Abstract
The adsorption of acrolein (AC) onto the surface of B36 borophene nanosheet was studied using dispersion-corrected density functional theory (DFT). The structural and electronic properties were scrutinized by several quantum chemical parameters such as HOMO–LUMO gap, condensed Fukui function, molecular electrostatic potential (ESP), and the density of states (DOS). The non-covalent interactions (NCI) were explored by combined reduced density gradient (RDG-NCI) and energy decomposition analysis (EDA) techniques. It was found that the adsorption of acrolein on both convex and concave surfaces of borophene is mainly governed by van der Waals interactions. Our calculations showed that the adsorption energy is strengthened and favored when multiple acrolein molecules adsorb on the edge sides of borophene through their terminal carbonyl oxygen atom. Furthermore, the calculated HOMO–LUMO energy gaps were significantly reduced upon adsorption affecting, therefore, the electrical conductance of borophene. These results should be useful in designing acrolein sensors.
Similar content being viewed by others
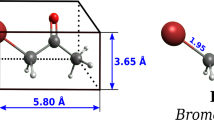
Avoid common mistakes on your manuscript.
Introduction
The recent emergence of new two-dimensional (2D) materials opened up a diversity of new applications in the fields of environment, energy storage, electronics, and biomedical engineering [1,2,3,4,5,6,7,8,9,10,11]. The first two-dimensional material known as graphene was synthesized by Novoselov et al. in 2004 [12]. Since its discovery, graphene has been a focus of numerous theoretical and experimental studies [13,14,15,16,17,18,19,20].
Very recently, new post-graphene 2D materials such as stanene [21,22,23,24], germanene [25], bismuthene [26], and borophene [27,28,29,30,31] were discovered after being theoretically predicted. Particular attention is drawn to the borophene, an allotropic form of two-dimensional boron defined as boron sheet which has been synthesized by depositing vaporizing boron on silver Ag(111) substrate [27]; the miscellaneous bonding configurations adopted by boron nanosheets allow the borophene to exhibit a polymorphism and different crystal structures, consisting of combined hexagonal and triangular motifs, thus resulting in the following most common polymorphs denominated β12 and χ3 and striped–borophenes [27, 28]. Diverse borophene allotropes could be obtained experimentally by controlling the synthesis conditions such as the nature of the substrate and its crystallographic orientation dependence [32].
New architectures based on 2D boron nanosheets have also been developed to enrich the class of borophene clusters [33,34,35,36,37]. Among them, the smallest borophene (B36) cluster [38] having sixfold symmetry was experimentally evidenced and described as a highly stable flattened bowl-shaped boron cluster bearing a hexagonal vacancy at the center [39,40,41]. The latest reports show that borophene, with its both metallicity and lightweight properties [27], has an interesting potential for catalysis, hydrogen storage, and high capacity electrode [42,43,44,45,46]. Additionally, borophene is a promising chemical sensor material and could be used for making sensing devices for detecting toxic substances [47, 48].
Acrolein (AC) or 2-propenal is an organic compound and the simplest unsaturated three-carbon aldehyde; it is used mainly as a pesticide and as a precursor for producing acrylic acid. However, exposure to the acrolein vapor causes mucosal inflammation; it is highly toxic if inhaled and irritating to the skin, eyes, and upper respiratory tract even at low levels of concentrations; acrolein released from tobacco smoke is associated with respiratory toxicity [49]; it was also reported that acrolein could potentially be human carcinogenic [50].
Due to the importance of designing high sensitive devices capable of detecting acrolein, a number of computational investigations focused on the adsorption of acrolein on various adsorbent materials were carried out [51,52,53,54,55,56,57,58]. The ability of pristine and Al-doped graphenes to adsorb acrolein has been studied using DFT by Rastegar et al. [51]; their investigations confirmed the sensitivity of Al-doped graphene toward acrolein. Karami [52] reported a DFT study of acrolein adsorption on graphyne nanotube and revealed the potential of graphyne sheets for acrolein sensing applications. Pirillo et al. [55] explored theoretically the adsorption properties of acrolein on Pt(111); the authors indicated that the adsorption process is mainly due to the formation of C–Pt bonds.
Quantum chemical calculations may provide a tremendous insight regarding the adsorption process including the nature of involved interactions; it is worth noting that numerous 2D materials were predicted theoretically before being experimentally confirmed.
Herein, the plausibility of adsorption of acrolein on borophene B36 was assessed with dispersion-corrected density functional calculations; geometrical parameters and electronic structures, as well as the adsorption properties of the interacting systems, have been examined systematically.
Computational details
The geometries of all studied structures in this work were optimized using DFT-D3 methodology where dispersion correction with Becke–Johnson D3(BJ) and standard zero D3(0) damping functions are applied to account for the dispersion interactions. B3LYP-D3(BJ) [59,60,61,62], B97-D3(BJ) [60, 63], and M06-2X-D3(0) [59, 60, 64] levels of calculations were employed and combined with the def2-SVP [65,66,67] basis set in vacuum. These computations were carried out with ORCA program package [68] (version-4.2.0). For improving the computational efficiency procedure, the resolution of identity (RI) and chain of spheres exchange (COSX) approximations were applied [69,70,71]. A geometrical counterpoise correction (gCP) was incorporated to account for the basis set superposition error (BSSE) [72]. The frequency calculations were performed in order to confirm and verify the minima of all obtained stationary points. The visualization of the molecular orbitals was performed with the Avogadro software [73]. Analysis of weak intramolecular and intermolecular interactions between AC molecule and B36 nanosheet was carried out with the non-covalent interaction-reduced density gradient analysis approach (NCI-RDG) [74] using the Multiwfn software [75] and VMD visualization program [76]. The energy decomposition analysis (EDA) was determined with the GAMESS code [77] by the M06-2X [62] functional and 6-31G(d,p) basis set [78].
The adsorption energy is calculated according to the following expression [79,80,81,82,83,84,85,86,87,88,89,90]:
where E[B36(AC)n] corresponds to the total energy of the B36(AC)n complex, E(B36) is the energy of the isolated B36 nanosheet, and E(AC) is the energy of the isolated single AC molecule.
The change in the HOMO–LUMO gap |ΔEg|, as an indicator of the electronic sensitivity of the B36 toward the dissociative adsorption of AC molecule, is obtained by:
where Eg1 and Eg2 are, respectively, the values of Eg in the initial and the final states.
Results and discussion
Properties of B36 borophene
The optimized geometry of the B36 borophene nanosheet was determined by the following theoretical levels of calculations: B3LYP-D3(BJ)/def2-SVP, B97-D3(BJ)/def2-SVP, and M06-2X-D3(0)/def2-SVP. Our results are corroborated by the experimental data [38] and previous theoretical [79,80,81,82,83,84,85,86] studies, indicating that the B36 borophene is consisting of three different sizes of rings: internal (B1–B6), median (B7–B18), and external rings (B19–B36), as shown in Fig. 1. The B36 borophene bears a quasi-planar structure with a central hexagonal hole and exhibits a perfect hexagonal C6v symmetry [48, 81]. The optimized structure at B97-D3(BJ)/def2-SVP predicts different B–B bond lengths ranging between 1.609 and 1.749 Å and close to the values calculated by Rostami and Soleymanabadi [80]. Frequency calculations were performed at the same level of theory for the reported structures without producing imaginary frequencies.
The calculated electronic parameters, such as the frontier molecular orbital energy (EHOMO, ELUMO) and HOMO–LUMO gap energy |Eg|, offer a valuable method for evaluating the reactivity and stability of such molecular systems [81,82,83,84,85,86], where smaller Eg (absolute values) indicates high reactivity, whereas larger values of |Eg| show a greater stability [91, 92].
Based on the calculated values of |Eg| (Table 1), it was found that the neutral B36 nanosheet is more reactive than cationic and anionic species, following the order: B36 >\( {B}_{36}^{-} \)>\( {B}_{36}^{+} \). The larger HOMO–LUMO gaps predicted by M06-2X functional are due to its higher amount of exact Hartree-Fock (HF) exchange (54%). These results are in agreement with previous similar studies [93,94,95] which concluded that increasing HF exchange percentage in DFT functionals leads to the increase of HOMO–LUMO gap.
The condensed Fukui function (Fig. 2) and molecular electrostatic (ESP) potential (Fig. 3) were used as reactivity descriptors in assessment and prediction of the most reactive centers, especially nucleophilic and electrophilic sites.
In the literature [91,92,93,94, 96], it has been reported that the preferred center for electrophilic attack is the atom in the molecule where the value of f− is the highest, while the site for nucleophilic attack is the atom in the molecule where the value of f+is the highest.
The calculated values of the Fukui functions (f+ and f−) are illustrated in Fig. 2. The results predict that \( {f}_{\mathrm{k}}^{+} \) values are highest on the external rings of neutral B36 borophene, precisely on the corner sites (B21, B24, B27, B30, B33, and B36 atoms), suggesting that these sites would be most favorable for nucleophilic attack. The most susceptible sites for electrophilic attacks are B2, B5, B7, and B13 atoms, followed by atoms of the corner sites B21, B24, B27, B30, B33, and B36. This observation clearly establishes that the most reactive sites are those located at the corner sites of neutral B36, susceptible to both nucleophilic and electrophilic attacks. These findings are supported by several studies [48, 82, 84] indicating that the edge boron atoms of neutral B36 borophene are more reactive than the inner atoms.
Figure 3 shows the ESP maps of the van der Waals surface of the optimized neutral B36, in which the blue and red regions on the scale bar indicate respectively the lowest and highest ESP energy values. The surface local minima and maxima of ESP are represented respectively by cyan and orange spheres.
The inspection of the calculated ESP plots on the van der Waals surface revealed that the local minima of ESP surface are dispersed near the outer side of B36 (external ring “B19–B36”), with larger negative values (− 5.874 to − 5.907 kcal mol−1); thus, they could act as reactive sites and also may favor the electrophilic reaction.
The positive ESP regions on the van der Waals surface with a large value (i.e., ˃ 17 kcal mol−1) are distributed in both convex and concave sides of B36 surface, in which their local maxima of ESP are significant but relatively larger on the concave side (21.618 to 21.653 kcal mol−1) than convex side (17.451 to 17.546 kcal mol−1). This interesting observation suggests that the concave side is chemically more reactive than the convex side.
Adsorption of single acrolein molecule on the B36 borophene
In order to find and examine the most stable adsorption geometries, several initial configurations of AC adsorption on the B36 borophene were investigated, taking into consideration the convex and concave shapes of the B36 borophene surface and various possible orientations of AC molecule. With this aim, we have explored various sites by placing the oxygen atom of the acrolein molecule on top of the boron atoms, and also by orienting the AC molecule both in perpendicular and parallel directions to the B36 borophene sheet, as shown in supplementary data (see Fig. S1).
Different configurations for the adsorption of the acrolein on B36 borophene were considered in this study. However, only geometries with local minima obtained at the B97-D3(BJ)/def2-SVP level of theory were kept, in which the calculated adsorption energy was higher than − 0.99 kcal mol−1, indicating that the adsorption is spontaneous and the process is exothermic. Three local minima are selected with an adsorption energy higher or equal to − 9.8 kcal mol−1. Figure 4(B)–(D) show the optimized geometries of the three most stable configurations, and their associated frontier molecular orbital distributions (HOMO and LUMO).
The most stable formed complex is (B), in which the AC molecule is attached via the oxygen atom of its carbonyl group to the boron atom of corner (edge) site of B36 structure. The calculated adsorption energy is − 25.20 kcal mol−1, and the newly formed bond length B–O was 1.466 Å. As shown in Table 2, the maximum change of |ΔEg| has been observed in the edge configuration (|ΔEg| = 67.10%), where both HOMO and LUMO levels were shifted respectively to higher and lower energies, thus resulting in the significant decrease of HOMO–LUMO gap.
From Table 2, it can be observed that the adsorption energies decrease in the following order: Eads edge > Eads concave > Eads convex. The calculated density of states (DOS) for B36 nanosheet and its three complexes AC@B36 edge, AC@B36 concave, and AC@B36 convex is illustrated in Fig. 5. The results clearly reveal that the AC@B36 edge complex affects strongly the electronic properties of B36 borophene if compared with both AC@B36 concave and AC@B36 convex.
For C and D complexes (Fig. 4), the AC molecule is horizontally adsorbed on the concave and convex surfaces of B36 borophene with the adsorption energies of − 15.28 and − 9.60 kcal mol−1 respectively, indicating a weak interaction. This observation is obviously consistent with our previous ESP analysis (Fig. 3) which predicted that the concave side of B36 borophene is relatively more reactive than the convex side.
Furthermore, we introduced the non-covalent interaction (NCI) analysis based on the reduced density gradient (RDG) function, in order to analyze the intermolecular interactions and to distinguish the nature of the interactions between the AC molecule and B36 nanosheet. The RDG quantities are calculated by the following equation [93, 94, 96,97,98,99,100]:
where ρ and ∇ρ are density and its first derivative, respectively. The regions and types of interactions between AC molecule and B36 nanosheet can be identified and visualized by colored RDG isosurface, in which the blue regions represent strong attractive interactions like H-bond, green denotes weak van der Waals interactions, and the red regions represent steric repulsion.
The RDG isosurfaces for the three complexes are shown in Fig. 6, with the color ranging from blue to red corresponding to the value of − 0.05 < ρ*sign(λ2) < 0.05 a.u.
For AC@B36 concave and AC@B36 convex complexes, larger green isosurfaces (dark green and light green) were observed between AC molecule and B36 nanosheet, corresponding to the ranges between − 0.010 and − 0.015 a.u. (light green) and between − 0.01 a.u. and − 0.015 a.u. (dark green), which are characteristic of intermolecular van der Waals interactions, confirming that van der Waals (vdW) interactions are the key driving force for the adsorption of acrolein molecule on the B36 borophene in both AC@B36 concave and AC@B36 convex complexes.
In addition, for the AC@B36 edge complex, two green isosurfaces were determined. The first is located between hydrogen atom (H41) of AC molecule and boron atom (B20) of B36 borophene. The second isosurface is an intramolecular interaction occurring between oxygen (O42) of the carbonyl group and hydrogen atom (H44) of AC molecule. These weak van der Waals interactions contribute to global stability and explain the orientation of AC molecule in the AC@B36 edge complex.
Energy decomposition analysis (EDA) was performed with GAMESS code for the analysis of intermolecular interactions between the acrolein and B36 borophene using M06-2X functional which is recommended for predicting and describing non-covalent interactions and 6-31G(d,p) basis set. The total interaction energy obtained from a complex calculation (AC@B36) in both concave and convex configurations is decomposed into electrostatic, exchange, repulsion, and polarization terms; the computed decomposed energy terms are reported in Table 3.
From Table 3, it is clear that dispersive energy dominates the attractive energy components and provides the largest contribution to the total interaction energy, the dispersive energy of AC@B36 concave is greater by 7.42 kcal mol−1 than that of AC@B36 convex; however, the repulsion energy due to the destabilization is larger for AC@B36 concave (+ 35.44 kcal mol−1) and higher than AC@B36 convex by 5.36 kcal mol−1. These results are consistent with those obtained from the RDG-NCI analysis confirming that vdW dispersion interactions play a key role in the stabilization of both complexes.
Adsorption of multiple acrolein molecules on the B36 borophene
In order to examine the concentration effect on the adsorption of several acrolein molecules, we have investigated the adsorption of up to six acrolein molecules on the B36 surface. For this purpose, we firstly selected the most stable complex with a singly edge-adsorbed acrolein, and then, the additional acrolein molecules were added increasingly and successively at the edge, concave, and convex sides, while focusing primarily on the adsorption at the edge sides. A reoptimization procedure was performed for each configuration at B3LYP-D3(BJ)/def2-SVP, B97-D3(BJ)/def2-SVP, and M06-2X-D3(0)/def2-SVP computational levels. For each newly optimized complex, various possible configurations are compared as shown in Fig. 7. The calculated adsorption energy, energy of HOMO and LUMO, HOMO–LUMO gaps, and the percentage of variation of HOMO–LUMO gap |ΔEg| for studied complexes with multiple AC molecules at the B97-D3(BJ)/def2-SVP theoretical level are reported in Table 4. Computational results obtained at B3LYP-D3(BJ)/def2-SVP and M06-2X-D3(0)/def2-SVP theoretical levels are listed in Tables S1 and S2 (see supplementary data).
From the calculated adsorption energies at the B97-D3(BJ)/def2-SVP level (Table 4), it is evident that the most stable complexes are A1, B1, C1, D1, and E1, in which AC molecules are adsorbed at the edge sites of B36 nanosheet.
The results from Table 4 clearly show that the HOMO levels are shifted to upper energies whereas the LUMO levels are shifted to lower energies after the adsorption process, particularly when increasing the number of adsorbed AC molecules, leading to a significant decrease of the HOMO–LUMO energy gap. In addition, the percentage of variation of HOMO–LUMO gap |ΔEg| exhibits greater values following the order: 2AC@36 < 3AC@36 < 4AC@36 < 5AC@b36 ≈ 6AC@B36.
Based on these results, it can be concluded that the concentration of acrolein affects the electronic properties of borophene by decreasing the HOMO–LUMO gap and, therefore, increasing the electrical conductance. Thus, the borophene can serve as a sensing material for the detection of acrolein molecule.
Conclusions
Using DFT calculations, we extensively studied the electronic and geometrical properties of acrolein adsorption on B36 borophene nanosheet. Our results reveal that the B36 borophene bears a quasi-planar structure with a central hexagonal hole, and the neutral B36 nanosheet is more reactive than cationic and anionic species. The calculated values of the Fukui functions show that the most reactive sites are those located at the corner sites of neutral B36, susceptible to both nucleophilic and electrophilic attacks; more specifically, the edge boron atoms are the most stable sites of adsorption. The acrolein molecule is attached to the borophene surface via the oxygen atom of its carbonyl group with an adsorption energy of − 25.20 kcal mol−1. The NCI-RDG analysis highlighted the role of van der Waals interactions in stabilizing the adsorption of acrolein on both convex and concave surfaces of borophene, in accordance with the results of the energy decomposition analysis. Up to six acrolein molecules can be adsorbed preferentially at the edge sides of the B36 borophene. According to the calculated density of states, acrolein molecule affects strongly the electronic properties of B36 borophene by decreasing the HOMO–LUMO gap and, thus, inducing changes in its electrical conductance. B36 borophene nanosheet is expected to be applied as a nanosensor for detecting acrolein.
References
Zhang P, Wang F, Yu M, Zhuang X, Feng X (2018) Two-dimensional materials for miniaturized energy storage devices: from individual devices to smart integrated systems. Chem Soc Rev 47:7426–7451
Schwierz F, Pezoldt J, Granzner R (2015) Two-dimensional materials and their prospects in transistor electronics. Nanoscale 7:8261–8283
Hynek DJ, Pondick JV, Cha JJ (2019) The development of 2D materials for electrochemical energy applications: a mechanistic approach. APL Mater 7:030902
Chhowalla M, Jena D, Zhang H (2016) Two-dimensional semiconductors for transistors. Nat Rev Mater 1:16052
Gupta A, Sakthivel T, Seal S (2015) Recent development in 2D materials beyond graphene. Prog Mater Sci 73:44–126
Wang Z, Mi B (2017) Environmental applications of 2D molybdenum disulfide (MoS2) nanosheets. Environ Sci Technol 51:8229–8244
Jun BM, Kim S, Heo J, Park CM, Her N, Jang M, Huang Y, Han J, Yoon Y (2019) Review of MXenes as new nanomaterials for energy storage/delivery and selected environmental applications. Nano Res 12:471–487
Kemp KC, Seema H, Saleh M, Le NH, Mahesh K, Chandra V, Kim KS (2013) Environmental applications using graphene composites: water remediation and gas adsorption. Nanoscale 5:3149–3171
Wang J, Ma F, Sun M (2017) Graphene, hexagonal boron nitride, and their heterostructures: properties and applications. RSC Adv 7:16801–16822
Kurapati R, Kostarelos K, Prato M, Bianco A (2016) Biomedical uses for 2D materials beyond graphene: current advances and challenges ahead. Adv Mater 28:6052–6074
Pradeep AV, Satya Prasad SV, Suryam LV, Prasanna Kumari P (2019) A review on 2D materials for bio-applications. Mater Today Proc 19:380–383
Novoselov KS, Geim AK, Morozov SV, Jiang D, Zhang Y, Dubonos SV, Grigorieva IV, Firsov AA (2004) Electric field effect in atomically thin carbon films. Science 306:666–669
Geim AK, Novoselov KS (2007) The rise of graphene. Nat Mater 6:183–191
Senese A, Chalifoux W (2019) Nanographene and graphene nanoribbon synthesis via alkyne benzannulations. Molecules 24:118
Hosseinzadeh A, Bidmeshkipour S, Abdi Y, Arzi E, Mohajerzadeh S (2018) Graphene based strain sensors: a comparative study on graphene and its derivatives. Appl Surf Sci 448:71–77
Calogero G, Alcón I, Papior N, Jauho AP, Brandbyge M (2019) Quantum interference engineering of nanoporous graphene for carbon nanocircuitry. J Am Chem Soc 141:13081–13088
Liao L, Peng H, Liu Z (2014) Chemistry makes graphene beyond graphene. J Am Chem Soc 136:12194–12200
Tao J, Tang H, Patra A, Bhattarai P, Perdew JP (2019) Erratum: Modeling the physisorption of graphene on metals. Phys Rev B 97 (2018) 165403. Phys Rev B Condens Matter Mater Phys 99:169901
Tang Q, Zhou Z, Chen Z (2015) Innovation and discovery of graphene-like materials via density-functional theory computations: innovation and discovery of graphene-like materials via DFT computations. WIREs Comput Mol Sci 5:360–379
Rahali S, Belhocine Y, Touzeau J, Tangour B, Maurel F, Seydou M (2017) Balance between physical and chemical interactions of second-row diatomic molecules with graphene sheet. Superlattice Microst 102:45–55
Saxena S, Chaudhary RP, Shukla S (2016) Stanene: atomically thick free-standing layer of 2D hexagonal tin. Sci Rep 6:31073
Gao J, Zhang G, Zhang YW (2016) Exploring Ag(111) substrate for epitaxially growing monolayer stanene: a first-principles study. Sci Rep 6:29107
Zhu F, Chen W, Xu Y, Gao C, Guan D, Liu C, Qian D, Zhang SC, Jia JF (2015) Epitaxial growth of two-dimensional stanene. Nat Mater 14:1020–1025
Yuhara J, Fujii Y, Nishino K, Isobe N, Nakatake M, Xian L, Rubio A, Le Lay G (2018) Large area planar stanene epitaxially grown on Ag(111). 2D Mater 5:025002
Dávila ME, Xian L, Cahangirov S, Rubio A, Le Lay G (2014) Germanene: a novel two-dimensional germanium allotrope akin to graphene and silicene. New J Phys 16:095002
Reis F, Li G, Dudy L, Bauernfeind M, Glass S, Hanke W, Thomale R, Schäfer J, Claessen R (2017) Bismuthene on a SiC substrate: a candidate for a high-temperature quantum spin Hall material. Science 357:287–290
Mannix AJ, Zhou XF, Kiraly B, Wood JD, Alducin D, Myers BD, Liu X, Fisher BL, Santiago U, Guest JR, Yacaman MJ, Ponce A, Oganov AR, Hersam MC, Guisinger NP (2015) Synthesis of borophenes: anisotropic, two-dimensional boron polymorphs. Science 350:1513–1516
Feng B, Zhang J, Zhong Q, Li W, Li S, Li H, Cheng P, Meng S, Chen L, Wu K (2016) Experimental realization of two-dimensional boron sheets. Nat Chem 8:563–568
Tai G, Hu T, Zhou Y, Wang X, Kong J, Zeng T, You Y, Wang Q (2015) Synthesis of atomically thin boron films on copper foils. Angew Chem Int Ed 54:15473–15477
Zhang Z, Yang Y, Gao G, Yakobson BI (2015) Two-dimensional boron monolayers mediated by metal substrates. Angew Chem Int Ed 54:13022–13026
Wu X, Dai J, Zhao Y, Zhuo Z, Yang J, Zeng XC (2012) Two-dimensional boron monolayer sheets. ACS Nano 6:7443–7453
Liu X, Zhang Z, Wang L, Yakobson BI, Hersam MC (2018) Intermixing and periodic self-assembly of borophene line defects. Nat Mater 17:783–788
Boustani I (1997) Systematic ab initio investigation of bare boron clusters: determination of the geometry and electronic structures of Bn (n=2–14). Phys Rev B 55:16426
Li WL, Chen X, Jian T, Chen TT, Li J, Wang LS (2017) From planar boron clusters to borophenes and metalloborophenes. Nat Rev Chem 1:0071
Luo XM, Jian T, Cheng LJ, Li WL, Chen Q, Li R, Zhai HJ, Li SD, Boldyrev AI, Li J, Wang LS (2017) \( {B}_{26}^{-} \): the smallest planar boron cluster with a hexagonal vacancy and a complicated potential landscape. Chem Phys Lett 683:336–341
Zhao J, Wang L, Li F, Chen Z (2010) B80 and other medium-sized boron clusters: core-shell structures, not hollow cages. J Phys Chem A 114:9969–9972
Jian T, Chen X, Li SD, Boldyrev AI, Li J, Wang LS (2019) Probing the structures and bonding of size-selected boron and doped-boron clusters. Chem Soc Rev 48:3550–3591
Piazza ZA, Hu HS, Li WL, Zhao YF, Li J, Wang LS (2014) Planar hexagonal B36 as a potential basis for extended single-atom layer boron sheets. Nat Commun 5:3113
Tang H, Ismail-Beigi S (2007) Novel precursors for boron nanotubes: the competition of two-center and three-center bonding in boron sheets. Phys Rev Lett 99:115501
Yang X, Ding Y, Ni J (2008) Ab initio prediction of stable boron sheets and boron nanotubes: structure, stability, and electronic properties. Phys Rev B 77:041402
Penev ES, Bhowmick S, Sadrzadeh A, Yakobson BI (2012) Polymorphism of two-dimensional boron. Nano Lett 12:2441–2445
Er S, de Wijs GA, Brocks G (2009) DFT study of planar boron sheets: a new template for hydrogen storage. J Phys Chem C 113:18962–18967
Zhang Z, Penev ES, Yakobson BI (2017) Two-dimensional boron: structures, properties and applications. Chem Soc Rev 46:6746–6763
Wang ZQ, Lü TY, Wang HQ, Feng YP, Zheng JC (2019) Review of borophene and its potential applications. Front Phys 14:33403
Jiang HR, Lu Z, Wu MC, Ciucci F, Zhao TS (2016) Borophene: a promising anode material offering high specific capacity and high rate capability for lithium-ion batteries. Nano Energy 23:97–104
Zhang X, Hu J, Cheng Y, Yang HY, Yao Y, Yang SA (2016) Borophene as an extremely high capacity electrode material for Li-ion and Na-ion batteries. Nanoscale 8:15340–15347
Shukla V, Wärnå J, Jena NK, Grigoriev A, Ahuja R (2017) Toward the realization of 2D borophene based gas sensor. J Phys Chem C 121:26869–26876
Omidvar A (2017) Borophene: a novel boron sheet with a hexagonal vacancy offering high sensitivity for hydrogen cyanide detection. Comput Theor Chem 1115:179–184
Yeager RP, Kushman M, Chemerynski S, Weil R, Fu X, White M, Callahan-Lyon P, Rosenfeldt H (2016) Proposed mode of action for acrolein respiratory toxicity associated with inhaled tobacco smoke. Toxicol Sci 151:347–364
Feng Z, Hu W, Hu Y, Tang MS (2006) Acrolein is a major cigarette-related lung cancer agent: preferential binding at p53 mutational hotspots and inhibition of DNA repair. Proc Natl Acad Sci 103:15404–15409
Rastegar SF, Hadipour NL, Tabar MB, Soleymanabadi H (2013) DFT studies of acrolein molecule adsorption on pristine and Al-doped graphenes. J Mol Model 19:3733–3740
Karami AR (2015) Acrolein adsorption on graphyne nanotube: a density functional theory study. Fuller Nanotub Car N 23:885–889
Dheivamalar S, Banu KB (2019) A DFT study on functionalization of acrolein on Ni-doped (ZnO)6 nanocluster in dye-sensitized solar cells. Heliyon 5:e02903
Abbasi A, Sardroodi JJ (2016) The interaction of acrolein with pristine and N-doped TiO2 anatase nanoparticles: a DFT study. Acta Chim Slov 63:713–720
Pirillo S, López-Corral I, Germán E, Juan A (2014) Density functional study of acrolein adsorption on Pt(111). Vacuum 99:259–264
Loffreda D, Jugnet Y, Delbecq F, Bertolini JC, Sautet P (2004) Coverage dependent adsorption of acrolein on Pt(111) from a combination of first principle theory and HREELS study. J Phys Chem B 108:9085–9093
Kang GJ, Chen ZX, Li Z (2011) Acrolein adsorption on gold clusters, a theoretical study of conjugation effect on C=C and C=O interaction with Au clusters. Catal Lett 141:996–1003
Ferullo R, Branda MM, Illas F (2010) Coverage dependence of the structure of acrolein adsorbed on Ag(111). J Phys Chem Lett 1:2546–2549
Grimme S, Antony J, Ehrlich S, Krieg H (2010) A consistent and accurate ab initio parametrization of density functional dispersion correction (DFT-D) for the 94 elements H-Pu. J Chem Phys 132:154104
Grimme S, Ehrlich S, Goerigk L (2011) Effect of the damping function in dispersion corrected density functional theory. J Comput Chem 32:1456–1465
Johnson ER, Becke AD (2006) A post-Hartree-Fock model of intermolecular interactions: inclusion of higher-order corrections. J Chem Phys 124:174104
Becke AD, Johnson ER (2005) A density-functional model of the dispersion interaction. J Chem Phys 123:154101
Becke AD (1997) Density-functional thermochemistry. V. Systematic optimization of exchange-correlation functionals. J Chem Phys 107:8554–8560
Zhao Y, Truhlar DG (2008) The M06 suite of density functionals for main group thermochemistry, thermochemical kinetics, noncovalent interactions, excited states, and transition elements: two new functionals and systematic testing of four M06-class functionals and 12 other functionals. Theor Chem Accounts 120:215–241
Schäfer A, Horn H, Ahlrichs R (1992) Fully optimized contracted Gaussian basis sets for atoms Li to Kr. J Chem Phys 97:2571–2577
Schäfer A, Huber C, Ahlrichs R (1994) Fully optimized contracted Gaussian basis sets of triple zeta valence quality for atoms Li to Kr. J Chem Phys 100:5829–5835
Weigend F (2006) Accurate Coulomb-fitting basis sets for H to Rn. Phys Chem Chem Phys 8:1057–1065
Neese F (2018) Software update: the ORCA program system. Version 4.0: software update. Wiley Interdiscip Rev Comput Mol Sci 8:e1327
Neese F, Wennmohs F, Hansen A, Becker U (2009) Efficient, approximate and parallel Hartree–Fock and hybrid DFT calculations. A ‘chain-of-spheres’ algorithm for the Hartree–Fock exchange. Chem Phys 356:98–109
Neese F (2003) An improvement of the resolution of the identity approximation for the formation of the Coulomb matrix. J Comput Chem 24:1740–1747
Eichkorn K, Treutler O, Öhm H, Häser M, Ahlrichs R (1995) Auxiliary basis sets to approximate Coulomb potentials. Chem Phys Lett 240:283–290
Kruse H, Grimme S (2012) A geometrical correction for the inter- and intra-molecular basis set superposition error in Hartree-Fock and density functional theory calculations for large systems. J Chem Phys 136:154101
Hanwell MD, Curtis DE, Lonie DC, Vandermeersch T, Zurek E, Hutchison GR (2012) Avogadro: an advanced semantic chemical editor, visualization, and analysis platform. J Cheminf 4:17
Johnson ER, Keinan S, Mori-Sánchez P, Contreras-García J, Cohen AJ, Yang W (2010) Revealing noncovalent interactions. J Am Chem Soc 132:6498–6506
Lu T, Chen F (2012) Multiwfn: a multifunctional wavefunction analyzer. J Comput Chem 33:580–592
Humphrey W, Dalke A, Schulten K (1996) VMD: visual molecular dynamics. J Mol Graph 14:33–38
Schmidt MW, Baldridge KK, Boatz JA, Elbert ST, Gordon MS, Jensen JH, Koseki S, Matsunaga N, Nguyen KA, Su S, Windus TL, Dupuis M, Montgomery JA (1993) General atomic and molecular electronic structure system. J Comput Chem 14:1347–1363
Hariharan PC, Pople JA (1973) The influence of polarization functions on molecular orbital hydrogenation energies. Theor Chim Acta 28:213–222
Valadbeigi Y, Farrokhpour H, Tabrizchi M (2015) Adsorption of small gas molecules on B36 nanocluster. J Chem Sci 127:2029–2038
Rostami Z, Soleymanabadi H (2016) N–H bond cleavage of ammonia on graphene-like B36 borophene: DFT studies. J Mol Model 22:70
Rastgou A, Soleymanabadi H, Bodaghi A (2017) DNA sequencing by borophene nanosheet via an electronic response: a theoretical study. Microelectron Eng 169:9–15
Shahbazi Kootenaei A, Ansari G (2016) B36 borophene as an electronic sensor for formaldehyde: quantum chemical analysis. Phys Lett A 380:2664–2668
Solimannejad M, Kamalinahad S, Shakerzadeh E (2017) Lin@B36 (n = 1, 2) nanosheet with remarkable electro-optical properties: a DFT study. J Electron Mater 46:4420–4425
Mohsenpour Z, Shakerzadeh E, Zare M (2017) Quantum chemical description of formaldehyde (HCHO), acetaldehyde (CH3CHO) and propanal (CH3CH2CHO) pollutants adsorption behaviors onto the bowl-shaped B36 nanosheet. Adsorption 23:1041–1053
Shahsavar A, Mohajeri A (2018) Impact of position and number of nitrogen atom substitution on the curvature and hydrogen adsorption properties of metallized borophene. J Mater Sci 53:4540–4553
Li WY, Zhao X, Dang JS (2019) A mechanistic study of B36-supported atomic au promoted CO2 electroreduction to formic acid. J Mater Chem A 7:13935–13940
Hassani A, Mosavian MTH, Ahmadpour A, Farhadian N (2016) A comparative theoretical study of methane adsorption on the nitrogen, boron and lithium doped graphene sheets including density functional dispersion correction. Comput Theor Chem 1084:43–50
Hosseinian A, Asadi Z, Edjlali L, Bekhradnia A, Vessally E (2017) NO2 sensing properties of a borazine doped nanographene: a DFT study. Comput Theor Chem 1106:36–42
Montejo-Alvaro F, Oliva J, Herrera-Trejo M, Hdz-García HM, Mtz-Enriquez AI (2019) DFT study of small gas molecules adsorbed on undoped and N-, Si-, B-, and Al-doped graphene quantum dots. Theor Chem Accounts 138:37
Jin P, Hou Q, Tang C, Chen Z (2015) Computational investigation on the endohedral borofullerenes M@B40 (M=Sc, Y, La). Theor Chem Accounts 134:1–10
Allal H, Belhocine Y, Zouaoui E (2018) Computational study of some thiophene derivatives as aluminium corrosion inhibitors. J Mol Liq 265:668–678
Ammouchi N, Allal H, Belhocine Y, Bettaz S, Zouaoui E (2020) DFT computations and molecular dynamics investigations on conformers of some pyrazinamide derivatives as corrosion inhibitors for aluminum. J Mol Liq 300:112309
Sánchez-Márquez J (2019) New advances in conceptual-DFT: an alternative way to calculate the Fukui function and dual descriptor. J Mol Model 25:123
Wu J, Yan H, Zhong A, Chen H, Jin Y, Dai G (2019) Theoretical and conceptual DFT study of pnicogen- and halogen-bonded complexes of PH2X---BrCl. J Mol Model 25:28
Vessally E, Soleimani-Amiri S, Hosseinian A, Edjlali L, Bekhradnia A (2017) The Hartree-Fock exchange effect on the CO adsorption by the boron nitride nanocage. Phys E 87:308–311
De H, Paul A, Datta A (2017) Theoretical study of Au4 thymine, Au20 and Ag20 uracil and thymine complexes for surface enhanced Raman scattering. Comput Theor Chem 1111:1–13
Pahlavan F, Hosseinnezhad S, Samieadel A, Hung A, Fini E (2019) Fused aromatics to restore molecular packing of aged bituminous materials. Ind Eng Chem Res 58:11939–11953
Ni Y, Pan Y, Zhang J, Gu X, Pan X, Jiang J, Wang Q (2018) Theoretical study on reaction mechanism of thermal decomposition of dialkyl peroxides. Comput Theor Chem 1135:11–17
Zhang L, Wang JM, Wang QR, Zhang DW, Li ZT, Li ZM (2015) Theoretical investigation on SnCl4-catalyzed tandem dimerization/oxy-2-azonia-Cope rearrangements between β,γ-unsaturated ketones and imines. Theor Chem Accounts 134:4
Liang Z, Wang H, Zheng X, Wang X (2020) Theoretical investigation on rotaxanes containing a pyridyl-acyl hydrazone moiety: chemical Z → E and photochemical E → Z isomerizations. Theor Chem Accounts 139:39
Author information
Authors and Affiliations
Corresponding author
Additional information
Publisher’s note
Springer Nature remains neutral with regard to jurisdictional claims in published maps and institutional affiliations.
Electronic supplementary material
ESM 1
(DOCX 587 kb).
Rights and permissions
About this article
Cite this article
Allal, H., Belhocine, Y., Rahali, S. et al. Structural, electronic, and energetic investigations of acrolein adsorption on B36 borophene nanosheet: a dispersion-corrected DFT insight. J Mol Model 26, 128 (2020). https://doi.org/10.1007/s00894-020-04388-3
Received:
Accepted:
Published:
DOI: https://doi.org/10.1007/s00894-020-04388-3