Abstract
The formation, stability and in vitro digestion of milk fat globule membrane (MFGM) proteins stabilized emulsions with 0.2 wt% β-carotene were investigated. The average particle size of β-carotene emulsions stabilized with various MFGM proteins levels (1%, 2%, 3%, 4%, 5% wt%) decreased with the increase of MFGM proteins levels. When MFGM proteins concentration in emulsions is above 2%, the average particle size of β-carotene emulsions is below 1.0 μm. A quite stable emulsion was formed at pH 6.0 and 7.0, but particle size increased with decrease in acidity of the β-carotene emulsion. β-carotene emulsions stabilized with MFGM proteins were stable with a certain salt concentrations (0–500 mMNaCl). β-carotene emulsions were quite stable to aggregation of the particles at elevated temperature and time (85 °C for 90 min). At the same time, β-carotene emulsions were stable against degradation under heat treatment conditions. In vitro digestion of β-carotene emulsion showed the mean particle size of β-carotene emulsions stabilized with MFGM proteins in the simulated stomach conditions and intestinal conditions is larger than that of initial emulsions and simulated mouth conditions. Confocal laser scanning microscopy of β-carotene MFGM proteins emulsions also showed the corresponding results to different vitro digestion model. There was a rapid release of free fatty acid (FFA) during the first 10 min and after this period, an almost constant 70% digestion extent was reached. Approximately 80% of β-carotene was released within 2 h of incubation under the simulated intestinal fluid. These results showed that MFGM protein can be used as a good emulsifier in emulsion stabilization, β-carotene rapid release as well as lipophilic bioactive compounds delivery.
Similar content being viewed by others
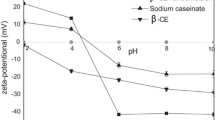
Explore related subjects
Discover the latest articles, news and stories from top researchers in related subjects.Avoid common mistakes on your manuscript.
Introduction
β-carotene as pro-vitamin A is thought to provide protection to the human body, through reducing the risk for some disorders such as cancers, heart disease and colorectal adenomas [1]. In addition, β-carotene plays an important role as a natural colorant and antioxidant in food products [2]. However, the utilization of β-carotene in the food industry is currently limited because of its poor solubility in water and instability in the presence of heat, light, and oxygen. More specifically, β-carotene is readily prone to chemical degradation during both food processing and storage due to chemical, mechanical, and thermal stresses. In recent years, oil-in-water (O/W) emulsions are an important element and widely used in some popular food products such as sauces, dips, desserts, ice cream, beverages, soups, and delivery systems [3]. Oil in-water (O/W) emulsions as delivery systems are used to deliver lipophilic bioactive compounds, such as polyunsaturated fats, oil-soluble vitamins, β-carotene and flavonoids [4]. These compounds have attracted word wide attention because of their low toxicity, anti-oxidative activity, and high bioactivities [5].
Milk proteins, as surface-active molecules, are widely used as food emulsifiers. The ability of milk proteins were used to stabilize emulsions against flocculation and coalescence occurred by a combination of electrostatic and steric interactions [6, 7]. However, MFGM proteins because of the complexity of their molecular structures and compositions can offer unique challenges for being used as emulsifiers to stabilize β-carotene emulsion through homogenization, which expects to improve the stability of emulsions under environmental stresses (thermal processing, freezing, different pH and ionic strength) during their storage and utilization. Similar to other milk proteins (e.g., β-lactoglobulin and caseinate), MFGM proteins also exhibits excellent emulsion properties, which can also be used to stabilize oil-in-fat emulsion and deliver bioactive compounds [8]. MFGM proteins have high bioactivities and interesting physiological functions, such as anticancer properties, prevention of Helicobacter pylori infection and immune function of the brain [9,10,11,12]. There are several beneficial proteins in the MFGM such as mucoprotein1 (MUC1), xanthine dehydrogenase/oxidase (XDH/ XO), periodic acid Schiff III (PAS III), periodic acid Schiff IV (PAS IV), butyrophilin (BTN), periodic acid Schiff 6/7(PAS 6/7), and GTP-binding proteins (GTPrS) [13, 14]. In addition, MFGM proteins have higher molecular weight(48~225 kDa) than β-lactoglobulin and casein, the surface protein coverage (mg/m2) of the MFGM proteins-stabilized emulsions may be higher than β-lactoglobulin and casein stabilized emulsions, because high molecular weight protein has higher surface protein coverage than lowmolecular weight protein, which can improve stabilization of emulsions of MFGM proteins [15].
In a previous study, we found that MFGM proteins have good emulsifying properties, and then try to utilize MFGM proteins to deliver lipophilic bioactive compounds [16]. Therefore, the objective of present study was to investigate formation, stability and in vitro digestion of β-carotene prepared with MFGM proteins, so as to provide a better understanding of MFGM proteins as a vehicle to deliver lipophilic bioactive compounds.
Materials and Methods
Materials
β-carotene, trypsin from porcine pancreas, α-amylase type VI-B from porcine pancreas, mucin type II from porcine stomach, and pepsin from porcine gastric mucosa were purchased from Sigma-Aldrich (Sigma-Aldrich, St. Louis, MO, USA). All other chemicals were used of analytical grade.
Isolation of the MFGM Proteins
The MFGM proteins were isolated from fresh bovine milk. The fresh bovine milk was fractionized using a 9NDS-50A cream separator (Qinghai Kangping, China). The cream was isolated and washed with phosphate buffer (PBS, 0.1 M, pH 6.8), and then centrifuged at 1500×g for 10 min. The supernatant was discarded and the washing step was repeated three times. The washed cream was then used for the isolation MFGM proteins. The separation of the MFGM proteins was based on the procedure reported by Lu et al. [17].
Composition Analysis of the MFGM Proteins
The composition analyses of MFGM proteins were carried out using official AOAC methods, and moisture (14.004), ash (14.006), and crude protein (47.021) were determined [18]. Nitrogen to protein conversion factor of 6.25 was used in the crude protein determination. The lipid content was calculated by subtraction of the percentage of moisture, protein, and ash from the whole mass. All experiments were conducted in triplicates.
Sodium Dodecyl Sulfate Polyacrylamide Gel Electrophoresis (SDS-PAGE) of MFGM Proteins
The MFGM proteins samples were suspended in 0.5 mL of a reducing buffer (6% Tris-0.5 M, 10% glycerol, 5% β-mercaptoethanol, 2% SDS, and 0.05% bromophenol blue). Samples were heated in boiling water for 5 min and then centrifuged at 2500×g for 30 min in order to remove the fat from the samples. The supernatants (10 mL) were loaded onto 12% SDS-polyacrylamide gels. Molecular mass markers ranging from 14.4 to 200 kDa (TransGen Biotech, Co., Ltd., China) and milk proteins migrated at 200 V. Protein bands were stained with a solution of Coomassie Brilliant Blue R-250. Gels were destained with a mix solution of isopropanol, glacial acetic acid and water (1:1:8, v: v: v).
Solution Preparation
MFGM proteins were prepared at various concentrations (1, 2, 3, 4 and 5 wt%) with deionized water. These solutions were stirred 12 h at a speed of approximately 200 rpm to ensure complete dispersion and dissolution. The β-carotene was dissolved in oil at 140 °C by stirring for several seconds, until completely dissolved.
Preparation of β-carotene Emulsions
β-carotene emulsions were prepared using MFGM proteins solutions as the continuous phase and soybean oil containing β-carotene (0.2 wt%) as the dispersed phase at a ratio of 90:10 (w/w). emulsifier solution was mixed by high speed homogenization at 13,000 rpm for 5 min (IKA, Germany). Then the emulsions went through two-stage valve high pressure homogenizer (SPX Flow Technology) at 80 MPa for five cycles at room temperature (25 °C). Sodium azide (0.02 w/v %) was added to final emulsions to prevent microbial growth.
The Size Distribution and Particle Size of β-carotene Emulsions
The size distribution and mean particle size of β-carotene emulsions were determined by laser scattering method using a Malvern Mastersizer 2000 (Malvern Instruments Ltd., Worcestershire, UK). The refractive index of absorption and index of oil phase were 1.45 and 1.33, respectively. The volume weighted mean diameter D4,3 was the parameter used to monitor changes in the droplet size distribution. Each measurement was carried out in triplicate and the mean droplet size D4,3(μm) was calculated using the following equation:
Where ni is the number of particles with the same diameter, and di is the particle size.
Zeta-Potential Measurement
The ζ-potentialof β-carotene emulsion was determined using a Nano-ZS Malvern Instrument (Nano-ZS, Malvern Instruments, Worcestershire, UK). β-carotene emulsions were diluted to a final oil concentration of 0.005 wt% with pH-adjusted deionized water (pH 7.0) prior to zeta potential analysis.
Stability of β-carotene Emulsion
A series of tests was carried out to establish the impact of environmental stresses on the stability of β-carotene emulsions prepared with MFGM proteins. These environmental stresses were selected to cover a range of representative conditions that emulsions may experience in commercial food products:
Emulsion Susceptibility to pH
The pH of β-carotene emulsions were adjusted to various pH levels (2, 4, 6, 8 and 10) with 1 M HCl or NaOH. Following an overnight equilibration period, theparticle size and ζ-potentialof samples were measured to investigate the physical stability of β-carotene emulsions.
Emulsion Susceptibility to Ion Environment
Emulsions were prepared at pH 6.8 and then they were mixed with 0 mL, 10 mL, 20 mL, 30 mL, 40 mL and 50 mL NaCl stock solution (1 M, pH 6.8) to achieve the final volume 100 mL, the emulsion reach NaCl concentration of 0–500 mM at pH 6.8. After equilibrating an overnight, the particle size and ζ-potential of samples were measured to investigate the physical stability of β-carotene emulsions.
Emulsion Susceptibility to Temperature
β-carotene emulsions were heated at 85 °C for 90 min, the samples were taken out at intervals of 15 min. After cooling to room temperature, the particle size distributions of samples were measured. The β-Carotene stability for thermal stresses was analysed to investigate the chemical stability of β-carotene emulsions, the control group samples were solutions without any emulsion.
Analysis of β-carotene Content
The β-carotene content in the emulsions was determined as described by Yuan et al. [19]. The relative β-Carotene content was used for thermal stability analysis and expressed as C/C0, where C0 is the initial concentration of β-carotene C is the concentration of β-carotene at any time during the heat treatment.
Simulated Gastrointestinal Digestion
An in vitro gastrointestinal tract (GIT) model consisting of mouth, gastric and intestinal phases was used to simulate the biological fate of ingested samples. Emulsions were exposed to conditions that simulated those of the mouth, stomach and small intestine, and then were taken out after each phase. This method has been reported in detail previously, and so we only give a brief description here [20,21,22,23].
Initial System
The β-carotene emulsions were placed into a glass beaker in an incubator shaker at 37 °C.
Mouth Phase
A 20 mL of simulated saliva fluid (pH 6.8, 62 mM NaHCO3, 6 mM K2HPO4·3H2O, 15 mMNaCl, 6.4 mM KCl, 3 mM CaCl2, 3 mg/mL mucin type II, 75 U/mL α-amylase type VI-B) was preheated at 37 °C in a temperature-controlled (37 °C) chamber (KEMEG Technology Co., Ltd., Guangdong, Guangdong Province, China), and then mixed with 20 mL-aliquot of initial emulsion, so that the initial mixture contained 1.25% (w/w) oil. The pH of mixture was adjusted to 6.8, and then incubated at 37 °C and stirred at 100 rpm for 10 min by a magnetic stirrer.
Stomach Phase
The sample obtained after the mouth phase was mixed (50:50 volume ratio) with simulated gastric fluid (pH 2.5, 53 mM NaCl, 1 mM CaCl2, 14.8 mM KCl, 5.7 mM Na2CO3, 3.2 mg/mL pepsin). The mixture was then adjusted to pH 2.5 and placed in a shaker at 100 rpm and 37 °C for 2 h to mimic stomach digestion.
Small Intestine Phase
24 mL samples from the stomach phase were diluted with buffer solution (10 mM PBS, 6.5) to obtain a final soybean oil concentration of 1.0%. The diluted sample was then incubated in a water bath (37 °C) for10 min and then the solution was adjusted back to pH 7.0. Next, 3 mL of simulated intestinal fluid (containing 0.5 M CaCl2 and 7.5 M NaCl) was added to 60 mL digestion. Then, 7 mL bile extract, containing 375.0 mg bile extract (pH 7.0, PBS), was added with stirring and the pH was adjusted to 7.0. Finally, 5 mL of lipase suspension, containing 120 mg of lipase (pH 7.0, PBS), was added to the sample and an automatic titration unit (Metrohm, USA Inc.) was used to monitor the pH and control it to a fixed value (pH 7.0) by titrating 0.25 M NaOH solution into the reaction vessel for 2 h at 37 °C. The percentage of free fatty acids released in the sample was calculated from the number of moles of NaOH required to maintain neutral pH as described previously.
Confocal Laser Scanning Microscopy
Confocal laser scanning microscopy (CLSM) experiments were performed by using a Leica TCS SP5 confocal microscope (Leica Microsystems Inc., Heidelberg, Germany). Various emulsions were dyed with fluorescent dye solution (1 mg mL−1 Nile Red in isopropyl alcohol). The dyed emulsions were placed in the hole of a concave slide, and then they were covered with coverslips. Finally, glycerol was coated around the edge of the coverslips to seal the samples. The fluorescent dyes were excited by either an argon laser at 488 nm.
Statistical Analysis
A total 200 g MFGM proteins was prepared from a MFGM isolates, an 800 mL emulsion was prepared for stability analysis and a 400 mL emulsion was prepared for simulated gastrointestinal digestion. Each measurement was carried out in triplicate and average values were calculated. The statistical difference between the mean values was analyzed by the one-factor analysis of the variance, and the least significant difference (LSD) at a significant level set at 0.05. The LSD values were calculated using Duncan’s post hoc test. The calculations were performed using the Statistical Package for the Social Sciences v. 10.0 (SPSS, Chicago, IL, USA).
Results and Discussion
SDS-PAGE of MFGM Proteins
The MFGM proteins isolated from fresh bovine milk contained about 85% protein and 12% lipids. The SDS-PAGE separation of MFGM proteins is shown in Fig. 1. As can be seen, the MFGM proteins were mainly composed of mucin 1(MUC), xanthine oxidase (XO), butyrophilin (BUT), and periodic acid-schiff 6 and 7(PAS6 and PAS7). During the process of MFGM separation, the cream was washed tree times withpH 6.8phosphate buffer solutions, there were small amount of casein and whey proteins were found in the MFGM protein samples (Fig. 1). Study also reported that there was small amount of casein and whey proteins were found in the MFGM protein when separating fat globules were washed three times with deionized water [24]. There were also some reports that MFGM proteins consist of mucin 1(MUC), xanthine oxidase (XO), butyrophilin (BUT), and periodic acid-schiff 6 and 7(PAS6 and PAS7) were found in bovine milk samples [25, 26].
Effects of MFGM Proteins Concentration on the Droplet Size Distribution and Particle Size
Figure 2 illustrates the droplet size distributions and the mean droplet size of MFGM protein-stabilized β-carotene emulsions as a function of MFGM proteins concentration. A similar trend was found in all β-carotene emulsions that the large fat droplet size was shifted to a smaller range along with the increase of MFGM protein levels (Fig. 2a). At low MFGM proteins concentration (1 wt%), the β-carotene emulsion had a wide size distribution, the diversity in particle size contributes to the emulsions had a wide size distribution. The results could be explained by the fact that MFGM proteins at a lower content might insufficient coverage or cross binding to form strong electrostatic repulsion between droplets. Along with the increase of MFGM proteins levels, more MFGM proteins might absorb to the surface of fat droplets and promotes strong electrostatic repulsion between droplets, prevents the fat droplets from flocculating and results in small fat droplets size distributions.
Effects of MFGM protein concentrations (1, 2, 3, 4 and 5 wt%) on the particle distribution (a) and the mean droplet size (b). Samples designated with different letters (a, b) were significantly different (Duncan, p < 0.05) when compared between different emulsions prepared with different MFGM proteins
Figure 2b showed that the mean droplet size of β-carotene emulsions prepared with various MFGM proteins levels. The mean droplet size of β-carotene emulsion prepared with 1 wt% MFGM proteins is about 9.0 μm, which is larger than that of other of β-carotene emulsions prepared with high concentration of MFGM proteins (2-5 wt%). The large droplet size was a clear indication of the flocculation and aggregation of the droplets. Figure 2b also clearly showed the flocculation and aggregation of the droplets and the cream layer separation in β-carotene emulsion prepared with 1 wt% MFGM protein. When the concentration range of MFGM proteins is from 3% to 5%, the mean droplet size of β-carotene emulsions is lower 0.5 μm and no significant changes (p > 0.05) occurred in the mean droplet size, so the 3% MFGM proteins concentration was selected as the next concentration of emulsions preparation. The results may be low MFGM proteins have not the ability to form a thicker protective layer at the interface of oil droplets, promoting electrostatic repulsion between charged droplets which increases emulsion stability to the fat coalescence [27]. The high MFGM proteins level means more proteins will be absorbed to at the oil-water interface and increase emulsion stability.
The Physical-Chemical of Stability
Emulsion stability is important for shelf life, consumer acceptability, and food structure required for pleasant feeling during consumption [28]. The stability of β-carotene emulsions including physical stability and chemical stability, the physical stability refer to the stability of droplets particle, and the chemical stability refer to the stability of degradation of β-carotene. Many factors can influence the stability of emulsions, including pH, temperature and salt concentration. The average droplet size and zeta potential of the β-carotene emulsions as a function of pH values (2.0–10.0) and NaCl concentrations (0–500 mMNaCl) were shown in Fig. 3. Average particle size increased with decrease in acidity of the β-carotene emulsion. This result may be due to the reduction in the net negative charge of fat droplets and resulted in the aggregation of particles at pH values close to the isoelectric point of MFGM proteins. The zeta potential of the β-carotene emulsions presented net positive at pH 2.0 and 4.0 compared to particle with net negative charge at pH from 6.0 to 10.0. This shift in the zeta potential indicates that the surface charge of the prepared emulsion is contributed by milk proteins. β-carotene emulsions prepared with MFGM proteins showed small average particle at pH from 6.0 to 10.0. Reported that emulsions prepared with whey protein showed relatively stable at pH 6.0 and 7.0, but extensive particle growth/aggregation occurred at lower pH values, which was attributed to either chemical (hydrolysis) or physical (electrical charge) effects [29]. The reductions in the electrical charge on the particles at lower pH value would reduce the electrostatic repulsion between them, thereby leading to aggregation [30].
Salts are common food additives and are also present in the human gastrointestinal tract. Salts may therefore affect the functional performance of emulsion delivery systems in a product or after indigestion. For these reasons, the influence of salt concentration on stability of β-carotene emulsions was examined. In the absence of NaCl solution, the zeta-potential of β-carotene emulsions was about −32 mV, indicating that these emulsion droplets had a relatively high negative charge. When the salt concentration of β-carotene emulsions was 500 mMNaCl, the zeta-potential of β-carotene emulsions increased to -8 mV. However, the changes of zeta-potential did not cause significant change (p > 0.05) in the particle size (Fig. 3b). The zeta potential of β-carotene emulsions shifted toward zero due to the decrease in the electrostatic repulsion between the particles upon addition of salt. The results indicated that β-carotene emulsions stabilized with MFGM protein was stable with a certain salt concentrations (0–500 mMNaCl). Although the electrostatic repulsion is still sufficiently strong to overcome the weak van derWaals and hydrophobic attraction at relatively low salt concentration, it is no longer strong enough so that the attractive forces dominate, leading to droplet aggregation above a critical salt level [30].
The influence of heat treatment on the droplets size distribution of the β-carotene emulsions was evaluated at 85 °C for 90 min and results are presented in Fig. 4a. The droplets size distribution of the β-carotene emulsions presented coincident trend at different heat treatmenttime. The results indicated that MFGM proteins-stabilized β-carotene emulsions were stable to aggregation of the particles at elevated temperature and time. Although the heat treatment temperature is above 65 °C can result in the denaturation of MFGM proteins, the fat droplet surface of β-carotene emulsions absorbed high concentration of MFGM protein can prevent the fat from aggregating [31]. The stability of β-Carotene refer to the β-Carotene was protected from degradation under certain conditions. The stability of β-Carotene can be determined by the content changes of β-Carotene,the relative β-carotene content in MFGM proteins stabilized-emulsions and control samples was investigated (Fig. 4b). The degradation of β-carotene in MFGM proteins stabilized-emulsions was slower than that of control samples. Control samplesshowed that the relative β-carotene content decreases by about 80% after 90 min heat treatment, however, the relative β-carotene content in MFGM proteins stabilized-emulsions decreases by only about 20%. The results indicated that β-carotene in MFGM proteins stabilized-emulsions were stable against degradation under heat treatment conditions.
The effects of heat treatment at 85 °C (0, 15, 30, 45, 60, 75 and 90 min) on the particle size distribution (a) and the relative β-carotenecontent of emulsions (b). Samples designated with different letters (a, b, c, d, e) were significantly different (Duncan, p < 0.05) when compared between the same emulsions were heated at 90°Cfor different time
In Vitro Digestion of β-carotene Emulsion
There was evidence for the fact that the carotenoid bioaccessibility was affected by the particle size of the complex food matrix [32]. Particle size measurements were conducted to clarify the emulsion particle changes during each digestive process. As can be seen in Fig. 5, significant changes in the particle size distribution occurred in different vitro digestion stages, a bigger fat droplet distribution range of β-carotene emulsions showed in the simulated stomach conditions and intestinal conditions than initial emulsion and the simulated mouth conditions (Fig. 5a). The mean particle size of β-carotene emulsions stabilized with MFGM proteins is also bigger in the simulated stomach conditions and intestinal conditions than that of initial emulsion and simulated mouth conditions (Fig. 5b). Significant changes in the particle size distribution and mean particle size in the different simulated vitro digestion conditions may be explained by zeta potential of emulsion. After exposure to the simulated mouth phase, the negative charge on the lipid droplets was reduced but no significant changes (p > 0.05) occurred compare to initial emulsion (Fig. 5c). There are no significant changes (p > 0.05) in mean particle size in the simulated mouth phase. The magnitude of the negative charge on the lipid droplets decreased drastically after exposure to the simulated stomach conditions. Otherwise, under acid condition, H+ combination with COO− become neutral (COOH) and NH2 become positively charged (NH3+) and resulted in positive charged of lipid droplets in the simulated stomach conditions. It indicated that the change in the zeta-potential from negative values to positive values was due to a change in pH to below the isoelectric point of MFGM proteins. There were no adequate electrostatic repulsion and steric hindrance required for emulsion stability in the simulated stomach conditions and lead to droplet aggregation and flocculation occurred in the simulated stomach conditions. Confocal microscopy images also indicated that some lipid droplets flocculation had occurred in the simulated stomach conditions (Fig. 6). Otherwise, another fact may be due to the pepsin hydrolysed MFGM proteins and produced more small peptides, they were absorbed to the surface of fat droplets and enable β-carotene emulsions to unstable than before. There was report that the mean particle size of β-carotene emulsions stabilized with β-lactoglobulin and sodium caseinate increased after the pepsin hydrolysis in the simulated stomach conditions [33]. These findings could be possibly explained by the fact the electrostatic repulsive and steric barriers of the peptides remaining at the interface were not sufficiently strong to overcome the attractive forces between droplets. Therefore, extensive flocculation followed by coalescence of the droplets occurred. Otherwise, the presence of salts in the gastric juices also promotes droplet flocculation due to electrostatic screening effects [34].
The change in particle size distribution (a), average droplet size (b) and ζ-potential (c) of the β-carotene emulsions prepared with MFGM proteins in digestion model. Values are expressed as means ± S.D. (n = 3). Samples designated with different letters (a, b, c) were significantly different (Duncan, p < 0.05) when compared between different digestion stages (same emulsion)
Particle size distributions and average particle size were analysed after the incubation under the simulated intestinal conditions, the changes in the peaks during digestion in the simulated intestinal conditions were broader than in the simulated mouth conditions, and initial emulsion (Fig. 5a). The mean particle size of fat droplets stabilized with MFGM proteins in the simulated intestinal conditions are also bigger than that of initial emulsion and simulated mouth conditions (Fig. 5b). These results suggested that coalescence, which was promoted by pancreatin, occurred in the emulsions. The increase of mean particle in the simulated small intestinal fluids were largely attributed to the enzymolysis of the hydrophobic lipids and the proteolysis of the adsorbed proteins, as well as the interactions among the milk proteins, bile salts, phospholipids and fatty acids. Bile salts in simulated intestinal fluid may involve in the process of destabilization, as these surface-active compounds displace the interfacial proteins/peptides from the surfaces of emulsion droplets, which facilitate the pancreatin hydrolyse by accessing to the emulsified lipid core. In addition, more and more fatty acids and monoacylglycerols are released from the core of the droplets during lipid digestion. Fatty acids and monoacylglycerols are surface-active substances and accumulate at the surfaces of emulsion droplets [3]. These emulsion droplets with new surfaces consisting of small peptides, fatty acids and monoacylglycerols will be more susceptible to coalescence, because of their lower surface viscosity and elasticity [35]. The microstructures of emulsions as examined using confocal laser scanning microscopy confirmed the size distribution results (Fig. 6). It was apparent that there was some very large oil droplets in the samples that were digested for 120 min, the number of small droplets reduced during the 2 h of intestinal digestion time. These were probably because the small droplets have large surface area and increase the absorption of pancreatic lipase and were digested for a long time (120 min). The increase in the size of the emulsion droplets during digestion in the simulated intestinal conditions indicated coalescence of oil droplets occurred in intestinal digestion stage, which matched with the broad particle size distribution in those samples.
Release of Free Fatty Acid and β-carotene
The digestion of bioactives in the gastrointestinal tract is a complex process and its impact on the release of bioactive component plays a major role in the uptake, distribution as well as bioavailability of the component. Free fatty acid and β-carotene digestion extent of emulsions during in vitro simulated intestinal fluid digestion were shown in Fig. 7.There was a rapid release of FFA during the first 10 min and after this period, an almost constant value was reached. Similar results were also observed on the digestion of β-lactoglobulin, sodium caseinate, lactalbumin and lactoferrin emulsions under the same intestinal conditions [33]. As reported in the literature, when working at a high lipase concentration (100 units g−1), the adsorption of lipase at the o/w interface occursrapidly and consequently, the digestion process begins almostimmediately after the lipase incorporation to the reaction vessel [36]. The slower rate of FFA release after 10 min of the digestion process could be associated with the release of FFA molecules that have interfacial activity so they could compete with the lipase molecules in order to get adsorbed at the interface and reduce the lipase activity [37, 38].
There was a rapid release of β-carotene during the first 10 min and after this period, an almost constant value was reached. This result could be explained the fact that the pancreatic lipases in the small intestine would rapidly adsorb onto the droplet surface, usually via complexion with bile salts during the first 10 min, thus increasing the accessibility of the lipase to the hydrophobic lipid region and resulted in a rapid release of β-carotene [39]. The incubation with simulated intestinal fluid resulted in destabilization of the emulsion and approximately 80% of β-carotene was released within 2 h of incubation (Fig. 7). This indicates that the prepared β-carotene emulsions can be destabilized with subsequent release of β-carotene in presence of pancreatin. Liu et al. studied the effects of milk proteins on release properties of β-carotene emulsions during in vitro digestion and reported that the β-carotene digestion extent in the emulsions stabilized with β-lactoglobulin, sodium caseinate, lactalbumin and lactoferrin were 92%, 84%, 81%, and83%, respectively [33]. These phenomena implied that targeted release of β-carotene could be found in the small intestine, depending on the interfacial components and digestive fluids.
Conclusions
Emulsions with 0.2 wt% β-carotene can be stabilized when MFGM proteins concentration was above 2 wt%; a relatively stable emulsion was formed at pH 6.0 and 7.0. β-carotene emulsions were physical stable to a certain sat concentration (0–500 mMNaCl) and heat treatment temperature and time (85 °C for 90 min). In vitro digestion indicated that the β-carotene emulsion stabilized with MFGM proteins exhibited the good release tendency in both gastric and intestinal fluids with distinct changes in the particle size. Most FFA and β-carotene release occurred in the stage that lipase was included. Overall, this study shows that MFGM proteins can be used to protect lipophilic bioactive compounds from chemical degradation during food process and promote their utilization as a functional ingredient in food products.
References
M.K. Das, P.K. Sahu, G.S. Rao, K. Mukkanti, L. Silpavathi, J. Saudi, Biol Sci 21(6), 539–546 (2014)
Z.Q. Hou, M. Zhang, B. Liu, Q.L. Yan, F. Yuan, D.X. Xu, Y.X. Gao, Food Hydrocoll. 26(1), 205–211 (2012)
D.J. McClements, Annu. Rev. Food Sci. Technol. 1(1), 241–269 (2010)
D.J. McClements, E.A. Decker, Y. Park, Crit. Rev. Food Sci. 49(1), 48–67 (2009)
M.A. Das Neves, I. Kobayashi, M. Nakajima, J. Food Drug Anal. 20, 184–188 (2012)
D. Guzey, D.J. McClements, Adv. Colloid Interfac 128-130, 227–248 (2006)
D.J. McClements, Curr. Opin. Colloid In 9(5), 305–313 (2004)
T.T.Q. Phan, M. Asaduzzaman, T.T. Le, E. Fredrick, P. Van der Meeren, K. Dewettinck, Int. Dairy J. 29(2), 99–106 (2013)
V.L. Spitsberg, J. Dairy Sci. 88(7), 2289–2294 (2005)
S. Gallier, X.Q. Zhu, S.M. Rutherfurd, A. Ye, P.J. Moughan, H. Singh, Food Chem. 141(3), 3215–3223 (2013)
O. Ito, S. Kamata, M. Hayashi, K. Ushiyama, J. Food Sci. 58(4), 753–755 (1993)
H.M. Martin, J.T. Hancock, V. Salisbury, R. Harrison, Infect. Immun. 72(9), 4933–4939 (2004)
K. Dewettinck, R. Rombaut, N. Thienpont, T.T. Le, K. Messens, J.V. Camp, Int. Dairy J. 18(5), 436–457 (2008)
V.L. Spitsberg, E. Matitashvili, R.C. Gorewit, Eur. J. Biochem. 230(3), 872–878 (1995)
A. Ye, H. Singh, J Colloid Interf Sci 295(1), 249–254 (2006)
S.H. He, H.S. Tang, H.X. Yi, W.L. Xu, Y. Ma, R.C. Wang, Int. J. Food Prop. 20(S2), S1342–S1353 (2017)
J. Lu, X.Y. Wang, W.Q. Zhang, L. Liu, X.Y. Pang, S.W. Zhang, J.P. Lv, Food Chem. 196, 665–672 (2016)
AOAC. Official methods of analysis, in 15th Edn.; Washington DC. Association of official analytical chemists (1990)
Y. Yuan, Y. Gao, J. Zhao, L. Mao, Food Res. Int. 41(1), 61–68 (2008)
L. Salvia-Trujillo, C. Qian, O. Martin-Belloso, D.J. McClements, Food Chem. 141(3), 1472–1480 (2013)
L. Salvia-Trujillo, C. Qian, O. Martin-Belloso, D.J. McClements, Food Chem. 139(1–4), 878–884 (2013)
Y.G. Chang, D.J. McClements, Food Hydrocoll. 61, 92–101 (2016)
M. Espinal-Ruiz, F. Parada-Alfonso, L.P. Restrepo-Sanchez, C.E. Narvaez-Cuenca, D.J. McClements, Food Funct. 5(12), 3083–3095 (2014)
T.T. Le, J. Van Camp, R. Rombaut, F. Van Leeckwyck, K. Dewettinck, J. Dairy Sci. 92(8), 3592–3603 (2009)
A. Ye, H. Singh, M.W. Taylor, S. Anema, Int. Dairy J. 12(4), 393–402 (2002)
K. Dewettinck, R. Rombaut, N. Thienpont, T.T. Le, K. Messens, J.V. Camp, Int. Dairy J. 18(5), 436–457 (2008)
D.X. Xu, Y.M. Qi, X. Wang, X. Li, S.J. Wang, Y.P. Cao, C.T. Wang, B.G. Sun, E. Decker, A. Panya, Food Funct. 8(1), 415–423 (2017)
E.A. Ercelebi, E. Ibanoglu, Int. J. Food Prop. 13(3), 618–630 (2010)
R.A. Mantovani, Â.L. Cavallieri, F.M. Netto, R.L. Cunha, Food Funct. 4(9), 1322–1331 (2013)
D.J. McClements, Food Emulsions: Principles, Practice, And Techniques (CRC Press, Boca Raton, FL, 2005)
A. Ye, H. Singh, M.W. Taylor, S. Anema, Lait 84(3), 269–283 (2004)
K.R.N. Moelants, L. Lemmens, M. Vandebroeck, S. Van Buggenhout, A.M. Van Loey, M.E. Hendrickx, J. Agric. Food Chem. 60(48), 11995–12003 (2012)
Y.W. Liu, F. Lei, F. Yuan, Y.X. Gao, Food Funct. 5(11), 2940–2947 (2014)
A. Sarkar, D.K.K.T. Goh, R.P. Singh, H. Singh, Food Hydrocoll. 23(6), 1563–1569 (2009)
J.E. Staggers, O. Hernell, R.J. Stafford, M.C. Carey, Biochemistry 29(8), 2028–2040 (1990)
S. Mun, E.A. Decker, D.J. McClements, Food Res. Int. 40(6), 770–781 (2007)
P. Reis, K. Holmberg, H. Watzke, M.E. Leser, R. Miller, Adv. Colloid Interfac 147-148, 237–250 (2009)
E. Troncoso, J.M. Aguilera, D.J. McClements, Food Hydrocoll. 27(2), 355–363 (2012)
A. Sarkar, D.S. Horne, H. Singh, Food Hydrocoll. 24(2–3), 142–151 (2010)
Acknowledgements
This work was supported by China Postdoctoral Science Foundation Grant (2012 M520756; 2014 T70360), from Chinese Postdoctoral Science Foundation Commission.
Author information
Authors and Affiliations
Corresponding author
Ethics declarations
Conflict of Interest
We have no conflict of interest in this research.
Rights and permissions
About this article
Cite this article
Li, Q., He, S., Xu, W. et al. Formation, Stability and In Vitro Digestion of β-carotene in Oil-in-Water Milk Fat Globule Membrane Protein Emulsions. Food Biophysics 13, 198–207 (2018). https://doi.org/10.1007/s11483-018-9525-8
Received:
Accepted:
Published:
Issue Date:
DOI: https://doi.org/10.1007/s11483-018-9525-8