Abstract
Climate change-related alterations of Antarctic sea-ice habitats will significantly impact the interaction of ice-associated organisms with the environment, with repercussions on ecosystem functioning. The nature of this interaction is poorly understood, particularly during the critical period of winter–spring transition. To investigate the role of sea-ice and underlying water-column properties in structuring under-ice communities during late winter/early spring, we used a Surface and Under Ice Trawl to sample animals and environmental properties in the upper 2-m layer under the sea ice in the northern Weddell Sea from August to October 2013. The area of investigation was largely homogeneous in terms of hydrography and sea-ice coverage. We hypothesised that this apparent homogeneity in the physical regime was mirrored in the structure of the under-ice community. The under-ice community was numerically dominated by the copepods Stephos longipes, Ctenocalanus spp. and Calanus propinquus (altogether 67 %), and furcilia larvae of Antarctic krill Euphausia superba (30 %). In spite of the apparent homogeneity of the environment, abundance and biomass distributions at our sampling stations indicated the presence of three community types, following a geographical gradient in the investigation area: (1) high biomass, krill-dominated in the west, (2) high abundance, copepod-dominated in the east, and (3) low abundance, low biomass at the ice edge. Combined analysis with environmental data indicated that under-ice community structure was correlated with sea-ice coverage, chlorophyll a concentration, and bottom depth. The heterogeneity of the Antarctic under-ice community was probably also driven by other factors, such as advection, sea-ice drift, and seasonal progression. The response of under-ice communities to changing sea-ice habitats may thus considerably vary seasonally and regionally.
Similar content being viewed by others
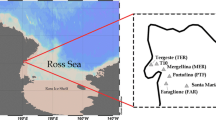
Explore related subjects
Discover the latest articles, news and stories from top researchers in related subjects.Avoid common mistakes on your manuscript.
Introduction
The most prominent environmental feature of the Southern Ocean is a seasonal pack-ice cover varying in extent from 4 million km2 in summer to 20 million km2 in winter (Gloersen and Campbell 1991; Zwally et al. 2002; Turner et al. 2013). Phytoplankton primary production can exceed 2 g C m−2 day−1 during summer and drops to nearly zero in winter (Arrigo et al. 2008). During winter, the main primary production occurs within sea ice, making sea-ice habitats an important seasonal refuge for many species (Siegel and Loeb 1995; Lizotte 2001; Thomas and Dieckmann 2002; Quetin et al. 2013).
Sea ice hosts a specific algal community that can serve as a critical carbon source for young Antarctic krill Euphausia superba (hereafter referred to as Antarctic krill) (O’Brien 1987; Atkinson et al. 2002; Schmidt et al. 2014) and a variety of other species (Hopkins and Torres 1989; Hopkins et al. 1993; Gannefors et al. 2005). Besides ice algae, other resources provided by sea-ice habitats such as protozoans, small copepods, and detritus may offer an alternative food source for species dwelling under the sea ice during winter (Daly 1990; Gannefors et al. 2005; Meyer 2012; Schmidt et al. 2014). Substantial top predator communities foraging in ice-covered regions (Loeb et al. 1997; van Franeker et al. 1997; Ainley et al. 2007, 2012) indicate the potential of the under-ice habitat to sustain large productivity.
The Weddell Sea is one of the most productive sectors of the Southern Ocean (Arrigo et al. 1997, 2008). Its main feature is the Weddell Gyre, which is shaped by the influence of bathymetry and northern islands, enclosing this area as a distinct biogeographical region, entirely sea ice covered during winter (De Broyer et al. 2014). Only few winter studies exist from this area, since logistic difficulties in extreme environmental winter conditions impede field work (Flores et al. 2011; Hunt et al. 2011).
Many studies in the Southern Ocean focused on Antarctic krill (Atkinson et al. 2008; Flores et al. 2012; Meyer 2012), on sea-ice meiofauna (Schnack-Schiel et al. 2001a, b, 2008a, b), or on the pelagic community structure (Pakhomov and Froneman 2004; Schnack-Schiel et al. 2008b; Hunt et al. 2011; Yang et al. 2011), but not much is known about under-ice communities, i.e. meso- and macrofauna dwelling at the sea ice–water interface layer. Previous research has shown that the community near the sea ice–water interface differs from the community in deeper water in terms of species composition and density, and community structure (Flores et al. 2011, 2014). Typically, the surface community is integrated into the epipelagic community (White and Piatkowski 1993; Fisher et al. 2004; Hunt et al. 2011; Giesecke and González 2012). However, it was shown that the surface layer in sea-ice-covered regions hosts a distinct under-ice community, comprised of a mixture of ice-associated and pelagic organisms (Flores et al. 2014). In the Lazarev Sea, Flores et al. (2014) found a marked difference in macrofauna community structure between the 0–2 m surface layer and the 0–200 m epipelagic layer. In addition, Flores et al. (2014) demonstrated a significant response of under-ice macrofauna communities to habitat properties. Under varying habitat properties, the community changed along gradients of surface water temperature and sea ice parameters such as sea-ice coverage, floe size, or distance from the ice edge. When physical gradients were weak during winter, there was no significant structure in the macrofauna community under sea ice (Flores et al. 2014).
Sampling under sea ice is particularly challenging. Most commonly, under-ice fauna, i.e. organisms dwelling at the sea-ice underside or at the ice–water interface layer, have been sampled by scuba divers. This method is excellent in describing the small-scale structure of sea-ice habitats during sampling, yet the larger-scale spatial distribution and variability of the organisms, and species diversity cannot be representatively sampled. Furthermore, there is a need to record habitat properties simultaneously with species sampling on larger spatial scales in order to realistically relate species distribution with their habitat variability and dynamics.
This study aims to investigate the association of metazoan communities in the under-ice water layer (0–2 m) with sea-ice habitat properties in the northern Weddell Sea during late winter/early spring 2013. Using a Surface and Under Ice Trawl (SUIT) with an attached sensors array, we studied the community composition of under-ice fauna, and simultaneously recorded environmental parameters. The sampled waters of the northern Weddell Sea were relatively uniform in terms of hydrography and sea-ice coverage. Our objectives were (1) to provide an inventory of under-ice fauna in the northern Weddell Sea in terms of diversity, abundance, and biomass on a large scale and (2) to test the hypothesis that the apparent uniformity of the physical environment was mirrored by the structure of the under-ice community.
Materials and methods
Sampling technique and data collection
Sampling was performed during RV Polarstern expedition PS 81 (ANT XXIX/7), between 31 August and 2 October 2013, across the ice-covered Weddell Sea, between 61°S, 42°W and 58°S, 25°W (Fig. 1). Eleven stations were sampled, four during daytime and seven during nighttime (Table 1). The first seven stations (Stns 551–567) were sampled from west to east approximately along the 60°S parallel, and the last four stations (Stns 570–579) northward along the 26°W meridian (Fig. 1). These last four stations were positioned at the eastern side of South Sandwich Islands, in shallower waters than the earlier stations (Fig. 1, Table 1). Stations 567–579 were sampled almost 2 weeks after stations 551–565.
SUIT station map during RV Polarstern expedition ANT XXIX/7. Sea-ice concentration acquired from Bremen of University (http://www.iup.uni-bremen.de: 8084/amsr/); sampling was performed from west to east, from August to October 2013. Number codes next to sampling locations indicate station numbers
Horizontal hauls were performed with a Surface and Under Ice Trawl (SUIT) (van Franeker et al. 2009; Flores et al. 2012). The SUIT consisted of a steel frame with a 2 m × 2 m opening and two parallel 15-m long nets attached: (1) a 7-mm half-mesh commercial shrimp net, which covered 1.5 m of the opening width and was lined with 0.3-mm mesh at the rearmost 3 m of the net; and (2) a 0.3-mm mesh zooplankton net, which covered 0.5 m of the opening width. Floats attached to the top of the frame kept the net at the surface or the sea-ice underside. To enable sampling under undisturbed ice, an asymmetric bridle forces the net to shear away from the ship, towing at an angle of approximately 60° to starboard of the ship’s track, at a cable length of 150 m. Depending on the sea-ice conditions, SUIT haul durations varied between 17 and 42 min (mean = 29 min) over an average distance of 1.5 km (Table 1). More information on SUIT sampling during PS81 is available in Schaafsma et al. (2016). A detailed description of the SUIT sampling technique and performance was provided as supplementary material in Flores et al. (2012).
Environmental data
A sensor array was mounted in the SUIT frame, including a conductivity-temperature-depth (CTD) probe with built-in fluorometer, two spectral radiometers, and a video camera. Water inflow speed and direction were estimated using a Nortek Aquadopp® Acoustic Doppler Current Profiler (ADCP). Temperature and salinity profiles were obtained with a Sea and Sun CTD75M probe. Calibration of fluorometric chlorophyll a concentrations was done from water samples obtained during stationary work. The calibration coefficients were derived from the linear relationship between chlorophyll a concentrations of water samples (measured with a Turner 10–AU fluorometer) with fluorometric chlorophyll a concentrations of the corresponding 10 m depth range. Data gaps in the CTD measurements caused by low battery voltage were filled using complementary datasets from the shipboard sensors (temperature, salinity and chlorophyll a at Stns 557, 560 and at Stn 562 only for chlorophyll a), using correction factors determined by linear regression. An altimeter Tritech PA500/6-E connected to the CTD measured the distance between the net and the sea-ice underside. Sea-ice draft was calculated as the difference between the depth of the net relative to the water level, measured by the CTD pressure sensor, and the distance to the sea-ice underside, measured by the altimeter, and corrected for pitch and roll angles. Draft was then converted into sea-ice thickness by using a sea-ice density value of 900 kg m−3. Sea-ice roughness was calculated after the formula:
where (x 1, x 2, …, x n) is the set of n values of sea-ice thickness along one sampling profile.
The trawled area was calculated by multiplying the distance sampled in water, estimated from ADCP data, with the net width (0.5 m for the zooplankton net, and 1.5 m for the shrimp net, respectively).
During each haul, sea-ice concentration (%), sea-ice thickness and snow depth, changes in ship speed and irregularities were estimated visually by an observer on deck. A detailed description of environmental data acquisition was provided in David et al. (2015) and Schaafsma et al. (2016).
Gridded daily sea-ice concentrations for the Southern Ocean derived from AMSR2 satellite data, using the algorithm specified by Spreen et al. (2008), were downloaded from the sea-ice portal hosted by University Bremen and Alfred Wegener Institute (www.meereisportal.de).
Biological data
The catch was partially sorted on board. Ctenophores were immediately extracted from samples, identified, and their volume was measured. Several species were sampled for lipid and stable isotope analyses (data not included in this study). The remaining material was then preserved in 4 % formaldehyde/seawater solution for quantitative analysis. After the cruise, the quantitative samples were analysed for species composition and abundance at the Alfred Wegener Institute. Abundances of macrofauna >0.5 cm were derived from the analysis of the shrimp net and zooplankton net samples, and summed for total abundance. Mesofauna abundances, e.g. Antarctic krill larvae, copepods, and ostracods, were derived from analysis of the zooplankton net samples only. High-abundance zooplankton net samples were fractionated with a plankton splitter (Motoda 1959), and only a sub-sample (1/2–1/8 depending on the sample size) of the original sample was counted and subsequently scaled to the full sample size by multiplication with the subsampling factor. With few exceptions, all animals were identified to the species level, and to developmental stage and sex in Antarctic krill and copepod species. The adult copepods and their juvenile stages were both considered in abundance calculations. Areal abundances were calculated dividing the total number of animals per haul by the trawled area. In all macrofauna species, total body length was measured to the nearest 1 mm, and a mean size per species was used for biomass calculations. Mesofauna biomass was calculated using known species length to weight relationships and was expressed as mg dry weight m−2 (Mizdalski 1988). For copepod species, separated into developmental stages, and ostracods a mean dry weight was theoretically assumed (Mizdalski 1988). A list of all species including names of authors and years of description is presented in Table 2.
Data analysis
The patterns of diversity over the sampling area were investigated by three diversity indices, calculated for the whole biological dataset: (1) species richness (the number of species observed at each station) [S]; (2) the Shannon index [H] (Shannon 1948); and (3) Pielou’s evenness index [J].
Species abundance data were analysed using non-metric multidimensional scaling (NMDS) (Kruskal 1964) based on a Bray–Curtis dissimilarity matrix (Bray and Curtis 1957). The NMDS is commonly regarded as the most robust unconstrained ordination method in community ecology (Minchin 1987). The performance of the NMDS was assessed with Shepard plots and stress values (Clarke and Warwick 2001). A hierarchical clustering of the sampling stations was performed using the Bray–Curtis dissimilarity matrix of the species abundance data (Legendre and Legendre 2012).
To assess the statistical differences between day and night sampling, and geographical location of sampling sites, i.e. proximity to islands, the nonparametric Mann–Whitney–Wilcoxon test was performed on species abundance data (Wilcoxon 1945).
The association of the under-ice community structure with all possible combinations of environmental variables was evaluated with the BioEnv analysis (Clarke and Ainsworth 1993). The BioEnv analysis estimates the subset of environmental variables that has the highest correlation with the biological data. The association of the community structure with the selected subsets of environmental variables was tested for significance with a Mantel test (Mantel 1967). The Mantel test relates two distance matrices, one from the biological and one from the environmental dataset, using Spearman’s correlation. The significance of Mantel test correlations was assessed with a bootstrapping procedure with 999 iterations. For all analyses, R version 3.2.0 was used with the libraries ‘vegan’, ‘FactoMineR’, ‘plyr’ and ‘MASS’ (R Core Team 2015).
Results
Sea-ice habitats
All 11 stations were ice-covered. Satellite-derived sea-ice concentrations at sampling locations, ranged from almost 50 to 100 %, with the lowest values present at the two northernmost stations 577 and 579 (Table 1). Modal sea-ice thickness ranged from 0.2 to 0.7 m. Snow depth ranged between 0.05 and 0.60 m. The sea-ice roughness coefficient generally varied between 0.8 and 2.3, with a maximal value of 3.7 at station 555. Surface-water temperature was on average −1.85 °C (range from −1.83 to −1.87 °C) at a mean salinity of 34 (range 33.6–34.4). Surface-water chlorophyll a concentrations ranged between 0.10 and 0.27 mg m−3.
Variability in species diversity, abundance and biomass distribution
In total, 45 species belonging to 12 phyla were identified in our samples (Table 2). Species richness (S) at most of the stations ranged between 20 and 28. The maximum number of 28 species was encountered at station 557, and the minimum of 9 species at station 577 (Table 3). The highest Shannon diversity (H) was encountered at station 579, and the highest evenness (J) at station 577, in the north-eastern part of the sampled area. The lowest Shannon and evenness indices were found in the centre of the sampled area, at station 560 (Table 3). No spatial patterns were noticed in the distribution of diversity indices.
Among higher level taxa, copepods had the highest abundances, accounting for 67 % of the mean relative abundance over all stations, followed by euphausiids with 30 % (Fig. 2). At most stations, copepods accounted for about 70–95 % of the abundance, whereas at stations 571, 577, and 579 copepods contributed only about 30 %. At these stations, euphausiids dominated with 65 % of the mean abundance (Fig. 2). The other taxonomic groups each accounted for less than 1 % of the abundance, yet most groups had high frequencies of occurrence (Table 2). Exceptions were salps, which were present at only six stations, and ctenophores, which were present at only four stations (Table 2).
Antarctic krill Euphausia superba had the highest biomass as a single species, accounting for 60 % of the mean biomass over all stations, while the other euphausiids together contributed less than 1 % (Fig. 2). Notably, krill heavily dominated total biomass at the western (551–557) and the northern stations (571–579). The second most important biomass-rich taxonomic group was copepods with 17 % of the mean biomass over all stations. Four of the taxonomic groups had a noteworthy contribution to the total biomass: amphipods (4.1 %), polychaetes (5.1 %), chaetognaths (3.9 %), and ctenophores (3.1 %), while the contribution of the remaining taxonomic groups was approximately 1 %.
Cumulative abundances of all species ranged from 0.1 ind. m−2 at station 577 to 18.7 ind. m−2 at station 562 (Fig. 3). The most abundant species were the small calanoid copepods Stephos longipes and Ctenocalanus spp., followed by the larger species Calanus propinquus (Table 2). The ice-associated cyclopoid Pseudocyclopina sp. occurred in higher numbers in the western and central region at stations 551, 555, and 567. The copepods were followed numerically by euphausiids, mainly Antarctic krill larvae and age class 0 juveniles. Sub-adult krill were only encountered in significant abundance at station 551. A detailed description of the population structure of age class 0 krill in the investigation area was provided by Schaafsma et al. (2016). Among the amphipods, the ice-associated species Eusirus laticarpus was numerically dominant at all stations. At station 562, a single fish, Aethotaxis mitopteryx, was caught.
The chaetognaths Eukrohnia hamata and Sagitta spp. were widely distributed over the sampled area, with somewhat increased abundances at the stations with higher copepod abundances (Online Resource 1). E. hamata was highly correlated with the abundances of C. propinquus (r = 0.91), Ctenocalanus spp. (r = 0.68), and to a lesser extent krill larvae (r = 48). Sagitta spp. was correlated with krill larvae (r = 0.56) and Ctenocalanus spp. (r = 0.66) (Online Resource 2).
Cumulative dry-weight biomass of all invertebrate species at each station ranged from 0.1 mg DW m−2 at station 577 to 14.5 mg DW m−2 at station 551 (Fig. 3). Higher biomass encountered at the first three stations was largely driven by the contribution of Antarctic krill. At stations 562 and 567 biomass was shared about equally between Antarctic krill, copepods and ctenophores.
Community structure and association with sea-ice habitat properties
Based on the species abundance and biomass at sampling stations, three community types were visually identified, namely (1) krill-dominated, (2) copepod-dominated, and (3) low biomass/abundance (Fig. 3). The ‘krill-dominated’ community was characterised by high biomass contribution of larval, juvenile, and adult Antarctic krill, yet by relatively low cumulative abundances. The krill-dominated community was present at the westernmost stations 551, 555, and 557 (Fig. 3). The copepod-dominated community had the highest cumulative abundances and was numerically dominated by copepods. It had variable to high biomass values, with a higher contribution from copepods and Antarctic krill and moderate contribution of amphipods, polychaetes, chaetognaths, and ctenophores. This copepod-dominated community was present at stations 560, 562, 565, and 567 in the central part of the investigation area. The low biomass/abundances community was characterised by low cumulative abundances and biomass values. It was dominated by Antarctic krill larvae in abundance and biomass. This community characterised the northernmost four stations 570, 571, 577, and 579, situated in close proximity to the marginal ice zone (MIZ).
The three community types were confirmed by the NMDS ordination and cluster analysis (Fig. 4). The first NMDS axis separated the ‘copepod-dominated’ community type (stations 560–567), which was associated with amphipods, ostracods, Sagitta spp., and the copepods S. longipes and Ctenocalanus spp., from the ‘low biomass/abundances’ community type (stations 570–579), which was associated with Thysanoessa macrura and Salpa thompsoni. The second axis of the NMDS ordination was mainly influenced by station 567 at the upper part of the ordination plot, which was associated with the copepods C. propinquus and Ctenocalanus spp., and Antarctic krill larvae. The ‘krill-dominated’ community stations 551 and 555 were positioned at the lower part of the ordination plot and were associated with sub-adult and juvenile Antarctic krill, ice copepods Pseudocylopina sp., and the pteropods Limacina helicina and Clione limacina. Station 557 had an intermediate position between the ‘krill-dominated’ and the ‘copepod-dominated’ community stations, but was grouped with the ‘krill-dominated’ stations in the cluster analysis.
Water depth alone had the highest correlation, of any single environmental variable, with the variability of species abundances (r = 0.42; Mantel test p = 0.004; Table 4). The highest correlation between species abundance and environmental variables was achieved by a combination of snow depth, sea-ice coverage, temperature, chlorophyll a concentration, and water depth (r = 0.47; Mantel test p = 0.008; Table 4). Summarising these parameters by community type stations, a decrease in water depth, sea-ice coverage, and snow depth and an increase in chlorophyll a concentration was evidenced at the low biomass/abundances group stations compared to krill- and copepod-dominated groups (Table 5). Median species abundances within each community type largely mirrored the NMDS ordination map (Table 5).
Discussion
Sea-ice habitats
During winter 2013, the Southern Ocean had a sea-ice extent of approximately 19 million km2 (Data source: www.meereisportal.de, University of Bremen and Alfred Wegener Institute). During this time, RV Polarstern expedition ARK XXIX/7 sampled in the pack-ice of the Weddell Sea from west to east approximately along the 60°S parallel. Daily sea-ice concentration data, from passive microwave-satellite measurements, were over 90 % during August–September along our cruise track, and decreased to approximately 50 % when sampling northward during the beginning of October. These values were in good agreement with the range of sea-ice coverage determined from SUIT sensors. Only at the last two stations (577 and 579), the SUIT sensor-derived ice coverage was about 95 %, whereas satellite-derived ice coverage, averaged over a 39 km2 area, was 50 %, placing these stations into the MIZ. In our ice thickness profiles, modal ice thicknesses ranged from 0.2 to 0.7 m, while on-board visual observations of snow depth during profiles ranged from 0.05 to 0.6 m. Snow depth and modal ice thickness values from our SUIT hauls were consistent with the general pattern observed during airborne-electromagnetic ice thickness surveys, and ground-based snow and ice surveys conducted in the vicinity of the sampling area during our cruise (Ricker and Krumpen unpublished data). Our snow depth and ice thickness values are also in agreement with previous winter measurements carried out in the Weddell Sea (Worby et al. 2008). Therefore, our local sampling profiles were representative of the regional-scale snow and sea-ice conditions.
Under-ice chlorophyll a concentrations were relatively low over the sampled area and were consistent with previous winter values reported for the Weddell Sea (Nöthig et al. 1991). We did, however, observe a steady increase in surface chlorophyll a concentrations at the northern five sampling locations, reaching a maximum concentration of 0.27 mg m−3 at the last station 579 (Table 1). This indicates that the productive season, in the northern part of the research area, had commenced by the end of September. At the last two stations 577 and 579, the lower satellite-derived ice coverage observations indicated an advanced state of melting, which was also evident by our observed lower surface salinities. Besides advanced melting and a deteriorating sea-ice habitat with seasonal progression at the end of the survey, the observed variability of the sea-ice habitat properties remains difficult to explain on a rather small dataset covering such a vast area. The stations sampled during the first half of our cruise did not show any seasonal or regional patterns in sea-ice properties. Local differences in sea-ice properties may have a background in atmospheric anomalies, wind patterns and occasional storm events (Holland and Kwok 2012; Kohout et al. 2014). Changes in surface-water temperature and salinity were rather small, likely due to the influence a quasi-homogeneous Winter Water layer circulated within the Weddell Gyre (Nöthig et al. 1991).
Species diversity and sampling performance
We identified at least 45 species within the under-ice community sampled, i.e. in the upper 2 m of the ice-covered water column. In terms of species richness, copepods dominated the community with 12 species, followed by amphipods with 7 species (Table 2). Our diversity was lower than in epipelagic fauna from the Weddell Sea (Hopkins and Torres 1988; Siegel et al. 1992; Fisher et al. 2004). However, a comparison to previous sampling of the epipelagic layer is complicated by differences in net type, mesh sizes, sampling depth interval, season and sample size. Most Antarctic zooplankton studies integrated the epipelagic community over at least the upper 50 m (Hopkins and Torres 1988; Lancraft et al. 1991; Siegel et al. 1992). The species composition from those studies is thus much more influenced by pelagic fauna, often dominated by the deeper-dwelling copepods (Razouls et al. 2000; Schnack-Schiel et al. 2008b). Moreover, larger sample size in previous studies could have accounted for higher diversity due to increased sampling effort. Siegel et al. (1992), with a sampling size double the size compared to our study, found that epipelagic richness and diversity in the northern Weddell Sea were highly variable horizontally, and were lower directly under the pack-ice than in the underlying water column. In the western Weddell Sea, diversity was shown to increase with depth (Hopkins and Torres 1988). When the sampled depth range of the SUIT is taken into account (1 % of the 200 m epipelagic depth stratum), however, our species richness is surprisingly high. This agrees with previous studies from the Lazarev Sea that found the diversity in the under-ice surface layer does not decrease much during winter because only few species migrate to greater depths and some even exhibit a hibernal upward migration (Flores et al. 2011, 2014). Our overall species richness was slightly higher, with 8 species more than reported in these winter studies from the Lazarev Sea, even when excluding the copepods and ostracods, which were not representatively sampled by Flores et al. (2011, 2014). A notable difference in the under-ice community of the two regions, however, was the extremely low numbers of post-larval Antarctic krill and the absence of fish larvae and cephalopods under the pack-ice in the northern Weddell Sea compared to the Lazarev Sea. This difference could be due to regional, but interannual variability cannot be excluded.
Sampling the sea-ice underside with the SUIT, over an average profile distance of 1.5 km, results in an increased sampling effort per station compared to other methods such as under-ice pumps, hand nets, or remotely operated vehicles (Brierley and Thomas 2002). This allowed us to capture the larger spatial variability of fauna with a patchy distribution (Schnack-Schiel 2003). Behavioural avoidance of the net by macrofauna cannot be excluded, but footage from the video camera mounted in the SUIT frame showed no visible avoidance. A potential underestimation of species, which are protected by the sea-ice underside topography, however, is difficult to assess with certainty. Due to known diel patterns (Siegel 2005; Flores et al. 2012), the abundance of some species, e.g. E. superba, C. propinquus, may have been underestimated at our daytime stations 555, 565, 571, and 577. At these stations, however, the abundances of E. superba and C. propinquus were well within the range of the other night time stations, and no significant diel effect was found (Wilcoxon test: E. superba p = 0.92; C. propinquus p = 0.79). This indicates that the general variability of species abundances was similar or larger compared to diel variability within our small dataset.
Under-ice community structure
In terms of species presence, we found a similar under-ice community composition over the sampling area, largely resembling the Weddell Sea ice-covered surface community dominated by sympagic and pelagic copepods, and larger grazers, such as euphausiids and amphipods (Schnack-Schiel 2003). Differences in community structure between sampling locations were largely determined by the variability in species abundances and biomass, rather than in the variability of species composition.
Copepods
Copepods numerically dominated (67 %) the under-ice community. The dominant species in our samples, S. longipes, is ubiquitous under the Weddell Sea pack-ice (Schnack-Schiel et al. 2001b, 2008a; Kiko et al. 2008) and has a life cycle strongly associated with the seasonal fluctuations of sea-ice (Kurbjeweit et al. 1993). Our abundances were lower than those found in the western Weddell Sea, during spring and summer (Schnack-Schiel et al. 2001b; Kiko et al. 2008), likely due to the inclusion of smaller stages in the abundance calculations by these authors. Ctenocalanus spp. was the second most abundant genus in our samples. This small calanoid is abundant in the epipelagic layer (Schnack-Schiel et al. 2008b). Its population structure in the surface layer is female-dominated during late winter/early spring (Schnack-Schiel and Mizdalski 1994), which agrees with our findings. The cyclopoid Pseudocyclopina sp. and the harpacticoid Idomene sp. are inhabitants of sea ice, ubiquitous in the western Weddell Sea (Menshenina and Melnikov 1995; Schnack-Schiel et al. 2008a) and eastern Weddell Sea (Schnack-Schiel et al. 1995). These species appeared frequently in our catches, yet with generally low under-ice abundances, except at the two most western stations 551 and 555. Due to their small size compared to the mesh size used, however, an underestimation of our sampling was likely. One of the dominant pelagic copepods in the Weddell Sea, C. propinquus, had much lower abundances under ice than previously reported in epipelagic studies (Hopkins and Torres 1988; Schnack-Schiel and Hagen 1994; Siegel et al. 1992). This species was described to remain in the upper 200 m during winter (Hopkins and Torres 1988; Schnack-Schiel and Hagen 1995) and actively feed (Pasternak and Schnack-Schiel 2001), and demonstrated the ability to switch to an omnivorous diet (Metz and Schnack-Schiel 1995).
Antarctic krill
Antarctic krill numerically dominated the species composition at the three northernmost stations 571, 577, and 579 (Fig. 2). Age class 0 krill dominated the population structure in this study (Schaafsma et al. 2016). Adult krill numbers were very low, while dominance of sub-adult krill within the Antarctic krill population was restricted to the westernmost station 551, where krill heavily dominated the cumulative biomass composition (95 %). Our results agree with previous late winter/early spring studies from the Scotia/Weddell Sea and Antarctic Peninsula regions, which also found furcilia VI to be the dominant stage in the under-ice layer (Daly 1990, 2004). A winter study from the Lazarev Sea often found higher abundances of sub-adult krill under ice than in the epipelagic layer (0–200 m depth layer), highlighting the pivotal role of sea ice in the functional ecology of other development stages of this species, besides larvae (Flores et al. 2012). In addition, rectangular midwater trawls (RMT) conducted in the 0–500 m depth layer near the SUIT locations showed that abundances of (sub-) adult krill were very low (Schaafsma et al. 2016). This indicates that (sub-) adult krill in the study region were in general low in abundance or too patchily distributed to be representatively sampled with the small sample size of this study. In larval and juvenile Antarctic krill, a comparison of our catches with the RMT krill catches from the 0–500 m layer showed that volumetric densities were higher in the under-ice layer than in the 0–500 m depth layer, but areal densities indicated that large parts of the juvenile population dwelled in the under-ice layer (Schaafsma et al. 2016).
Amphipods, chaetognaths, and pteropods
Other taxonomic groups, e.g. amphipods, chaetognaths, pteropods, occurred in lower abundances but nevertheless contributed significantly to the total biomass, mainly at stations 560, 562, 565, and 567 in the central part of the survey area. The ice-associated amphipod E. laticarpus occurred at stations with higher ice coverage and thicker ice (Table 1, Online Resource 1). E. laticarpus was distributed everywhere over the sampling area and was the most abundant amphipod in our samples. Their mean abundance of 1.48 ind. 100 m−2, however, was about half the winter under-ice abundance found in the Lazarev Sea, where, similarly to our study, the under-ice fauna was sampled in the upper two metres of the water column with the SUIT (Flores et al. 2011).
The chaetognaths E. hamata and Sagitta gazellae are known predators of copepods and Antarctic krill larvae (Giesecke and González 2012). E. hamata feeds year-round, mainly on copepods (Kruse et al. 2010), while Sagitta spp. feeds on krill larvae during winter (Lancraft et al. 1991). Higher chaetognaths abundances observed at stations with higher copepod abundances and krill larvae indicates a potential behavioural predator response of chaetognaths, such that they may have followed the prey distribution. When copepods were attracted to the under-ice resources during winter, chaetognaths were likely attracted by increased prey abundance under the ice. Similarly, the simultaneous migration of chaetognaths and copepods to deep water at the beginning of the productive season was suggested by our data and also confirms predatory behaviour by the chaetognaths.
Pteropods have been reported to numerically account for up to 35 % of the Southern Ocean zooplankton community (Hunt et al. 2008). Two of the major contributors, the thecosome L. helicina, and its allegedly monophagous predator, the gymnosome C. limacina (Hunt et al. 2008), were observed at less than half of our sampling locations. The low abundances found during our study (Table 2) agree with the winter under-ice abundances reported from the Lazarev Sea (Flores et al. 2011). Flores et al. (2011) suggested an association of C. limacina with the ice–water interface during winter, which were likely attracted to the under-ice environment by a predatory response to the presence of their prey L. helicina, at the sea-ice underside. The abundances of L. helicina in this study, however, were probably too low to serve as a sufficient food source for C. limacina.
Community structure associated with sea-ice habitat properties
Our hypothesis that the largely uniform environment of the northern Weddell Gyre was mirrored by a uniform under-ice community was not confirmed. When overall abundance and biomass distribution at our stations were considered, three community types were observed, characterised by gradual changes in the relative abundances and biomass contributions of species (Figs. 3, 4, Table 5). The krill-dominated community was characterised by higher overall biomass with Antarctic krill being the major contributor. There were, however, notable differences in the Antarctic krill composition at these stations (Online resource 3). At the westernmost station, 551 the biomass was dominated by sub-adults, while at stations 555 and 557 the biomass was dominated by age class 0 juveniles. Excluding post-larval krill, krill larvae-dominated and copepod-dominated communities had similar biomass over the sampling area.
The copepod-dominated community was characterised by higher abundances dominated by copepods. On the NMDS plot the copepod-dominated community was associated with the dominant species of the under-ice community, e.g. S. longipes, C. propinquus, and krill larvae. One evident characteristic of this community was not only the dominance of copepods (in abundance), but also the high contribution of other taxonomic groups, e.g. amphipods, pteropods, chaetognaths, and ctenophores (in biomass), which is an indication of a heterotrophic food web with filter feeders (L. helicina, appendicularians) as herbivores. Ammonium concentrations at the surface were higher at the copepod-dominated community sampling locations (0.4–1.1 μmol l−1) than the rest of our locations (C. Klaas, unpublished data.), supporting the assumption of extensive heterotrophic activity.
At the four northernmost stations, which were characterised as low biomass/abundances communities, the sea ice was in an advanced state of melt. The decrease in surface salinities below typical Winter Water values (<34.4) (Krell et al. 2005) suggests melting had already started at the time of our sampling. Copepod abundances were low, while some other species, e.g. L. helicina, C. limacina, were absent from our samples (Online resource 1). This could be due to a combined effect of the seasonal evolution of sea-ice habitats by the end of winter, inducing a behavioural response of some species performing vertical migration (Schnack-Schiel and Hagen 1995; Schnack-Schiel et al. 1995), closeness to the MIZ and the geographic location of these stations at the eastern side of the South Sandwich Islands. At the time of our sampling, the satellite-derived sea-ice coverage decreased to about 50 %, and only the thicker and rougher ice remained. The MIZ is typically more productive than other ice-covered areas (Brierley and Thomas 2002), which is in part a contradiction to our low biomass under-ice catches at the MIZ stations. On the NMDS map, the cluster of low biomass/abundances community stations was associated with a more pelagic assemblage, e.g. S. thompsoni, T. macrura, T. carpenteri, indicating a potential transition away from an ice-associated community. Moreover, the shallower bathymetry surrounding the islands, where these stations were located, would have also been expected to provide the increased productivity that is typical of shelf and slope areas. Increased productivity in the water column could have caused pelagic species to migrate into more productive deeper water layers. With such small sample size, it remains difficult to differentiate the seasonal effect, i.e. retreat of sea ice, from local effects, i.e. proximity of islands, at smaller scales. Nonetheless, the above mentioned contradictions would rather indicate that reduction of the sea-ice coverage induced an immediate response in the surface community structure.
In the BioEnv analysis, the combinations of environmental variables, which resulted in the three best correlations with the species abundance matrix, contained satellite-derived sea-ice coverage and snow depth, indicating that the community composition responds to both regional and local properties of sea-ice habitats. Broad scale hydrography and bathymetry played an equally important role, as was evident from the occurrence of temperature, salinity, and water depth as selected variables among the eight best BioEnv model combinations. Using only coarse descriptors of sea-ice properties such as visually observed sea-ice coverage and floe size, Flores et al. (2014) could not identify a correlation between community structure and sea-ice properties during winter. Using sensor-derived data, our study gives a first insight, albeit limited by a small sample size, on the relationships between sea-ice properties and under-ice community structure. This demonstrates the potential of this approach for larger sample sizes.
Conclusion
Distinct patterns in under-ice community structure in a physically largely uniform environment show that under-ice fauna distribution is very heterogeneous in space and time, making generalisations difficult. This complexity is relevant to ecological modelling of sea-ice systems. Drivers of these patterns may be controlled temporally, e.g. by advection of water masses and sea ice drift, or spatially, e.g. by biogeographical structures. To understand which species will be the winners and which will be losers in a changing ice-covered environment, extended observations are needed for future predictions of such a complex system. As the climate continues to warm, it is prudent to understand the ecological relationships between sea-ice-dependent Antarctic krill and Antarctic krill predators, as well as the interactions among sea-ice-dependent species that may be forced into competition for a shared food resource. Therefore, besides species distribution our focus should extend to quantifying food web energy fluxes, which would provide a broader view of the changing system, ultimately reflected in carbon cycle alterations.
References
Ainley DG, Dugger KM, Toniolo V, Gaffney I (2007) Cetacean occurrence patterns in the Amundsen and southern Bellingshausen Sea sector, Southern Ocean. Mar Mamm Sci 23:287–305
Ainley D, Jongsomjit D, Ballard G, Thiele D, Fraser W, Tynan C (2012) Modeling the relationship of Antarctic minke whales to major ocean boundaries. Polar Biol 35:281–290. doi:10.1007/s00300-011-1075-1
Arrigo KR, Worthen DL, Lizotte MP, Dixon P, Dieckmann G (1997) Primary production in antarctic Sea Ice. Science 276:394–397. doi:10.1126/science.276.5311.394
Arrigo KR, van Dijken GL, Bushinsky S (2008) Primary production in the Southern Ocean, 1997–2006. J Geophys Res-Oceans. doi:10.1029/2007jc004551
Atkinson A, Meyer B, Bathmann U, Stübing D, Hagen W, Schmidt K (2002) Feeding and energy budget of Antarctic krill Euphausia superba at the onset of winter-II Juveniles and adults. Limnol Oceanogr 47:953–966
Atkinson A et al (2008) Oceanic circumpolar habitats of antarctic krill. Mar Ecol Prog Ser 362:1–23
Bray JR, Curtis JT (1957) An ordination of the upland forest communities of southern Wisconsin. Ecol Monogr 27:325–349
Brierley AS, Thomas DN (2002) Ecology of Southern Ocean pack ice. Adv Marine Biol 43:171–276. doi:10.1016/S0065-2881(02)43005-2
Clarke K, Ainsworth M (1993) A method of linking multivariate community structure to environmental variables. Mar Ecol Prog Ser 92:205
Clarke KR, Warwick RM (2001) Change in marine communities: an approach to statistical analysis and interpretation. PRIMER-E Limited, Plymouth
Daly KL (1990) Overwintering development, growth, and feeding of larval Euphausia superba in the Antarctic marginal ice zone. Limnol Oceanogr 35:1564–1576
Daly KL (2004) Overwintering growth and development of larval Euphausia superba: an interannual comparison under varying environmental conditions west of the Antarctic Peninsula. Deep-Sea Res Pt II 51:2139–2168
David C, Lange B, Rabe B, Flores H (2015) Community structure of under-ice fauna in the Eurasian central Arctic Ocean in relation to environmental properties of sea ice habitats. Mar Ecol Prog Ser 522:15–32. doi:10.3354/meps11156
De Broyer C, Koubbi P, Griffiths H, Raymond B, Udekem d’Acoz C d’, Van de Putte A, Danis B, David B, Grant S, Gutt J, Held C, Hosie G, Huettmann F, Post A, Ropert-Coudert Y (2014) Biogeographic Atlas of the Southern Ocean. Scientific Committee on Antarctic Research, Cambridge, UK
Fisher EC, Kaufmann RS, Smith KL (2004) Variability of epipelagic macrozooplankton/micronekton community structure in the NW Weddell Sea, Antarctica (1995–1996). Mar Biol 144:345–360
Flores H et al (2011) Macrofauna under sea ice and in the open surface layer of the Lazarev Sea, Southern Ocean. Deep-Sea Res Pt II 58:1948–1961
Flores H et al (2012) The association of Antarctic krill Euphausia superba with the under-ice habitat. PLoS ONE. doi:10.1371/journal.pone.0031775
Flores H et al (2014) Seasonal changes in the vertical distribution and community structure of Antarctic macrozooplankton and micronekton. Deep-Sea Res Pt I 84:127–141. doi:10.1016/j.dsr.2013.11.001
Gannefors C et al (2005) The Arctic sea butterfly Limacina helicina: lipids and life strategy. Mar Biol 147:169–177
Giesecke R, González HE (2012) Distribution and feeding of chaetognaths in the epipelagic zone of the Lazarev Sea (Antarctica) during austral summer. Polar Biol 35:689–703. doi:10.1007/s00300-011-1114-y
Gloersen P, Campbell WJ (1991) Recent variations in Arctic and Antarctic sea-ice covers. Nature 352:33–36
Holland PR, Kwok R (2012) Wind-driven trends in Antarctic sea-ice drift. Nature Geosci 5:872–875. doi:10.1038/ngeo1627
Hopkins T, Torres J (1988) The zooplankton community in the vicinity of the ice edge, western Weddell Sea, March 1986. Polar Biol 9:79–87
Hopkins T, Torres J (1989) Midwater food web in the vicinity of a marginal ice zone in the western Weddell Sea. Deep Sea Res Pt I 36:543–560
Hopkins TL, Lancraft TM, Torres JJ, Donnelly J (1993) Community structure and trophic ecology of zooplankton in the scotia sea marginal ice zone in winter (1988). Deep Sea Res Pt I 40:81–105. doi:10.1016/0967-0637(93)90054-7
Hunt B, Pakhomov E, Hosie G, Siegel V, Ward P, Bernard K (2008) Pteropods in southern Ocean ecosystems. Prog Oceanogr 78:193–221
Hunt BPV, Pakhomov EA, Siegel V, Strass V, Cisewski B, Bathmann U (2011) The seasonal cycle of the Lazarev Sea macrozooplankton community and a potential shift to top-down trophic control in winter. Deep-Sea Res Pt II 58:1662–1676. doi:10.1016/j.dsr2.2010.11.016
Kiko R, Michels J, Mizdalski E, Schnack-Schiel SB, Werner I (2008) Living conditions, abundance and composition of the metazoan fauna in surface and sub-ice layers in pack ice of the western Weddell Sea during late spring. Deep-Sea Res Pt II 55:1000–1014. doi:10.1016/j.dsr2.2007.12.012
Kohout AL, Williams MJM, Dean SM, Meylan MH (2014) Storm-induced sea-ice breakup and the implications for ice extent. Nature 509:604–607. doi:10.1038/nature13262
Krell A, Schnack-Schiel SB, Thomas DN, Kattner G, Zipan W, Dieckmann GS (2005) Phytoplankton dynamics in relation to hydrography, nutrients and zooplankton at the onset of sea ice formation in the eastern Weddell Sea (Antarctica). Polar Biol 28:700–713
Kruse S, Hagen W, Bathmann U (2010) Feeding ecology and energetics of the Antarctic chaetognaths Eukrohnia hamata, E. bathypelagica and E. bathyantarctica. Mar Biol 157:2289–2302
Kruskal JB (1964) Nonmetric multidimensional scaling: a numerical method. Psychometrika 29:115–129. doi:10.1007/bf02289694
Kurbjeweit F, Gradinger R, Weissenberger J (1993) The life cycle of Stephos longipes-an example for cryopelagic coupling in the Weddell Sea (Antarctica). Mar Ecol Prog Ser 98:255–262
Lancraft TM, Hopkins TL, Torres JJ, Donnelly J (1991) Oceanic micronektonic/macrozooplanktonic community structure and feeding in ice covered Antarctic waters during the winter (AMERIEZ 1988). Polar Biol 11:157–167
Legendre P, Legendre L (2012) Numerical ecology. Elsevier, Oxford, UK
Lizotte MP (2001) The contributions of Sea Ice Algae to Antarctic marine primary production. Am Zool 41:57–73. doi:10.1093/icb/41.1.57
Loeb V, Siegel V, Holm-Hansen O, Hewitt R, Fraser W, Trivelpiece W, Trivelpiece S (1997) Effects of sea-ice extent and krill or salp dominance on the Antarctic food web. Nature 387:897–900
Mantel N (1967) The detection of disease clustering and a generalized regression approach. Cancer Res 27:209–220
Menshenina LL, Melnikov IA (1995) Under-ice zooplankton of the western Weddell Sea. Proc NIPR Symp Polar Biol 8:126–138
Metz C, Schnack-Schiel S (1995) Observations on carnivorous feeding in Antarctic calanoid copepods. Mar Ecol Prog Ser 129:71–75
Meyer B (2012) The overwintering of Antarctic krill, Euphausia superba, from an ecophysiological perspective. Polar Biol 35:15–37. doi:10.1007/s00300-011-1120-0
Minchin PR (1987) An evaluation of the relative robustness of techniques for ecological ordination. In: Prentice IC, van der Maarel E (eds) Theory and models in vegetation science. Springer, Berlin, pp 89–107
Mizdalski E (1988) Weight and length data of zooplankton in the Weddell Sea in austral spring 1986 (ANT V/3). Berichte zur Polarforschung (Reports on Polar Research) 55
Motoda S (1959) Devices of simple plankton apparatus. Memoirs of the Faculty of Fisheries, Hokkaido University 7:73–94
Nöthig EM, Bathmann U, Jennings JC Jr, Fahrbach E, Gradinger R, Gordon LI, Makarov R (1991) Regional relationships between biological and hydrographical properties in the Weddell Gyre in late austral winter 1989. Mar Chem 35:325–336. doi:10.1016/S0304-4203(09)90025-1
O’Brien D (1987) Direct observations of the behavior of Euphausia superba and Euphausia crystallorophias (Crustacea: euphausiacea) under pack ice during the Antarctic spring of 1985. J Crustacean Biol 7:437–448
Pakhomov EA, Froneman PW (2004) Zooplankton dynamics in the eastern Atlantic sector of the Southern Ocean during the austral summer 1997/1998—Part 1: community structure. Deep-Sea Res Pt II 51:2599–2616. doi:10.1016/j.dsr2.2000.11.001
Pasternak AF, Schnack-Schiel SB (2001) Seasonal feeding patterns of the dominant Antarctic copepods Calanus propinquus and Calanoides acutus in the Weddell Sea. Polar Biol 24:771–784
Quetin LB, Ross RM, Frazer TK, Haberman KL (2013) Factors affecting distribution and abundance of zooplankton, with an emphasis on Antarctic krill, Euphausia superba. Antarct Res Series 70:357–371. doi:10.1029/AR070p0357
R Core Team (2015) R: A language and environment for statistical computing. R Foundation for Statistical Computing, Vienna, Austria
Razouls S, Razouls C, De Bovée F (2000) Biodiversity and biogeography of Antarctic copepods. Antarct Sci 12:343–362
Schaafsma FL, David C, Pakhomov EA, Hunt BPV, Lange BA, Flores H, van Franeker JA (2016) Size and stage composition of age class 0 Antarctic krill (Euphausia superba) in the ice-water interface layer during winter/early spring. Polar Biol. doi:10.1007/s00300-015-1877-7
Schmidt K, Atkinson A, Pond DW, Ireland LC (2014) Feeding and overwintering of Antarctic krill across its major habitats: the role of sea ice cover, water depth, and phytoplankton abundance. Limnol Oceanogr 59:17–36
Schnack-Schiel SB (2003) The macrobiology of sea ice. In: Thomas DN, Dieckmann GS (eds) Sea ice: an introduction to its physics, chemistry, biology and geology. Blackwell Science Ltd., Oxford, UK
Schnack-Schiel SB, Hagen W (1994) Life cycle strategies and seasonal variations in distribution and population structure of four dominant calanoid copepod species in the eastern Weddell Sea, Antarctica. J Plankton Res 16:1543–1566. doi:10.1093/plankt/16.11.1543
Schnack-Schiel SB, Hagen W (1995) Life-cycle strategies of Calanoides acutus, Calanus propinquus, and Metridia gerlachei (Copepoda: Calanoida) in the eastern Weddell Sea, Antarctica. ICES J Mar Sci 52:541–548. doi:10.1016/1054-3139(95)80068-9
Schnack-Schiel S, Mizdalski E (1994) Seasonal variations in distribution and population structure of Microcalanus pygmaeus and Ctenocalanus citer (Copepoda: Calanoida) in the eastern Weddell Sea, Antarctica. Mar Biol 119:357–366
Schnack-Schiel SB et al (1995) Life cycle strategy of the Antarctic calanoid copepod Stephos longipes. Prog Oceanogr 36:45–75. doi:10.1016/0079-6611(95)00014-3
Schnack-Schiel SB, Dieckmann GS, Gradinger R, Melnikov I, Spindler M, Thomas DN (2001a) Meiofauna in sea ice of the Weddell Sea (Antarctica). Polar Biol 24:724–728
Schnack-Schiel SB, Thomas DN, Haas C, Dieckmann GS, Alheit R (2001b) The occurrence of the copepods Stephos longipes (Calanoida) and Drescheriella glacialis (Harpacticoida) in summer sea ice in the Weddell Sea, Antarctica. Antarct Sci 13:150–157
Schnack-Schiel SB, Haas C, Michels J, Mizdalski E, Schünemann H, Steffens M, Thomas DN (2008a) Copepods in sea ice of the western Weddell Sea during austral spring 2004. Deep-Sea Res Pt II 55:1056–1067
Schnack-Schiel SB, Michels J, Mizdalski E, Schodlok MP, Schröder M (2008b) Composition and community structure of zooplankton in the sea ice-covered western Weddell Sea in spring 2004-with emphasis on calanoid copepods. Deep-Sea Res Pt II 55:1040–1055
Shannon C (1948) A mathematical theory of communication. Bell Sys Tech J 27:379–423
Siegel V (2005) Distribution and population dynamics of Euphausia superba: summary of recent findings. Polar Biol 29:1–22
Siegel V, Loeb V (1995) Recruitment of Antarctic krill Euphausia superba and possible causes for its variability. Mar Ecol Prog Ser 123:45–56
Siegel V, Skibowski A, Harm U (1992) Community structure of the epipelagic zooplankton community under the sea-ice of the northern Weddell Sea. Polar Biol 12:15–24
Spreen G, Kaleschke L, Heygster G (2008) Sea ice remote sensing using AMSR-E 89-GHz channels. J Geophys Res-Oceans 113:C02S03. doi:10.1029/2005jc003384
Thomas D, Dieckmann G (2002) Antarctic sea ice-a habitat for extremophiles. Science 295:641–644
Turner J, Hosking JS, Phillips T, Marshall GJ (2013) Temporal and spatial evolution of the Antarctic sea ice prior to the September 2012 record maximum extent. Geophys Res Lett 40:5494–5498. doi:10.1002/2013gl058371
van Franeker JA, Bathmann UV, Mathot S (1997) Carbon fluxes to Antarctic top predators. Deep-Sea Res Pt Ii 44:435–455. doi:10.1016/S0967-0645(96)00078-1
van Franeker JA, Flores H, Van Dorssen M (2009) Surface and Under Ice Trawl (SUIT). In: Flores H (author) Frozen desert alive -the role of sea ice for pelagic macrofauna and its predators: implications for the Antarctic pack-ice food web. Dissertation, University of Groningen, Groningen, Netherlands
White MG, Piatkowski U (1993) Abundance, horizontal and vertical distribution of fish in eastern Weddell Sea micronekton. Polar Biol 13:41–53
Wilcoxon F (1945) Individual comparisons by ranking methods. Biometrics Bull 1:80–83
Worby AP, Geiger CA, Paget MJ, Van Woert ML, Ackley SF, DeLiberty TL (2008) Thickness distribution of Antarctic sea ice. J Geophys Res-Oceans 113:C05S92. doi:10.1029/2007jc004254
Yang G, Li C, Sun S (2011) Inter-annual variation in summer zooplankton community structure in Prydz Bay, Antarctica, from 1999 to 2006. Polar Biol 34:921–932. doi:10.1007/s00300-010-0948-z
Zwally HJ, Comiso JC, Parkinson CL, Cavalieri DJ, Gloersen P (2002) Variability of Antarctic sea ice 1979–1998. J Geophys Res-Oceans 107:9-1–9-19. doi:10.1029/2000jc000733
Acknowledgments
We thank Captain Stephan Schwarze and the crew of RV Polarstern expedition ANT XXIX/7 for their excellent support with work at sea. We thank Michiel van Dorssen for operational and technical support with the Surface and Under-Ice Trawl (SUIT). SUIT was developed by IMARES with support from the Netherlands Ministry of EZ (Project WOT-04-009-036) and the Netherlands Polar Program (Projects ALW 851.20.011 and 866.13.009). This study is part of the Helmholtz Association Young Investigators Group Iceflux: Ice-ecosystem carbon flux in polar oceans (VH-NG-800). We thank Dr. Astrid Cornils for help with copepod species identification. We thank Dr. Christine Klaas for providing chlorophyll a measurements of water samples used for calibration.
Author information
Authors and Affiliations
Corresponding author
Electronic supplementary material
Below is the link to the electronic supplementary material.
Rights and permissions
About this article
Cite this article
David, C., Schaafsma, F.L., van Franeker, J.A. et al. Community structure of under-ice fauna in relation to winter sea-ice habitat properties from the Weddell Sea. Polar Biol 40, 247–261 (2017). https://doi.org/10.1007/s00300-016-1948-4
Received:
Revised:
Accepted:
Published:
Issue Date:
DOI: https://doi.org/10.1007/s00300-016-1948-4