Abstract
The addition of ectomycorrhizal fungi (ECMF), beneficial rhizosphere microorganisms, to the soil can promote plant growth and resistance. Here, Populus davidiana × Populus bolleana tissue culture seedlings were grown for 3 months in soils inoculated with one of the species, then seedlings were assessed for mycorrhizal colonization rate and growth, physiological and root traits. Suillus luteus and Populus involutus each formed ectomycorrhizal associations with the seedlings. Seedling height, ground diameter, biomass, and leaf area were significantly greater after treatment with ECMF than in the non-inoculated controls. Treatment improved all physiological and root variables assessed (chlorophylls and carotenoids, cellulose, and soluble sugars and proteins; root length, surface area, projected area, mean diameter, volume, number of root tips). Seedlings inoculated with S. luteus outperformed those inoculated with P. involutus.
Similar content being viewed by others
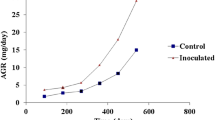
Avoid common mistakes on your manuscript.
Introduction
The Populus davidiana × Populus bolleana is noteworthy for its adaptability, short growth cycle, and superior wood and thus widely planted for its timber and in forests worldwide (Huang et al. 2012; Nasir and Tanweer 2014). It is important in ecological and environmental restorations and included in afforestations over large portions of arid and semiarid areas in northern China. However, poplar plantation forests can experience several problems including poor tree growth, extensive dieback, and frequent pest and disease outbreaks, which can significantly impact ecological functions and economic losses (Liu et al 2020). Therefore, high-quality poplar plantation forests are critical for the future of forestry production, and high-quality seedlings are critical to the success of afforestation projects (Yin et al 2015). One of the best methods to improve seedling and soil quality of afforestation sites is by introducing beneficial microorganisms such as ectomycorrhizal fungi (ECMF) into the rhizosphere, thus promoting seedling growth, increasing their resistance, and preventing disease (Podila et al 2009; Richardson et al 2009; Scheublin et al 2010; Chen et al 2019; de Vries et al 2020).
Poplar plants are colonized ectomycorrhizal fungi, primarily species belonging to Basidiomycotina, but also to Ascomycotina (Tang et al 2008). ECMF form a mutualistic symbiosis with roots (Genre et al 2020) and absorb inorganic nutrients such as nitrogen and phosphorus from the surrounding soil and deliver them to host plants via extraradical mycelia (Smith et al 2009; Das et al 2022). This dense mycelial network also increases the absorption area of the host plant root system and its ability to absorb water and various mineral nutrients (Liu 2021), thereby promoting plant growth and physiological and biochemical processes (Song et al 2007; Rooney et al 2009; Gong et al 2013; Yang et al 2014; Zhang et al 2020; Wang et al 2021a). Such mycorrhizal partnerships are common in poplars (Wang et al 2021b), and ECMF colonization can benefit poplar seedlings, laying the groundwork for good growth later in the season (Quoreshi and Khasa 2008). Thus, the introduction of seedlings with established mycorrhiza during afforestation could significantly improve their survival and quality.
In previous studies of Pinus sylvestris var. mongolica, S. luteus, introduced as a suspension into the soil of potted seedlings, established an ectomycorrhizal symbiosis with the seedlings and significantly enhanced their growth (Yin et al 2014; Wang et al 2021c) and improved their resistance to drought and tolerance to salt and alkalinity (Yin et al 2018, 2020). This growth promotion is significantly higher when external nutrients and probiotics are applied (Yin et al 2021). However, the impact of ectomycorrhizal fungi on the roots and the underlying mechanisms for the growth promotion are unknown.
To promote seedling establishment and survival of Populus davidiana × P. bolleana seedlings, two ectomycorrhizal fungi, S. luteus and P. involutus, were added to soils. We expected that seedling height, ground diameter, and root growth would be greater than for seedlings without the fungi. We also expected that physicochemical properties would be enhanced in the seedlings, yielding high-quality mycorrhizal seedlings that are more resilient to adverse conditions during afforestation. This work should also provide a reliable theoretical basis for developing the best protocol for growing the highest quality poplar seedlings for afforestation programs.
Materials and methods
Plant and fungal sources, site conditions
Populus davidiana × P. bolleana seedlings (1 year old) were generated by tissue culture. Suillus luteus and Paxillus involutus were isolated from a forest at the Zhanggutai Experimental Forest Farm, Changwu County, Liaoning Province. The region has a temperate continental monsoon climate, with an average annual growth period of 140 days and an average annual frost-free period of 155 days, with a maximum of 158 days and a minimum of 150 days, and mean annual sunshine of 3000 h and mean annual precipitation of 450 mm.
Material preparation
For growing seedlings, grass charcoal soil and vermiculite were mixed at a 2:1 ratio and sterilized in bags for 2 h at 120 °C in an autoclave, then placed in pots (diameter: 20 cm, height: 14 cm) and set aside.
For inoculum, Suillus luteus and Paxillus involutus were grown on potato dextrose (PDA) agar (20 g glucose, 200 g potato, 20 g agar, 3 g KH2PO4, 1.5 g MgSO4, 1.5 g peptone, and 1000 mL distilled water) approximately 1 month before seedling transplantation (Yin et al 2020).
Just before the cultures were used as inoculum, three 1-cm-diameter mycelial blocks from one of the fungal cultures on PDA were placed in 500 mL conical flask containing 200 mL of PD broth (PDB) and incubated on a shaker (25 °C, 170 rpm) for 24 h, then homogenized in a disintegrator and mixed 1:3 with water for use (Yin et al 2021).
Experimental design
The seedlings were inoculated using the perforated root irrigation method. Holes were made in the rhizosphere soil, then 100 mL of either a fungal solution (either Suillus luteus [Sl, Treatment 1]) or Paxillus involutus [Pi, Treatment 2]) or sterilized broth (Treatment 3: control check [CK]) was poured into the holes. Each treatment was replicated five times, and each pot was inoculated with 100 mL of fungal suspension and 15 seedlings. The seedlings were then grown for 2 months.
Measurement of relevant indicators
Mycorrhizal colonization rate
After 2 months of growth, three random seedlings from each treatment were removed from the pots to examine their mycorrhizal morphology using a stereoscope. A statistical sampling method was used to count the number of colonized root segments and calculate the rate of colonization as Mycorrhizal colonization rate (%) = (Number of root segments colonized by mycorrhizae/Total number of root segments) × 100.
Growth variables
Three other seedlings from each treatment were randomly removed from the soil, and the roots of cleaned of any attached soil using running tap water. Seedling height and ground diameter were measured using a tape measure and a Vernier caliper, respectively. Three leaves were chosen at random from the base up to the 10th leaf for each seedling, and the leaf area was calculated using the square grid method (Gao 2006). On standard calculation paper (minimum square size: 1 mm × 1 mm), the leaf contour was traced, and the squares within the leaf contour were counted (Squares that reached or exceeded the half lattice were counted as 1, and those that were less than half lattice were not counted.). The area of the three detached leaves from each seedling was measured using the square grid method and the average calculated. Each seedling was separated into shoots and roots, which were weighed with an electronic balance to determine the above- and belowground fresh mass and weighed again after they were dried to a constant mass at 80 °C to determine the dry mass.
Quantification of chlorophyll content
Total chlorophylls were extracted from 0.5 g of leaves using the method of Gao (2006) by grinding them with a mortar and pestle in 0.5 mL of acetone with a small amount of CaCO3 and quartz sand until homogeneous. The mixture and 10 mL of 80% acetone to rinse out the mortar was filtered through Whatman no. 1 filter paper. The filtrate was poured into a 25 mL volumetric flask and the volume to the scale line with 80% acetone. Absorption of each filtrate for each treatment was measured at 470 nm, 645 nm, and 663 nm using a NanoDrop Spectrophotomter (Thermo Fisher Scientific, Waltham, MA, USA) with 80% acetone as a blank. Three replicates were analyzed for each treatment.
Soluble proteins
The method of Gao (2006) was used. Three leaves from different seedlings (0.2 g) were ground in distilled water with a bit of quartz sand using a mortar and pestle, then transferred to a 10-mL volumetric bottle with 2–3 rinses of the mortar and pestle with distilled water, and volume brought to scale with distilled water. After 2–3 mL of the homogenate was centrifuged at 5000 × g for 10 min, 0.1 mL of the supernatant containing the soluble proteins was mixed with 0.9 mL of distilled water and 5 mL of Coomassie bright blue G250. After 2 min, absorbance of the solution at 595 nm was measured using the NanoDrop Spectrophotometer, and total proteins determined using an albumin standard curve (10 mL).
Soluble sugars and cellulose
The method of Gao (2006) based on anthrone was used to measure soluble sugars. The stem from one seedling was crushed, filtered through a 100 mesh screen, then dried to a constant mass at 80 °C, then 0.1 g was placed in a large test tube with 10 mL of distilled water. The mixture was heated for 20 min in a boiling water bath, cooled, and filtered through a Whatman no. 1 filter into a 100-mL volumetric bottle. The residue on the filter was rinsed several times with a constant volume of distilled water, then the sample solution was mixed well, and 1.0 mL was combined with 5 mL of anthrone and boiled for 10 min and allowed to cool. Absorbance was measured at 620 nm using the NanoDrop Spectrophotometer. Soluble sugars were quantified using a standard curve generated using soluble sugars.
For cellulose content, the method of Niu (1992) using anthrone was used. The dried stem of a seedling was ground to a powder as described for soluble sugars and 0.1 g was filtered through 100-mesh screen, then mixed with 60 mL of 60% concentrated sulfuric acid and held for 30 min in an ice bath. The digested cellulose mixture was transferred to a 100-mL volumetric flask to be scaled with 60% v/v sulfuric acid. The solution was mixed, filtered through a Buchner funnel, and 5 mL of the sample was added to a 100-mL volumetric flask in an ice water bath and the volume brought to the 100-mL mark with distilled water; 2 mL of this solution was added to a test tube with 0.5 mL of 2% anthrone reagent and 5 mL of concentrated sulfuric acid, the tube was capped and the solution mixed thoroughly and placed on ice for approximately 12 min. Absorbance of the sample was measured at 620 nm. Cellulose content was quantified using a standard curve generated using cellulose.
Root variables
A scanner was used to scan and grade the root system of each plant. The scanned images were analyzed for root parameters, which included the length, surface area, projected area, volume, mean diameter, and number of root tips, using the image analysis system Win/Mac RHIZO (Regent Instruments, Quebec City, Quebec, Canada).
Data analyses
The collected data were entered into Excel 2018 (Microsoft, Redmond, WA, USA). Means of measured variables were compared for significant differences (α = 0.05) among treatments using a one-way analysis of variance using SPSS 26.0 (IBM, Armonk, NY, USA). Data were plotted using Prism 8.0 (GraphPad, Boston, MA, USA).
Results
Mycorrhizal colonization rate and morphological observation
After 2 months, both fungal species formed ectomycorrhizal roots on Populus davidiana × P. bolleana, at a colonization rate of 64.83% for P. involutus and 68.08% for S. luteus (Fig. 1a). Light microscopy revealed that P. involutus had a darker color, a rod-like and bifurcated shape, and the root tips lacked root hairs. On the other hand, the mycorrhizal roots formed by S. luteus were rod-shaped, whereas the front end of the root system that formed mycorrhizal roots was shorter and thicker, with an enlarged shape (Figs. 1b, c). The root system of the control group was smooth and slender due to the absence of mycorrhiza formation (Fig. 1d).
Mycorrhizal colonization rate on Populus davidiana × P. bolleana after 2 months of growth in soil inoculated with Suillus luteus (Sl) or Paxillus involutus (Pi) and light micrographs of mycorrhizal morphology. a Mean (± SD) colonization rates. The same letters indicate means did not differ significantly (ANOVA, P < 0.05). b, c Mycorrhizal morphology of (b) P. involutus and (c) S. luteus. d Root morphology of control in soil without any fungi. Scale bars = 2 mm
Effect of ECMF on seedling growth
The seedlings inoculated with either of the fungal species grew more vigorously than the uninoculated controls, with significantly greater increases in seedling height, ground diameter, and biomass (Figs. 2, 3) and S. luteus-inoculated seedling performing significantly better than those inoculated with P. involutus (P < 0.05). After 2 months, seedling height was 17.70% greater (Fig. 2a) and ground diameter 10.21% greater (Fig. 2b) after inoculation with S. luteus compared with seedlings inoculated with P. involutus. Compared with fresh mass of controls, fresh mass of seedlings inoculated with S. luteus was 12.66% greater and 37.5% greater for those with P. involutus (Fig. 2c), whereas dry mass was 27.22% and 55.26% greater, respectively (Fig. 2d).
Growth traits of Populus davidiana × P. bolleana seedlings after 2 months of growth in uninoculated soil (CK) or soil inoculated with either Paxillus involutus (Pi) or Suillus luteus (Sl). Mean ± SD a height; b ground diameter; above- and belowground c fresh and d dry mass. Significant differences between means in (a) and (b) are indicated by different lowercase letters and by different capital letters for aboveground mass and different lowercase letters for above ground mass in (c) and (d) (ANOVA, P < 0.05)
Leaf area of the seedlings in both inoculation treatments was significantly greater than that of the control group (Fig. 4; P < 0.05) and compared with the controls, was 60.95% and 82.46% greater after inoculation with P. involutus and S. luteus, respectively (Fig. 4a). Leaf area after colonization with S. luteus was 13.37% greater than after colonization with P. involutus (Fig. 4a).
Leaf area of Populus davidiana × P. bolleana seedlings after 2 months of growth in uninoculated soil (CK) or soil inoculated with either Paxillus involutus (Pi) or Suillus luteus (Sl). a Mean (± SD) leaf area determined using the square grid method. Different lowercase letters indicate significant differences between means (ANOVA, N = 3 leaves per plant per treatment, P < 0.05). b Leaf traces on grip paper to determine leaf areas
Effect of ECMF on chlorophylls and carotenoids in seedlings
After 2 months, the two fungal species significantly increased the contents of chlorophyll a and b and carotenoids in the leaves compared with the controls (Fig. 5, ANOVA, P < 0.05). Only the chlorophyll a differed significantly between the seedlings inoculated with the different fungal species (P < 0.05; 3.52%, 1.74%, and 0.95% higher, respectively, with S. luteus than with P. involutus). Compared to levels in the control treatment, chlorophyll a, chlorophyll b, and carotenoids in seedlings with S. luteus was 25.81%, and 30.86% higher, respectively, and 8.40%, 23.66%, and 29.63% higher, respectively, in those with P. involutus.
Effect of ECMF on physiological indicators in Populus davidiana × P. bolleana seedlings after 2 months of growth in uninoculated soil (CK) or soil inoculated with either Paxillus involutus (Pi) or Suillus luteus (Sl). Mean (± SD) content of a chlorophyll a, b chlorophyll b, c carotenoids, d soluble proteins, e soluble sugars, f cellulose. Different lowercase letters indicate a significant difference between means (ANOVA, P < 0.05)
Effects of ECMF on soluble proteins, soluble sugars, and cellulose in seedlings
In contrast to levels in the control treatment after 2 months, soluble proteins, soluble sugars, and cellulose content were significantly higher in the inoculated seedlings (respectively: with S. luteus, 43.96%, 56.47%, and 10.44% higher; with P. involutus, 20.73%, 13.14%, and 8.14%). Comparing levels of inoculation with the two fungal species, the soluble proteins and soluble sugars were significantly higher with S. luteus; cellulose did not differ (respectively, 19.23%, 38.29%, and 2.13% higher with S. luteus).
Effect of ECMF on root variables of seedlings
Inoculation with either fungus significantly increased all root variables compared with the controls after 2 months (Fig. 6; ANOVA, P < 0.05). All root variables, except diameter, were significantly better in seedlings inoculated with S. luteus than with P. involutus. In comparison to the variables in the control, the root lengths with S. luteus and P. involutus were 122.79% and 67.64% longer, respectively (Fig. 6a), root surface area was 165.99 and 103.94% greater (Fig. 6b), root projected area was 157.70% and 100.12% greater (Fig. 6c), mean diameter of the roots was 16.40% and 15.31% larger (Fig. 6d), and root volume was 227.00% and 131.64% greater (Fig. 6e), and the number of root tips was 105.67% and 77.76% greater (Fig. 6f). Compared with the effects with P. involutus, S. luteus increased root length 32.90% more (Fig. 6a), root surface area 30.42% more (Fig. 6b), projected root area 28.75% more (Fig. 6c), root diameter only 0.39% more (Fig. 6d), root volume 41.16% more (Fig. 6e), number of root tips 15.70% more (Fig. 6f).
Effect of ECMF on the root parameters of Populus davidiana × P. bolleana after 2 months of growth in uninoculated soil (CK) or soil inoculated with either Paxillus involutus (Pi) or Suillus luteus (Sl). Mean (± SD) root a length, b surface area, c projected area, d diameter, e volume, and f number of root tips. Different lowercase letters indicate significant differences between means (ANOVA, P < 0.05)
Thus, compared to control seedlings, seedlings inoculated with S. luteus or P. involutus had more root tissue and better root systems in terms of area, number of bifurcations, diameter, and length. S. luteus and P. involutus both promoted seedling root growth, but S. luteus had a more pronounced effect (Fig. 7).
Discussion
In the present study of Populus davidiana × P. bolleana seedlings, inoculation with Suillus luteus or with Paxillus involutus significantly increased all growth variables for the shoots and roots and physiological variables that were assessed. Similarly in Prunus cerasifera seedlings (Berta et al 1995) and Elaeagnus angustifolia seedlings (Xu et al 2021), ECMF inoculation significantly increases leaf area compared with that of uninoculated seedlings.
Because chlorophylls are the major light-capturing pigment, chlorophyll content is an important indicator of photosynthetic efficiency (Zheng et al 2008). ECMF are known to increase chlorophyll content in host plants (Moran et al 1994; Mathur and Vyas 2000; Sheng et al 2008; Evelin et al 2009; Zai et al 2012; Gong et al 2013), so not surprisingly, we found that significantly higher chlorophyll levels in the ECMF-treated seedlings than in the uninoculated control, likely improving photosynthetic capacity, as do the larger leaves blades as shown for ectomycorrhizal Zea mays.
Like our present study, several studies have shown that inoculating host plants with mycorrhizal fungi significantly increases soluble sugar and soluble protein contents (Feng et al 2002; Sheng et al 2011). A proteomic analysis showed that protein and carbohydrate synthesis and photosynthesis increase in Populus canescens seedlings after inoculation with ECMF (Szuba et al 2020). Thus, ectomycorrhizal fungi clearly promote protein and carbohydrate accumulation, which is strongly reliant on photosynthesis (Aasamaa et al 2010). An increase in the soluble protein content of leaves can also indirectly increase the activity of key photosynthesis-related enzymes. Moreover, higher soluble sugar levels can increase the photosynthetic capacity, leading to an increase in dry matter (Xu et al 2021).
Larger root systems, as found for the inoculated seedlings in our present study, can take up more water and nutrients from the soil, which are needed for increases in biomass (Chen et al 2019). The larger root systems with larger root diameters and more numerous root tips of the inoculated seedlings indicate greater capacity for absorption (Zhai et al 2015; Qi et al 2019; Wang et al 2021a).
Inoculation with mycorrhizal fungi can improve host plant resistance to adverse environmental conditions (Diagne et al 2020). For example, mycorrhizae improve plant drought tolerance by increasing photosynthetic, osmoregulatory, and antioxidant capacity (Lehto and Zwiazek 2011; Karti et al 2012; Liu et al 2015) and salinity resistance by reducing nutrient deficits, maintaining the ion balance, and mitigating ion damage to plasma membranes and enzymes (Kumar et al 2015). Mycorrhizae can also prevent heavy metals from entering the plant root system and secreting chelates to precipitate them (Ashford et al 1988; Dighton and White 2017) and preventing pathogen invasion and inhibit pathogen growth (Tang et al 2008; Blom et al 2009). Therefore, ectomycorrhizal inoculation can promote seedling growth and improve seedling resistance to adverse environmental conditions, thereby improving seedling quality in all aspects.
The mechanisms by which ectomycorrhizal fungi promote the growth of poplar plants have not yet been studied. Although we did not design experiments to study the mechanisms, our results showing that photosynthetic pigments levels were significantly higher in P. davidiana × P. bolleana after inoculation with ectomycorrhizal fungi, indicate that an early increase in photosynthetic capacity, a prerequisite for rapid growth and a potential mechanism for the growth promotion in Populus davidiana × P. bolleana. Of course, the increase in the absorptive area of plant roots will promote the absorption of nutrients and waters that are required for plant growth. However, the specific mechanisms that cause these increases requires further research.
The colonization of Populus davidiana × P. bolleana seedlings, by the introduced ectomycorrhizal fungi Suillus luteus and Paxillus involutus enhanced the photosynthetic capacity, soluble proteins and sugars and cellulose content of the seedlings, which grew larger stems, leaves and root systems (Fig. 8). The larger roots also improve the absorptive capacity for water and essential nutrients for improved growth. We speculate that these seedlings can also generate a significant amount of antioxidant enzymes, which can enhance their tolerance to stresses (Ma et al 2024). The rise in the levels of intracellular osmoregulatory substances such as sugars can also help maintain turgor pressure and increase tolerance to unfavorable conditions.
Toward improving the vigor and survival of afforested plants in ecologically fragile areas of Northeast China, future research should focus on understanding the mechanisms by which ectomycorrhizal fungi enhance the growth of Populus davidiana × P. bolleana trees and develop the best methods for improving tolerance to stresses such as drought and salinity and resistance to disease. Additionally, because S. luteus was isolated from Liaoning Province, it should be particularly suitable for high-quality reforestation plantations in Northeast China.
Conclusion
Suillus luteus and Paxillus involutus colonized the roots of Populus davidiana × P. bolleana and significantly increased root length, surface area, projected area, mean diameter, volume, and root tip number in comparison to the uninoculated controls. Seedling height, ground diameter, biomass, leaf area and chlorophylls and carotenoids, cellulose, and soluble sugars and proteins also significantly increased. S. luteus was more effective than P. involutus.
References
Aasamaa K, Heinsoo K, Holm B (2010) Biomass production, water use and photosynthesis of Salix clones grown in a wastewater purification system. Biomass Bioenergy 34(6):897–905. https://doi.org/10.1016/j.biombioe.2010.01.035
Ashford AE, Peterson CA, Carpenter JL, Cairney JWG, Allaway WG (1988) Structure and permeability of the fungal sheath in the Pisonia mycorrhiza. Protoplasma 147(2):149–161. https://doi.org/10.1007/BF01403343
Berta G, Trotta A, Fusconi A, Hooker JE, Munro M, Atkinson D, Giovannetti M, Morini S, Fortuna P, Tisserant B, Gianinazzi-Pearson V, Gianinazzi S (1995) Arbuscular mycorrhizal induced changes to plant growth and root system morphology in Prunus cerasifera. Tree Physiol 15(5):281–293. https://doi.org/10.1093/treephys/15.5.281
Blom JM, Vannini A, Vettraino AM, Hale MD, Godbold DL (2009) Ectomycorrhizal community structure in a healthy and a Phytophthora-infected chestnut (Castanea sativa Mill.) stand in central Italy. Mycorrhiza 20(1):25–38. https://doi.org/10.1007/s00572-009-0256-z
Chen L, Swenson NG, Ji NN, Mi XC, Ren HB, Guo LD, Ma KP (2019) Differential soil fungus accumulation and density dependence of trees in a subtropical forest. Science 366(6461):124–128. https://doi.org/10.1126/science.aau1361
Das D, Paries M, Hobecker K, Gigl M, Dawid C, Lam HM, Zhang JH, Chen MX, Gutjahr C (2022) Phosphate starvation response transcription factors enable arbuscular mycorrhiza symbiosis. Nat Commun 13(1):477. https://doi.org/10.1038/s41467-022-27976-8
de Vries FT, Griffiths RI, Knight CG, Nicolitch O, Williams A (2020) Harnessing rhizosphere microbiomes for drought-resilient crop production. Science 368(6488):270–274. https://doi.org/10.1126/science.aaz5192
Diagne N, Ngom M, Djighaly PI, Fall D, Hocher V, Svistoonoff S (2020) Roles of arbuscular mycorrhizal fungi on plant growth and performance: importance in biotic and abiotic stressed regulation. Diversity 12(10):370. https://doi.org/10.3390/d12100370
Dighton J, White JF (2017) The fungal community: its organization and role in the ecosystem. CRC Press, Boca Raton, pp 25–30. https://doi.org/10.1201/9781315119496
Evelin H, Kapoor R, Giri B (2009) Arbuscular mycorrhizal fungi in alleviation of salt stress: a review. Ann Bot 104(7):1263–1280. https://doi.org/10.1093/aob/mcp251
Feng G, Zhang FS, Li XL, Tian CY, Tang C, Rengel Z (2002) Improved tolerance of maize plants to salt stress by arbuscular mycorrhiza is related to higher accumulation of soluble sugars in roots. Mycorrhiza 12(4):185–190. https://doi.org/10.1007/s00572-002-0170-0
Gao FJ (2006) Plant physiology test guide. Higher Education Press, Beijing, pp 74–144
Genre A, Lanfranco L, Perotto S, Bonfante P (2020) Unique and common traits in mycorrhizal symbioses. Nat Rev Microbiol 18(11):649–660. https://doi.org/10.1038/s41579-020-0402-3
Gong MG, Tang M, Chen H, Zhang QM, Feng XX (2013) Effects of two Glomus species on the growth and physiological performance of Sophora davidii seedlings under water stress. New for 44(3):399–408. https://doi.org/10.1007/s11056-012-9349-1
Huang Y, Liu H, Jia ZC, Fang Q, Luo KM (2012) Combined expression of antimicrobial genes (Bbchit1 and LJAMP2) in transgenic poplar enhances resistance to fungal pathogens. Tree Physiol 32(10):1313–1320. https://doi.org/10.1093/treephys/tps079
Karti PH, Astuti DA, Nofyangtri S (2012) The role of arbuscular mycorrhizal fungi in enhancing productivity, nutritional quality, and drought tolerance mechanism of Stylosanthes seabrana. Med Pet 35(1):67–72. https://doi.org/10.5398/medpet.2012.35.1.67
Kumar A, Dames JF, Gupta A, Sharma S, Gilbert JA, Ahmad P (2015) Current developments in arbuscular mycorrhizal fungi research and its role in salinity stress alleviation: a biotechnological perspective. Crit Rev Biotechnol 35(4):461–474. https://doi.org/10.3109/07388551.2014.899964
Lehto T, Zwiazek JJ (2011) Ectomycorrhizas and water relations of trees: a review. Mycorrhiza 21(2):71–90. https://doi.org/10.1007/s00572-010-0348-9
Liu D (2021) Managing both internal and foreign affairs—a PHR-centered gene network regulates plant-mycorrhizal symbiosis. Chin Bull Bot 56(6):647–650. https://doi.org/10.11983/CBB21177
Liu T, Sheng M, Wang CY, Chen H, Li Z, Tang M (2015) Impact of arbuscular mycorrhizal fungi on the growth, water status, and photosynthesis of hybrid poplar under drought stress and recovery. Photosynthetica 53(2):250–258. https://doi.org/10.1007/s11099-015-0100-y
Liu YL, Xin ZB, Li ZS, Keyimu M (2020) Climate effect on the radial tree growth of Populus simonii in Northwest of Hebei for last four decades. Acta Ecol Sin 40(24):9108–9119. https://doi.org/10.5846/stxb202003040406
Ma CX, Zhang Y, Kong DX, Gao Y, Xu LJ, Meng W (2024) Analysis of fungi from Parametarhizium on improving the growth of mung beans under salt and alkali stress. Bull Bot Res 44(2):239–247. https://doi.org/10.7525/j.issn.1673-5102.2024.02.009
Mathur N, Vyas A (2000) Influence of arbuscular mycorrhizae on biomass production, nutrient uptake and physiological changes in Ziziphus mauritiana Lam. under water stress. J Arid Environ 45(3):191–195. https://doi.org/10.1006/jare.2000.0644
Moran JF, Becana M, Iturbe-Ormaetxe I, Frechilla S, Klucas RV, Aparicio-Tejo P (1994) Drought induces oxidative stress in pea plants. Planta 194(3):346–352. https://doi.org/10.1007/BF00197534
Nasir RW, Tanweer HM (2014) Role of poplars in agroforestry systems in India. New York Science Journal 7(2):50–56. https://doi.org/10.7537/marsnys070214.06
Niu S (1992) Object quality analysis. China Agricultural Press, Beijing, pp 62–65
Podila GK, Sreedasyam A, Muratet MA (2009) Populus rhizosphere and the ectomycorrhizal interactome. Crit Rev Plant Sci 28(5):359–367. https://doi.org/10.1080/07352680903241220
Qi JY, Deng JF, Yin DC, Cai LX, Liu DP, Zhang LL, Lin M (2019) Effects of inoculation of exogenous mycorrhizal fungi on the antioxidant and root configuration enzyme activity of Pinus tabulaeformis seedlings. Acta Ecol Sin 39(8):2826–2832. https://doi.org/10.5846/stxb201805091027
Quoreshi AM, Khasa DP (2008) Effectiveness of mycorrhizal inoculation in the nursery on root colonization, growth, and nutrient uptake of aspen and balsam poplar. Biomass Bioenergy 32(5):381–391. https://doi.org/10.1016/j.biombioe.2007.10.010
Richardson AE, Barea JM, McNeill AM, Prigent CC (2009) Acquisition of phosphorus and nitrogen in the rhizosphere and plant growth promotion by microorganisms. Plant Soil 321(1–2):305–339
Rooney DC, Killham K, Bending GD, Baggs E, Weih M, Hodge A (2009) Mycorrhizas and biomass crops: opportunities for future sustainable development. Trends Plant Sci 14(10):542–549. https://doi.org/10.1016/j.tplants.2009.08.004
Scheublin TR, Sanders IR, Keel C, van der Meer JR (2010) Characterisation of microbial communities colonising the hyphal surfaces of arbuscular mycorrhizal fungi. ISME J 4(6):752–763. https://doi.org/10.1038/ismej.2010.5
Sheng M, Tang M, Chen H, Yang BW, Zhang FF, Huang YH (2008) Influence of arbuscular mycorrhizae on photosynthesis and water status of maize plants under salt stress. Mycorrhiza 18(6–7):287–296. https://doi.org/10.1007/s00572-008-0180-7
Sheng M, Tang M, Zhang FF, Huang YH (2011) Influence of arbuscular mycorrhiza on organic solutes in maize leaves under salt stress. Mycorrhiza 21(5):423–430. https://doi.org/10.1007/s00572-010-0353-z
Smith FA, Grace EJ, Smith SE (2009) More than a carbon economy: nutrient trade and ecological sustainability in facultative arbuscular mycorrhizal symbioses. New Phytol 182(2):347–358. https://doi.org/10.1111/j.1469-8137.2008.02753.x
Song RQ, Wang F, Ji RQ, Qi JY (2007) Effect of ectomycorrhizal fungi on the seedlings growth of Korea spruce. Acta Microbiol Sin 47(6):1091–1094. https://doi.org/10.13343/j.cnki.wsxb.2007.06.025
Szuba A, Marczak Ł, Ratajczak I, Kasprowicz-Maluśki A, Mucha J (2020) Integrated proteomic and metabolomic analyses revealed molecular adjustments in Populus × canescens colonized with the ectomycorrhizal fungus Paxillus involutus, which limited plant host growth. Environ Microbiol 22(9):3754–3771. https://doi.org/10.1111/1462-2920.15146
Tang M, Zhang RQ, Chen H, Zhang HH, Tian ZQ (2008) Induced hydrolytic enzymes of ectomycorrhizal fungi against pathogen Rhizoctonia solani. Biotechnol Lett 30(10):1777–1782. https://doi.org/10.1007/s10529-008-9760-z
Wang C, Xie HX, Liu RJ, Li W, Guo SX, Li M (2021) Salt tolerance of watermelon plants through AM fungus adjusting root architecture and mineral element balance. Mycosystema 40(10):2800–2810. https://doi.org/10.13346/j.mycosystema.210222
Wang JX, Zhang HQ, Gao J, Zhang Y, Liu YQ, Tang M (2021b) Effects of ectomycorrhizal fungi (Suillus variegatus) on the growth, hydraulic function, and non-structural carbohydrates of Pinus tabulaeformis under drought stress. BMC Plant Biol 21(1):171. https://doi.org/10.1186/s12870-021-02945-3
Wang XF, Hao LF, Hao JX, Hao WY, Bao HG, Bai SL (2021) Growth responses of Pinus sylvestris var. mongolica seedlings under simulated nitrogen deposition and different inoculation of ectomycorrhizal fungi treatments. Bull Bot Res 41(1):138–144. https://doi.org/10.7525/j.issn.1673-5102.2021.01.017
Xu N, Song FQ, Fan XX, Sui X, Chang W (2021) Effects of salt stress on growth characteristics of arbuscular mycorrhizal Elaeagnus angustifolia seedlings. J Northeast For Univ 49(6):29–33. https://doi.org/10.13759/j.cnki.dlxb.2021.06.007
Yang YR, Tang M, Sulpice R, Chen H, Tian S, Ban YH (2014) Arbuscular mycorrhizal fungi alter fractal dimension characteristics of Robinia pseudoacacia L. seedlings through regulating plant growth, leaf water status, photosynthesis, and nutrient concentration under drought stress. J Plant Growth Regul 33(3):612–625. https://doi.org/10.1007/s00344-013-9410-0
Yin DC, Deng X, Chet I, Song RQ (2014) Physiological responses of Pinus sylvestris var. mongolica seedlings to the interaction between Suillus luteus and Trichoderma virens. Curr Microbiol 69(3):334–342. https://doi.org/10.1007/s00284-014-0589-5
Yin DC, Yang LB, Deng X, Chet I, Song RQ (2015) How Trichoderma virens affects growth indicators, physiological and biochemical parameters of Pinus sylvestris var. mongolica seedlings. J Beijing For Univ 37(1):78–83. https://doi.org/10.13332/j.cnki.jbfu.2015.01.012
Yin DC, Song RQ, Qi JY, Deng X (2018) Ectomycorrhizal fungus enhances drought tolerance of Pinus sylvestris var. mongolica seedlings and improves soil condition. J For Res 29(6):1775–1788. https://doi.org/10.1007/s11676-017-0583-4
Yin DC, Halifu S, Song RQ, Qi JY, Deng X, Deng JF (2020) Effects of an ectomycorrhizal fungus on the growth and physiology of Pinus sylvestris var. mongolica seedlings subjected to saline–alkali stress. J For Res 31(3):781–788. https://doi.org/10.1007/s11676-019-01007-7
Yin DC, Wang HL, Qi JY (2021) The enhancement effect of calcium ions on ectomycorrhizal fungi-mediated drought resistance in Pinus sylvestris var. mongolica. J Plant Growth Regul 40(4):1389–1399. https://doi.org/10.1007/s00344-020-10197-y
Zai XM, Zhu SN, Qin P, Wang XY, Che L, Luo FX (2012) Effect of Glomus mosseae on chlorophyll content, chlorophyll fluorescence parameters, and chloroplast ultrastructure of beach plum (Prunus maritima) under NaCl stress. Photosynthetica 50(3):323–328. https://doi.org/10.1007/s11099-012-0035-5
Zhai SS, Ding GJ, Wang Y, Luo XM, Li M (2015) Effects of Suillus luteus on root architecture of Pinus massoniana. J For Enviro 35(3):243–248. https://doi.org/10.13324/j.cnki.jfcf.2015.03.010
Zhang YX, Bi YL, Shen HH, Zhang LJ (2020) Arbuscular mycorrhizal fungi enhance sea buckthorn growth in coal mining subsidence areas in Northwest China. J Microbiol Biotechnol 30(6):848–855. https://doi.org/10.4014/jmb.1907.07007
Zheng J, Hu MJ, Guo YP (2008) Regulation of photosynthesis by light quality and its mechanism in plants. Chin J Appl Ecol 19(7):1619–1624. https://doi.org/10.13287/j.1001-9332.2008.0264
Author information
Authors and Affiliations
Corresponding author
Additional information
Publisher's Note
Springer Nature remains neutral with regard to jurisdictional claims in published maps and institutional affiliations.
Project funding: This study was part of the Liaoning Provincial Department of Education project LJKZ0684 and supported by the National Natural Science Foundation of China (31800542).
The online version is available at https://springerlink.bibliotecabuap.elogim.com/.
Corresponding editor: Tao Xu.
Rights and permissions
Springer Nature or its licensor (e.g. a society or other partner) holds exclusive rights to this article under a publishing agreement with the author(s) or other rightsholder(s); author self-archiving of the accepted manuscript version of this article is solely governed by the terms of such publishing agreement and applicable law.
About this article
Cite this article
Zhang, T., Meng, F. & Yin, D. Promotion of biomass, photosynthesis, and root growth of seedling biomass, photosynthesis, and root growth of Populus davidiana × P. bolleana by two species of ectomycorrhizal fungi. J. For. Res. 35, 101 (2024). https://doi.org/10.1007/s11676-024-01756-0
Received:
Accepted:
Published:
DOI: https://doi.org/10.1007/s11676-024-01756-0