kumar et al. 2010; Munoz et al. 2012; Naziroglu et al. 2014a). In this regard, part of oxidative stress attenuation by H. perforatum has been attributed to its effects on modulation of NADPH oxidase and protein kinase C and modification of gating of voltage-gated calcium and TRPM2 channels (Naziroglu et al. 2014a, b). In addition, H. perforatum could alter the balance between the scavenging capacity of antioxidant defense system and free radicals in favor of the antioxidant defensive system (Bayramoglu et al. 2013).
Inflammation is regarded as a causative factor in pathogenesis of PD (Zhou et al. 2007; Miklossy et al. 2006). Pro-inflammatory cytokines freed from glial cells could stimulate nitric oxide generation and exert a damaging effect on dopaminergic neurons by activating receptors that contain intra-cytoplasmic death domains involved in apoptotic pathway (Sriram and O’Callaghan 2007). H. perforatum has been shown to exert anti-inflammatory effect (Hizli et al. 2014) through inhibition of lipoxygenase and cytosolic phospholipase A2, two important enzymes involved in the prostaglandin E2-mediated inflammatory responses, in this way blocking pro-inflammatory mediators but not enhancing inflammation-suppressing mediators (Hammer et al. 2008). Therefore, H. perforatum through lowering the level of inflammatory mediators within the brain contributes to neuroprotection in 6-OHDA-induced PD in rats, as observed in our study by a lower level of striatal TNFα. Apoptosis is also another contributing factor that plays an important role when cells are exposed to neurotoxins like 6-OHDA (Hwang and Chun 2012). In our study, H. perforatum was able to reduce nigral DNA fragmentation that has also been reported in literature regarding its constituents like hyperforin (Gomez del Rio et al. 2013; Hostanska et al. 2003). In our study, enhanced GFAP expression was observed in 6-OHDA-lesioned group that is suggestive of and is related to an inflammatory phenomenon in the injured striatum that has also been reported before (Calou et al. 2014), and H. perforatum extract treatment attenuated this inappropriate change that is to some extent related to its anti-inflammatory property. Previously, it has been shown that H. perforatum could inhibit activity of monoamine oxidase-B and reduce astrocyte activation following MPTP (Mohanasundari and Sabesan 2007).
In this study, we administered H. perforatum hydroalcoholic extract i.p. to hemiparkinsonian rats and observed an improvement in some behavioral, histological, and neurochemical variables. One issue that remains to be answered in such studies is the passage of extract constituents through the blood–brain barrier. The previous studies have shown that 4 h after feeding an oral dose of an alcoholic H. perforatum extract, some of its effective components like quercetin and isorhamnetin/tamarixetin are present in the rat brain (Paulke et al. 2006). However, it is noteworthy that its main constituent, i.e., hyperforin, poorly passes through this barrier (Cervo et al. 2002). According to existing data, it has been known that H. perforatum extract is capable to attenuate beta amyloid-induced histopathology and alleviate memory impairments in amyloid precursor protein-transgenic mice that is apparently independent of its main constituent hyperforin (Hofrichter et al. 2013). Recent studies indicated low bioavailability of hyperforin after oral administration so that minute amounts could be detected intracerebrally after its oral administration (Biber et al. 1998; Keller et al. 2003). Thus, it is possible that its other constituents have played a beneficial role in our study.
One of the cellular targets for H. perforatum extract ingredients is microglia. Kraus et al. have reported that treatment with extract of this may improve microglial viability, thereby attenuating severity of neurotoxic insults (Kraus et al. 2007). Research evidence indicates that neuroinflammation plays an important role in PD with the presence of activated microglia and reactive astrocytes (Teismann and Schulz 2004). A similar inflammatory pattern has been found in the rat substantia nigra after the striatal injection of 6-OHDA (Walsh et al. 2011). Activated microglia, as well as to a lesser extent reactive astrocytes, are found in the area associated with cell loss, possibly contributing to the inflammatory process (Teismann and Schulz 2004). Therefore, microglia may have been affected by H. perforatum extract in our study.
H. perforatum extract constituents could affect blood–brain barrier transporters (Mrozikiewicz et al. 2014) and have interaction with P-glycoproteins of this barrier for overcoming the passage issue (Ott et al. 2010). However, little pharmacokinetic data on its main components, particularly those of their brain distribution and concentrations, and the relationships with blood concentrations exist to elaborate in more detail (Caccia and Gobbi 2009).
Taken together, our results indicate the protective effect of H. perforatum hydroalcoholic extract in a model of PD via attenuation of DNA fragmentation, astrogliosis, inflammation, and oxidative stress that may be of benefit in protective strategies for PD at its early stages.
References
Afshin-Majd S, Khalili M, Roghani M, Mehranmehr N, Baluchnejadmojarad T (2015) Carnosine exerts neuroprotective effect against 6-hydroxydopamine toxicity in hemiparkinsonian rat. Mol Neurobiol 51(3):1064–1070. doi:10.1007/s12035-014-8771-0
Allbutt HN, Henderson JM (2007) Use of the narrow beam test in the rat, 6-hydroxydopamine model of Parkinson’s disease. J Neurosci Methods 159(2):195–202. doi:10.1016/j.jneumeth.2006.07.006
Ara I, Bano S (2009) St. John’s Wort modulates brain regional serotonin metabolism in swim stressed rats. Pak J Pharm Sci 22(1):94–101
Bayramoglu G, Bayramoglu A, Engur S, Senturk H, Ozturk N, Colak S (2013) The hepatoprotective effects of Hypericum perforatum L. on hepatic ischemia/reperfusion injury in rats. Cytotechnology. doi:10.1007/s10616-013-9595-x
Benedi J, Arroyo R, Romero C, Martin-Aragon S, Villar AM (2004) Antioxidant properties and protective effects of a standardized extract of Hypericum perforatum on hydrogen peroxide-induced oxidative damage in PC12 cells. Life Sci 75(10):1263–1276. doi:10.1016/j.lfs.2004.05.001
Biber A, Fischer H, Romer A, Chatterjee SS (1998) Oral bioavailability of hyperforin from hypericum extracts in rats and human volunteers. Pharmacopsychiatry 31:36–43. doi:10.1055/s-2007-979344
Bradford MM (1976) A rapid and sensitive method for the quantitation of microgram quantities of protein utilizing the principle of protein-dye binding. Anal Biochem 72:248–254
Breyer A, Elstner M, Gillessen T, Weiser D, Elstner E (2007) Glutamate-induced cell death in neuronal HT22 cells is attenuated by extracts from St. John’s wort (Hypericum perforatum L.). Phytomedicine 14(4):250–255. doi:10.1016/j.phymed.2007.02.001
Bukhari IA, Dar A (2013) Behavioral profile of Hypericum perforatum (St. John’s Wort) extract. A comparison with standard antidepressants in animal models of depression. Eur Rev Med Pharmacol Sci 17(8):1082–1089
Caccia S, Gobbi M (2009) St. John’s wort components and the brain: uptake, concentrations reached and the mechanisms underlying pharmacological effects. Curr Drug Metab 10(9):1055–1065
Calou I, Bandeira MA, AguiarGalvao W, Cerqueira G, Siqueira R, Neves KR, Brito GA, Viana G (2014) Neuroprotective properties of a standardized extract from Myracrodruon urundeuva Fr. All. (Aroeira-Do-Sertao), as evaluated by a Parkinson’s disease model in rats. Parkinsons Dis. doi:10.1155/2014/519615
Cervo L, Rozio M, Ekalle-Soppo CB, Guiso G, Morazzoni P, Caccia S (2002) Role of hyperforin in the antidepressant-like activity of Hypericum perforatum extracts. Psychopharmacology 164(4):423–428. doi:10.1007/s00213-002-1229-5
Chen S, Le W (2006) Neuroprotective therapy in Parkinson disease. Am J Ther 13(5):445–457. doi:10.1097/01.mjt.0000174353.28012.a7
Claiborne A (1985) Catalase activity. In: Greenwald RA (ed) Handbook of methods for oxygen radical research. CRC, Boca Raton
Cohen GM (1997) Caspases: the executioners of apoptosis. Biochem J 326(Pt 1):1–16
Di Carlo G, Pacilio M, Capasso R, Di Carlo R (2005) Effect on prolactin secretion of Echinacea purpurea, hypericum perforatum and Eleutherococcus senticosus. Phytomedicine 12(9):644–647. doi:10.1016/j.phymed.2004.03.011
Ellman GL (1959) Tissue sulfhydryl groups. Arch Biochem Biophys 82(1):70–77
Fearnley JM, Lees AJ (1991) Ageing and Parkinson’s disease: substantia nigra regional selectivity. Brain 114(Pt 5):2283–2301
Gomez del Rio MA, Sanchez-Reus MI, Iglesias I, Pozo MA, Garcia-Arencibia M, Fernandez-Ruiz J, Garcia-Garcia L, Delgado M, Benedi J (2013) Neuroprotective properties of standardized extracts of Hypericum perforatum on rotenone model of Parkinson’s disease. CNS Neurol Disord Drug Targets 12(5):665–679
Hammer KD, Hillwig ML, Neighbors JD, Sim YJ, Kohut ML, Wiemer DF, Wurtele ES, Birt DF (2008) Pseudohypericin is necessary for the light-activated inhibition of prostaglandin E2 pathways by a 4 component system mimicking an Hypericum perforatum fraction. Phytochemistry 69(12):2354–2362. doi:10.1016/j.phytochem.2008.06.010
Hatano T, Sameshima Y, Kawabata M, Yamada S, Shinozuka K, Nakabayashi T, Mizuno H (2014) St. John’s wort promotes adipocyte differentiation and modulates NF-kappaB activation in 3T3-L1 cells. Biol Pharm Bull 37(7):1132–1138
Healy-Stoffel M, Omar Ahmad S, Stanford JA, Levant B (2014) Differential effects of intrastriatal 6-hydroxydopamine on cell number and morphology in midbrain dopaminergic subregions of the rat. Brain Res 1574:113–119. doi:10.1016/j.brainres.2014.05.045
Hizli D, Hizli F, Kosus A, Yilmaz S, Kosus N, Haltas H, Dede H, Kafali H (2014) Effect of Hypericum perforatum on intraperitoneal adhesion formation in rats. Arch Med Sci 10(2):396–400. doi:10.5114/aoms.2013.33070
Hofrichter J, Krohn M, Schumacher T, Lange C, Feistel B, Walbroel B, Heinze HJ, Crockett S, Sharbel TF, Pahnke J (2013) Reduced Alzheimer’s disease pathology by St. John’s Wort treatment is independent of hyperforin and facilitated by ABCC1 and microglia activation in mice. Curr Alzheimer Res 10(10):1057–1069
Hostanska K, Reichling J, Bommer S, Weber M, Saller R (2003) Hyperforin a constituent of St John’s wort (Hypericum perforatum L.) extract induces apoptosis by triggering activation of caspases and with hypericin synergistically exerts cytotoxicity towards human malignant cell lines. Eur J Pharm Biopharm 56(1):121–132
Husain GM, Chatterjee SS, Singh PN, Kumar V (2011) Beneficial effect of Hypericum perforatum on depression and anxiety in a type 2 diabetic rat model. Acta Pol Pharm 68(6):913–918
Hwang CK, Chun HS (2012) Isoliquiritigenin isolated from licorice Glycyrrhiza uralensis prevents 6-hydroxydopamine-induced apoptosis in dopaminergic neurons. Biosci Biotechnol Biochem 76(3):536–543
Keller JH, Karas M, Muller WE, Volmer DA, Eckert GP, Tawab MA, Blume HH, Dingermann T, Schubert-Zsilavecz M (2003) Determination of hyperforin in mouse brain by high-performance liquid chromatography/tandem mass spectrometry. Anal Chem 75(22):6084–6088. doi:10.1021/ac034520z
Kraus B, Wolff H, Heilmann J, Elstner EF (2007) Influence of Hypericum perforatum extract and its single compounds on amyloid-beta mediated toxicity in microglial cells. Life Sci 81(11):884–894. doi:10.1016/j.lfs.2007.07.020
Kumar A, Garg R, Prakash AK (2010) Effect of St. John’s Wort (Hypericum perforatum) treatment on restraint stress-induced behavioral and biochemical alteration in mice. BMC Complement Altern Med 10:18. doi:10.1186/1472-6882-10-18
Lotharius J, Brundin P (2002) Pathogenesis of Parkinson’s disease: dopamine, vesicles and alpha-synuclein. Nat Rev Neurosci 3(12):932–942. doi:10.1038/nrn983
Miklossy J, Doudet DD, Schwab C, Yu S, McGeer EG, McGeer PL (2006) Role of ICAM-1 in persisting inflammation in Parkinson disease and MPTP monkeys. Exp Neurol 197(2):275–283. doi:10.1016/j.expneurol.2005.10.034
Miller AL (1998) St. John’s Wort (Hypericum perforatum): clinical effects on depression and other conditions. Altern Med Rev 3(1):18–26
Mohanasundari M, Sabesan M (2007) Modulating effect of Hypericum perforatum extract on astrocytes in MPTP induced Parkinson’s disease in mice. Eur Rev Med Pharmacol Sci 11(1):17–20
Mohanasundari M, Srinivasan MS, Sethupathy S, Sabesan M (2006) Enhanced neuroprotective effect by combination of bromocriptine and Hypericum perforatum extract against MPTP-induced neurotoxicity in mice. J Neurol Sci 249(2):140–144. doi:10.1016/j.jns.2006.06.018
Morroni F, Tarozzi A, Sita G, Bolondi C, Zolezzi Moraga JM, Cantelli-Forti G, Hrelia P (2013) Neuroprotective effect of sulforaphane in 6-hydroxydopamine-lesioned mouse model of Parkinson’s disease. Neurotoxicology 36:63–71. doi:10.1016/j.neuro.2013.03.004
Mrozikiewicz PM, Bogacz A, Bartkowiak-Wieczorek J, Kujawski R, Mikolajczak PL, Ozarowski M, Czerny B, Mrozikiewicz-Rakowska B, Grzeskowiak E (2014) Screening for impact of popular herbs improving mental abilities on the transcriptional level of brain transporters. Acta Pharm 64(2):223–232. doi:10.2478/acph-2014-0020
Munoz M, Bermejo-Bescos P, Romero C, Benedi J, Martin-Aragon S (2012) SNP-mediated neuroprotection under glucose deprivation is enhanced by Hypericum perforatum. CNS Neurol Disord Drug Targets 11(2):162–173
Naziroglu M, Cig B, Ozgul C (2014a) Modulation of oxidative stress and Ca(2 +) mobilization through TRPM2 channels in rat dorsal root ganglion neuron by Hypericum perforatum. Neuroscience 263:27–35. doi:10.1016/j.neuroscience.2014.01.006
Naziroglu M, Sahin M, Cig B, Aykur M, Erturan I, Ugan Y (2014b) Hypericum perforatum modulates apoptosis and calcium mobilization through voltage-gated and TRPM2 calcium channels in neutrophil of patients with Behcet’s disease. J Membr Biol 247(3):253–262. doi:10.1007/s00232-014-9630-7
Ott M, Huls M, Cornelius MG, Fricker G (2010) St. John’s Wort constituents modulate P-glycoprotein transport activity at the blood-brain barrier. Pharm Res 27(5):811–822. doi:10.1007/s11095-010-0074-1
Paulke A, Schubert-Zsilavecz M, Wurglics M (2006) Determination of St. John’s wort flavonoid-metabolites in rat brain through high performance liquid chromatography coupled with fluorescence detection. J Chromatogr B Analyt Technol Biomed Life Sci 832(1):109–113. doi:10.1016/j.jchromb.2005.12.043
Paxinos G, Watson C (1986) The rat brain in stereotaxic coordinates, 2nd edn. Academic Press, San Diego
Roghani M, Niknam A, Jalali-Nadoushan MR, Kiasalari Z, Khalili M, Baluchnejadmojarad T (2010) Oral pelargonidin exerts dose-dependent neuroprotection in 6-hydroxydopamine rat model of hemi-parkinsonism. Brain Res Bull 82(5–6):279–283. doi:10.1016/j.brainresbull.2010.06.004
Ruedeberg C, Wiesmann UN, Brattstroem A, Honegger UE (2010) Hypericum perforatum L. (St John’s wort) extract Ze 117 inhibits dopamine re-uptake in rat striatal brain slices. An implication for use in smoking cessation treatment? Phytother Res 24(2):249–251. doi:10.1002/ptr.2921
Sanchez-Reus MI, Gomez del Rio MA, Iglesias I, Elorza M, Slowing K, Benedi J (2007) Standardized Hypericum perforatum reduces oxidative stress and increases gene expression of antioxidant enzymes on rotenone-exposed rats. Neuropharmacology 52(2):606–616. doi:10.1016/j.neuropharm.2006.09.003
Schober A (2004) Classic toxin-induced animal models of Parkinson’s disease: 6-OHDA and MPTP. Cell Tissue Res 318(1):215–224. doi:10.1007/s00441-004-0938-y
Schwarting RK, Huston JP (1997) Behavioral and neurochemical dynamics of neurotoxic meso-striatal dopamine lesions. Neurotoxicology 18(3):689–708
Sedlak J, Lindsay RH (1968) Estimation of total, protein-bound, and nonprotein sulfhydryl groups in tissue with Ellman’s reagent. Anal Biochem 25(1):192–205
Shelton RC (2009) St John’s wort (Hypericum perforatum) in major depression. J Clin Psychiatry 70(Suppl 5):23–27. doi:10.4088/JCP.8157su1c.05
Shinko A, Agari T, Kameda M, Yasuhara T, Kondo A, Tayra JT, Sato K, Sasaki T, Sasada S, Takeuchi H, Wakamori T, Borlongan CV, Date I (2014) Spinal cord stimulation exerts neuroprotective effects against experimental Parkinson’s disease. PLoS One 9(7):e101468. doi:10.1371/journal.pone.0101468
Silva B, Oliveira PJ, Dias A, Malva JO (2008) Quercetin, kaempferol and biapigenin from Hypericum perforatum are neuroprotective against excitotoxic insults. Neurotox Res 13(3–4):265–279
Sriram K, O’Callaghan JP (2007) Divergent roles for tumor necrosis factor-alpha in the brain. J Neuroimmune Pharmacol 2(2):140–153. doi:10.1007/s11481-007-9070-6
Taylor JM, Main BS, Crack PJ (2013) Neuroinflammation and oxidative stress: co-conspirators in the pathology of Parkinson’s disease. Neurochem Int 62(5):803–819. doi:10.1016/j.neuint.2012.12.016
Teismann P, Schulz JB (2004) Cellular pathology of Parkinson’s disease: astrocytes, microglia and inflammation. Cell Tissue Res 318(1):149–161. doi:10.1007/s00441-004-0944-0
und Halbach OVB, Schober A, Krieglstein K (2004) Genes proteins and neurotoxins involved in Parkinson’s disease. Prog Neurobiol 73(3):151–177. doi:10.1016/j.pneurobio.2004.05.002
Walsh S, Finn DP, Dowd E (2011) Time-course of nigrostriatal neurodegeneration and neuroinflammation in the 6-hydroxydopamine-induced axonal and terminal lesion models of Parkinson’s disease in the rat. Neuroscience 175:251–261. doi:10.1016/j.neuroscience.2010.12.005
Yacoubian TA, Standaert DG (2009) Targets for neuroprotection in Parkinson’s disease. Biochim Biophys Acta 1792(7):676–687. doi:10.1016/j.bbadis.2008.09.009
Yoshitake T, Iizuka R, Yoshitake S, Weikop P, Muller WE, Ogren SO, Kehr J (2004) Hypericum perforatum L (St John’s wort) preferentially increases extracellular dopamine levels in the rat prefrontal cortex. Br J Pharmacol 142(3):414–418. doi:10.1038/sj.bjp.0705822
Zare K, Eidi A, Roghani M, Rohani AH (2015) The neuroprotective potential of sinapic acid in the 6-hydroxydopamine-induced hemi-parkinsonian rat. Metab Brain Dis 30(1):205–213. doi:10.1007/s11011-014-9604-6
Zeng KW, Wang XM, Ko H, Kwon HC, Cha JW, Yang HO (2011) Hyperoside protects primary rat cortical neurons from neurotoxicity induced by amyloid beta-protein via the PI3 K/Akt/Bad/Bcl(XL)-regulated mitochondrial apoptotic pathway. Eur J Pharmacol 672(1–3):45–55. doi:10.1016/j.ejphar.2011.09.177
Zhou F, Wu JY, Sun XL, Yao HH, Ding JH, Hu G (2007) Iptakalim alleviates rotenone-induced degeneration of dopaminergic neurons through inhibiting microglia-mediated neuroinflammation. Neuropsychopharmacology 32(12):2570–2580. doi:10.1038/sj.npp.1301381
Acknowledgments
This research study was financially supported by a Grant from Neurophysiology Research Center affiliated to Shahed University (Tehran, Iran) in 2013.
Author information
Authors and Affiliations
Corresponding author
Rights and permissions
About this article
Cite this article
Kiasalari, Z., Baluchnejadmojarad, T. & Roghani, M. Hypericum Perforatum Hydroalcoholic Extract Mitigates Motor Dysfunction and is Neuroprotective in Intrastriatal 6-Hydroxydopamine Rat Model of Parkinson’s Disease. Cell Mol Neurobiol 36, 521–530 (2016). https://doi.org/10.1007/s10571-015-0230-6
Received:
Accepted:
Published:
Issue Date:
DOI: https://doi.org/10.1007/s10571-015-0230-6