Abstract
Soil salinity is a serious threat to sustainable agriculture, and a number of research are going on to improve saline-resistant crops by using various breeding methods and genetic engineering tools. These methods are time-consuming, often face yield penalties, and many other ethical issues. There is a need to explore other more stable, environmentally friendly methods for the sustainable agriculture. Exploration of plant growth-promoting rhizobacteria (PGPR) associated with salt-tolerant plants (halophytes) and their use as probiotics for saline soil agriculture are a promising substitute for classical approaches. Salinity is one of the major abiotic stress reported from arid and semiarid regions which causes a major loss in the agriculture productivity. Halophytes are adapted to the saline environment because of their genetic makeup and associated microbiome. These microbiomes have potential to survive in the saline condition, but they are not thoroughly explored. Several studies showed that bacteria associated with halophytes, directly and indirectly, support the plant growth and yield in saline conditions; thus, these bacteria can be used as probiotics for salt-sensitive plants (glycophytes) grown in the salt-affected area to enhance the productivity. PGPR induce many morphological, physiological, and genetic changes in a plant which compensate the pressure of salt stress. The genetic level changes in plants due to application or the presence of PGPR are known as induced systemic resistance (ISR). PGPR secrete some beneficial elements like organic solutes, siderophores, etc. to survive in harsh conditions. PGPR also help plants to maintain their osmotic pressure and nutrient balance. The presence of PGPR also affects the level of various phytohormones in plants which play a major role in growth, development, and stress response of the plant.
Access provided by Autonomous University of Puebla. Download chapter PDF
Similar content being viewed by others
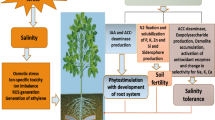
Keywords
3.1 Introduction
As human probiotics, plant-probiotic microorganisms (PPM) are beneficial microorganisms which can act as bio-protectants (reduce pathogen infection), bio-fertilizers (increase yield), and biostimulants, additionally also reduce various biotic and abiotic plant stresses (de Souza Vandenberghe et al. 2017). According to FAO/WHO expert consultation report, probiotics are “live microorganisms which when administered in adequate amounts confer a health benefit on the host” (Hill et al. 2014).
Plant growth is affected by various abiotic factors like abundance of heavy metals, soil toxicity, salinity, cold, drought, temperature and flood. Among these, soil salinity is a major cause which adversely affects the plant growth and productivity (Príncipe et al. 2007). The soil is considered saline when electrical conductivity (EC) of the saturation extract of the rhizosphere soil (root zone) exceeds 4 dS m−1 which is equivalent to approximately 40 mM NaCl at 25 °C; commonly, a higher EC limits the crop productivity (Munns and Tester 2008). Primary salinization is caused by the salts that are already present in the soil, and secondary salinization is a result of excessive use of chemical fertilizers, soil amendments, and inadequate irrigation management (Carillo et al. 2011). According to reports of FAO (2016), 20% of total agriculture land has been severely affected by excessive soil salinity.
Soil salinity adversely affects the plant health at a physiological, biochemical, and molecular level which decreases the growth and yield of crop plants (Roy et al. 2014). The development of salt stress-resistant transgenic plants is considered as an option for saline soil agriculture; however, there is a need for environmentally friendly, ethical, and natural procedure for sustainable agriculture (Ashraf 2009). Halophytic plant species are adapted to saline soils, naturally. These plants can survive in this harsh environment by their specific genetic makeup and their root-associated microbiome having very diverse rhizobacteria (Mishra and Tanna 2017; Mukhtar et al. 2017). Rhizobacteria isolated from microbiome of halophytes may help the glycophytic plant to grow in saline soil and enhance the productivity of glycophytic crop plants. These rhizobacteria can be used for the improvement of the soil quality.
3.2 Effect of Salt on Plant Growth
Plant growth is affected by salt at multiple levels. The primary effect of soil salinity on plant growth and productivity is briefly discussed as follows:
3.2.1 Osmotic Stress
During stress, water intake by root is very difficult which results in reduced transpiration rate and leaf expansion, stomatal closure, water retention, water use efficiency, and increased rate of senescence of older leaves (Munns and Tester 2008). Osmotic stress also leads to the production of reactive oxygen species (ROS) which results in autophagy (Han et al. 2011).
3.2.2 Nutrient Imbalance
Soil salinity profoundly influences the nutrient imbalance and causes nutrients deficiency in plants (Blaylock 1994). In saline soil, it is difficult to take phosphorus (P) because phosphate ions precipitate with Ca ions and become unavailable for plant utilization (Bano and Fatima 2009).
3.2.3 Decrease in Photosynthesis
Salt stress damages the photosynthetic capability of the plant. During salt stress, growth of the plant is affected, and it also causes a reduction in leaf area, degradation of chlorophyll and carotene content, low level of stomatal conductance, and harmful effects on PSII and PSI which altogether result in the decrease of photosynthesis ability of plant (Netondo et al. 2004).
3.2.4 Effect on Reproductivity
Salinity badly disturbs reproductive machinery of the affected plant. Salt stress inhibits microsporogenesis (formation of microspores), floral development, ovule abortion, embryo arrest, and senescence of fertilized embryos, but induces apoptosis (programmed cell death) in some reproductive tissues (Shrivastava and Kumar 2015).
3.2.5 Ion Toxicity
Ion toxicity is the major problem of soil salinity. During salinity, excess sodium ion causes leaf burn and defoliation (Podmore 2009). Chloride toxicity causes leaf bronzing and necrotic spots in plant species (Rahnama et al. 2010). A high level of Cl− disturbs NO3 − uptake in leaves which results in the decrease of nitrate reductase (NR) activity, affecting the nitrogen assimilation in plants (Baki et al. 2000). Excessive salt causes replacement of K+ by Na+ which promotes conformational changes in many proteins and enzymes, and these affect many metabolic and molecular alterations in plants (Zhu 2002; Chinnusamy et al. 2006).
3.2.6 Oxidative Stress
Salinity induces the generation of reactive oxygen species (ROS) and free radicals which causes oxidative stress and reduces the level of antioxidant enzymes such as catalase (CAT), superoxide dismutase (SOD), peroxidase (POX), glutathione (GSH), ascorbate peroxidase (APX), glutathione reductase (GR), glutathione-S-transferase (GST), and monodehydroascorbate reductase in plants (AbdElgawad et al. 2016). ROS are harmful to cell components and damage lipids, protein, and nucleic acids; furthermore, long-term exposure causes the death of host species (del Rio et al. 2003).
3.2.7 Production of Excess Ethylene
Plants, when subjected to salinity, produce excessive ethylene, which severely retards root development (Mahajan and Tuteja 2005). Under biotic and abiotic stress conditions, ethylene causes a decrease in root growth and senescence in crop plants (Ma et al. 2003). In general, salt stress adversely affects the cell cycle and differentiation by reducing the expression and activity of cyclins and cyclin-dependent kinases. Some reports also showed that salinity also reduces seed germination, seedling growth, and enzyme activity (Seckin et al. 2009), which ultimately affects plant yield.
3.3 Need for Plant Probiotic
For the sustainable agriculture toward biotic and abiotic stress, transgenic approaches are commonly used, but there are many shortcomings and limitation of transgenic plants. These approaches need a long time and need much manpower from lab to field and after that have less success rate (Coleman- Derr and Tringe 2014). Transgenic approaches caused unstable progeny due to the involvement of multiple genes in abiotic stress responses and also the uncertainty of gene flow in the next generation (Jewell et al. 2010). Transgenic crops are facing the difficulty of public acceptance as well as many ethical issues (Fedoroff 2010). Molecular techniques which are involved in the development of transgenic crops are most of the time not applicable or responsive in case of tetraploid and hexaploid species. Saline and alkaline stresses are typically linked in nature (Saslis-Lagoudakis et al. 2014), and the tolerance of transgenic toward high soil alkalinity (pH) and/or salt alkaline-mixed conditions could not fully obtain (Yamaguchi and Blumwald 2005). Sometimes transgenic salt-tolerant crops, especially in which transcription factors have been genetically modified, resulting in the low yield of the plant (Roy et al. 2014). When a plant is facing salt stress, many genes, proteins, and metabolites are activated at the same time, but transgenic plants are concentrated on either only one gene or one promoter only, so protection against salt stress is not obtained completely or up to desired level (Bhatnagar-Mathur et al. 2008).
To overcome these problems and for the fulfillment of increasing food demand in an environment-friendly manner, there is a need of exploring more useful alternative strategies for sustainable agriculture. The health of the plant and its response toward any stress are affected by not only its genome but also microbiome of its surroundings (Munns and Gilliham 2015; Vannier et al. 2015). Plant root system releases a large number of nutrients, known as rhizodeposits (e.g., exudates, border cells, and mucilage). Rhizodeposits influence the rhizosphere by regulating the microbial diversity and activity on plant roots (Cook et al. 1995). The rhizosphere is a narrow zone of soil including surrounding plant roots and it can contain up to 1011 microbial cells per gram of the root (Egamberdieva 2008).
The microbes have coevolved with their corresponding hosts and adapted their community structure for specific environmental stresses (Lau and Lennon 2012). Thus, interacting network among root, soil, and microorganisms plays an imperative role in supporting healthy growth and defense mechanism under unfavorable conditions (biotic and abiotic stress) for both the host and its associated organisms. Some of these microbes support their host plant regarding growth, yield, in biotic and abiotic stress (Fig. 3.1). Due to plant growth-promoting traits of these bacteria, they are called plant growth-promoting bacteria (PGPB), and PGPB which are found in the rhizosphere and stimulate plant growth are called plant growth-promoting rhizobacteria (PGPR) (Kloepper and Schroth 1981). PGPR can be classified as extracellular and intracellular. Extracellular PGPR (ePGPR) exist in the rhizosphere, on the rhizoplane, in the spaces between root cortex cells, while intracellular PGPR (iPGPR) exist inside root cells, generally in specialized nodules (Gray and Smith 2005).
3.4 Rhizobacteria as Probiotic
The term induced systemic tolerance (IST) has been proposed for PGPR-induced physical and chemical changes that result in enhanced abiotic stress tolerance (Shrivastava and Kumar 2015). Halotolerant rhizobacteria have many characteristics which improve the plant health (Fig. 3.2), including nitrogen fixation, phosphate solubilization, production of phytohormones and exopolysaccharide, aminocyclopropane-1-carboxylate deaminase (ACCD) production, and biolytic enzyme secretion (de Souza et al. 2015).
3.4.1 ACCD Activity
Many halotolerant bacterial isolates showed the ACCD activity which required for plant health (Santoyo et al. 2016). Plants naturally select ACCD-producing bacteria under stress conditions. PGPR produce the enzyme ACC deaminase which cleaves the plant ethylene precursor ACC into ammonia and α-ketobutyrate and decrease the level of ethylene in seedlings and plants. Additionally, ACCD stimulates IAA production and thus enhances the growth and survival of plant under abiotic stress (Glick 2014; Mayak et al. 2004a, b).
3.4.2 Osmolyte Production
Osmolytes are highly soluble substances with no net charge at physiological pH, so they do not interact with proteins and compatible with many cellular functions such as DNA–protein interactions, protein–protein interactions, cellular metabolism, and osmotic balance (Lippert and Galinski 1992; Welsh 2000). The most studied osmolytes, produced and secreted by halotolerant PGPR, are trehalose, glucosylglycerol (GG), proline, glycine betaine, and choline (Qurashi and Sabri 2013). Trehalose is nonreducing disaccharide which is not common in vascular plants but acts as an osmoprotectant. It stabilizes dehydrated enzymes and membranes, thus protects them from desiccation. Trehalose metabolism is important for plant growth, and yield of leguminous plants under abiotic stress conditions (Suarez et al. 2008). Stenotrophomonas rhizophila is halotolerant rhizobacteria which can grow at 4% NaCl and produce the trehalose in response to salt stress (Roder et al. 2005).
3.4.3 Ion-Exclusion Mechanisms
Rhizobacteria can alter root uptake of toxic ions by slight changes in the physiology of host plant (by regulating ion transporters) and by modification in physical barriers around the roots (Siddikee et al. 2011) or by directly reducing accumulation of toxic ions (Na+ and Cl−). Rhizobacteria also improve the nutritional status of the plant by maintaining the essential level of both macro (N, P, and K) and micronutrients (Zn, Fe, Cu, and Mn). Zahir et al. (2009) showed higher K+/Na+ ratios in salinized crop plants like wheat after inoculation with Pseudomonas and Serratia sp.
3.4.4 Increment in Nutrients Accessibility
Phosphorus (P) is abundantly available in soils in both organic and inorganic form, but it is not easily available for plants because the majority of soil P is found in insoluble forms. Microbial-induced changes in rhizosphere pH (organic acid excretion) convert insoluble phosphorus in soil into the soluble state (El-Tarabily and Youssef 2010). In soil, iron occurs as Fe3+ and forms insoluble hydroxides which becomes inaccessible to plants. PGPR also secret some organic molecules called as siderophores. These siderophores help plants to take iron because they chelate Fe3+ (Rajkumar et al. 2010). It is shown that siderophores produced by Enterobacter sp. 638 and Pseudomonas sp. enhance the growth of poplar and tomato, respectively (Taghavi et al. 2009; Nishma et al. 2014).
3.4.5 N2 Fixation
Nitrogen fixation is the process of conversion of atmospheric N2 to NH3. Microorganisms involved in N2 fixation are rhizosphere associated either symbiotic or endophytic. Rhizosphere-associated bacteria use carbon compounds from root exudates for N2 fixation. All these microorganisms contain specific genes for N2 fixation, for example, a nifH gene that encodes for dinitrogenase reductase enzyme which converts N2 to NH3 and makes it available for plants (Raymond et al. 2004).
3.4.6 Production of Phytohormones
Rhizobacteria also produce a variety of phytohormones like cytokinin, indole-3-acetic acid (IAA), abscisic acid (ABA), and gibberellic acid (GA) (Glick 2012). Phytohormones produced by PGPR play an important role in increasing root surface area, and numbers of root tip in many plants, thus increasing the accessibility of plants to soil nutrients (Narula et al. 2006). Similarly, GA enhances the shoot growth and total biomass of the plant (Boiero et al. 2007). ABA regulates the stomatal activity of plants hence indirectly affects the photosynthesis response to the saline soil (Dodd 2003). Many PGPR, isolated from saline soil, produced ABA which can enhance the growth of plants under saline condition (Naz et al. 2009).
3.4.7 Biocontrol Agent
PGPR also secret some antibiotic compounds which play a major role in the reduction of phytopathogens and deleterious rhizobacteria (Stutz et al. 1986). By acting as a biocontrol agent, rhizobacteria stimulate plant growth indirectly. They synthesize extracellular enzymes to hydrolyze the cell wall of pathogens and compete with harmful bacteria for niches within the rhizosphere (Zahir et al. 2004; van Loon 2007). PGPR also activate induced systemic resistance (ISR) in host plant to fight with pathogens at the molecular level (Chandler et al. 2008).
3.5 Halotolerant Rhizobacteria
Bacteria which are associated with halophytic plants can tolerate salt level up to 4–30% (Yuan et al. 2016) and play an essential role in the survival of halophytes in the harsh environment and are called as halophyte rhizosphere-associated microbes (HRAMs). These bacteria are endowed with specific metabolic signals which are essential for plant’s survival under salt stress (Szymanska et al. 2013). The plant–microbes interaction effectively improves the soil quality and fertility (Yuan et al. 2016). HRAMs have all PGPR activity with high salt tolerance, so they are the most suitable candidate for growth and yield enhancement in glycophytic plants in salt-affected areas in most environmentally friendly manner.
3.6 Halotolerant Bacteria as Plant Probiotics for Saline Soil- Based Agriculture
Proper osmotic regulation is a crucial point for plants growing in the saline area; the absence of this results in loss of turgidity, cell dehydration, failure of photosynthetic machinery, nutritional disorders, toxicities, less crop productivity, and ultimately death of cells (Ashraf 2004; Shrivastava and Kumar 2015).
Halotolerant bacteria survive under salt stress conditions because they modify the osmolality of the rhizosphere (Egamberdieva 2011). These modified osmolytes are made available for utilization of plant and act as free radical scavengers, a regulator of the photosynthetic apparatus, and stabilizer of subcellular structures (Yang et al. 2009), hence affecting the plant growth (Miller and Wood 1996), so, they are considered as an effective candidate for plant growth promotion.
Previously, many studies showed that halotolerant PGPR are capable to ameliorate the detrimental effect of salinity on the growth of basil, canola, maize, and tomato plants (Kang et al. 2014; Rojas-Tapias et al. 2012). Karuppasamy et al. (2011) showed that the growth of tree legumes Samanea saman could be improved by the application of stress-tolerant rhizobia. Halotolerant bacterial consortia isolated from avocado trees could ameliorate salinity stress in other plant species such as wheat (Barra et al. 2016). Upadhyay et al. (2009) isolated 130 rhizobacterial strains from the rhizosphere of wheat plants grown in a saline zone and showed that out of 130, 24 were tolerant to relatively high levels (up to 8%) of NaCl. All of the 24 salt-tolerant isolates were able to produce IAA, 10 isolates solubilized phosphorus, eight isolates produced siderophores, 6 were involved in gibberellin production, and 2 isolates contained the nifH gene, which indicates that the whole consortia have PGPR ability.
In another study, Nadeem et al. (2010) evaluate a number of axenic cultures of rhizobacterial strains for their plant–growth potential under varying salinity stress conditions. At a high salinity level (15 dS m−1), the PGPR–inoculants improved overall plant growth including plant height, root length, plant biomass, and also grain yield by 37%, 70%, 116%, and 111%, respectively. It was also observed that treated plants also had higher K+/Na+ ratios, relative water, and chlorophyll contents along with lower proline contents. Potential halotolerant rhizobacteria reported for sustainable saline soil agriculture are listed in Table 3.1.
3.7 Conclusion
Halotolerant rhizobacteria-based agriculture is more efficient and environment-friendly than plant breeding and modification at the genetic level. The plant microbiome is considered as a pan–genome (second plant genome) having the capability to enhance host stress tolerance. It is well established that multiple plant growth-promoting traits are present in the bacterial communities of a halophytic rhizosphere. A number of halotolerant bacteria were isolated from the rhizosphere of halophytes, and their potential was studied to enhance plant growth under saline environments. Although single PGPR strain can enhance plant growth and tolerance to abiotic stress, several reports suggest that plants often supported by more than a single type of symbiotic microbe to combat salinity. A co-culture system containing two or more microbes is proven to be more efficient for the plant health. However, the use of PGPR in agriculture needs more extensive research and awareness. In addition to the study on plant physiology or microbiome of the host plant, it is also needed to concentrate on plant-microbe interaction to understand the whole mechanism during biotic and abiotic stress. Identification of the plant–microbes exudates, plant–microbes signals, and key players in the rhizosphere microbiome will provide unique metabolic and physiological markers which can elucidate the mechanism of plant–growth promotion by PGPR, additionally can also reveal whether and how plants recruit and stimulate beneficial (micro)organisms or its result of any stress.
References
AbdElgawad H, Zinta G, Hegab MM, Pandey R, Asard H, Abuelsoud W (2016) High salinity induces different oxidative stress and antioxidant responses in maize seedlings organs. Front Plant Sci 7:276
Abdelwahab RAI, Cherif A, Cristina C, Nabti E (2017) Extracts from seaweeds and Opuntia ficus-indica cladodes enhance diazotrophic-PGPR halotolerance, their enzymatic potential, and their impact on wheat germination under salt stress. Pedosphere
Ahmad M, Zahir ZA, Nazli F, Akram F, Arshad M, Khalid M (2013) Effectiveness of halo-tolerant, auxin producing Pseudomonas and Rhizobium strains to improve osmotic stress tolerance in mung bean (Vigna radiata L.). Braz J Microbiol 44(4):1341–1348
Ashraf M (2004) Some important physiological selection criteria for salt tolerance in plants. Flora-Morphology, Distrib, Funct Ecol Plants 199(5):361–376
Ashraf M (2009) Biotechnological approach of improving plant salt tolerance using antioxidants as markers. Biotechnol Adv 27(1):84–93
Baki GK, Siefritz F, Man HM, Weiner H, Kaldenhoff R, Kaiser WM (2000) Nitrate reductase in Zea mays L. under salinity. Plant Cell Environ 23(5):515–521
Bal HB, Nayak L, Das S, Adhya TK (2013) Isolation of ACC deaminase producing PGPR from rice rhizosphere and evaluating their plant growth promoting activity under salt stress. Plant and Soil:1–13
Bano A, Fatima M (2009) Salt tolerance in Zea mays (L). following inoculation with Rhizobium and Pseudomonas. Biol Fertil Soils 45(4):405–413
Barra PJ, Inostroza NG, Acuña JJ, Mora ML, Crowley DE, Jorquera MA (2016) Formulation of bacterial consortia from avocado (Persea americana Mill.) and their effect on growth, biomass and superoxide dismutase activity of wheat seedlings under salt stress. Appl Soil Ecol 102:80–91
Bashan Y, Moreno M, Troyo E (2000) Growth promotion of the seawater-irrigated oilseed halophyte Salicornia bigelovii inoculated with mangrove rhizosphere bacteria and halotolerant Azospirillum spp. Biol Fertil Soils 32(4):265–272
Bhatnagar-Mathur P, Vadez V, Sharma KK (2008) Transgenic approaches for abiotic stress tolerance in plants: retrospect and prospects. Plant Cell Rep 27(3):411–424
Blaylock AD (1994) Soil salinity, salt tolerance, and growth potential of horticultural and landscape plants. University of Wyoming, Cooperative Extension Service, Department of Plant, Soil, and Insect Sciences, College of Agriculture
Boiero L, Perrig D, Masciarelli O, Penna C, Cassán F, Luna V (2007) Phytohormone production by three strains of Bradyrhizobium japonicum and possible physiological and technological implications. Appl Microbiol Biotechnol 74(4):874–880
Carillo P, Annunziata MG, Pontecorvo G, Fuggi A, Woodrow P (2011) Salinity stress and salt tolerance. In: Abiotic stress in plants-mechanisms and adaptations. InTech
Chandler D, Davidson G, Grant W, Greaves J, Tatchell G (2008) Microbial biopesticides for integrated crop management: an assessment of environmental and regulatory sustainability. Trends Food Sci Technol 19(5):275–283
Chinnusamy V, Zhu J, Zhu JK (2006) Gene regulation during cold acclimation in plants. Physiol Plant 126(1):52–61
Coleman-Derr D, Tringe SG (2014) Building the crops of tomorrow: advantages of symbiont-based approaches to improving abiotic stress tolerance. Front Microbiol 5:283
Cook RJ, Thomashow LS, Weller DM, Fujimoto D, Mazzola M, Bangera G, Kim D-S (1995) Molecular mechanisms of defense by rhizobacteria against root disease. Proc Natl Acad Sci 92(10):4197–4201
de Souza R, Ambrosini A, Passaglia LM (2015) Plant growth-promoting bacteria as inoculants in agricultural soils. Genet Mol Biol 38(4):401–419
de Souza Vandenberghe LP, Garcia LMB, Rodrigues C, Camara MC, de Melo Pereira GV, de Oliveira J, Soccol CR (2017) Potential applications of plant probiotic microorganisms in agriculture and forestry. AIMS Microbiol 3(3):629–648
Del Río LA, Corpas FJ, Sandalio LM, Palma JM, Barroso JB (2003) Plant peroxisomes, reactive oxygen metabolism and nitric oxide. IUBMB Life 55(2):71–81
Dodd IC (2003) Hormonal interactions and stomatal responses. J Plant Growth Regul 22(1):32–46
Egamberdieva D (2008) Plant growth promoting properties of rhizobacteria isolated from wheat and pea grown in loamy sand soil. Turk J Biol 32(1):9–15
Egamberdieva D (2011) Survival of Pseudomonas extremorientalis TSAU20 and P. chlororaphis TSAU13 in the rhizosphere of common bean (Phaseolus vulgaris) under saline conditions. Plant Soil Environ 57(3):122–127
Egamberdiyeva D, Höflich G (2004) Effect of plant growth-promoting bacteria on growth and nutrient uptake of cotton and pea in a semi-arid region of Uzbekistan. J Arid Environ 56(2):293–301
El-Tarabily KA, Youssef T (2010) Enhancement of morphological, anatomical and physiological characteristics of seedlings of the mangrove Avicennia marina inoculated with a native phosphate-solubilizing isolate of Oceanobacillus picturae under greenhouse conditions. Plant Soil 332(1–2):147–162
Essghaier B, Dhieb C, Rebib H, Ayari S, Boudabous ARA, Sadfi-Zouaoui N (2014) Antimicrobial behavior of intracellular proteins from two moderately halophilic bacteria: strain J31 of Terribacillus halophilus and strain M3-23 of Virgibacillus marismortui. J Plant Pathol Microbiol 5(1):1
FAO (2016) FAO Soil Portal. http://www.fao.org/soils-portal/en/
Fedoroff NV (2010) The past, present and future of crop genetic modification. New Biotechnol 27(5):461–465
Fu Q, Liu C, Ding N, Lin Y, Guo B (2010) Ameliorative effects of inoculation with the plant growth-promoting rhizobacterium Pseudomonas sp. DW1 on growth of eggplant (Solanum melongena L.) seedlings under salt stress. Agric Water Manag 97(12):1994–2000
Glick BR (2012) Plant growth-promoting bacteria: mechanisms and applications. Scientifica 2012:963401. https://doi.org/10.6064/2012/963401
Glick BR (2014) Bacteria with ACC deaminase can promote plant growth and help to feed the world. Microbiol Res 169(1):30–39
Gray EJ, Smith DL (2005) Intracellular and extracellular PGPR: commonalities and distinctions in the plant–bacterium signaling processes. Soil Biol Biochem 37(3):395–412
Habib SH, Kausar H, Saud HM (2016) Plant growth-promoting rhizobacteria enhance salinity stress tolerance in okra through ROS-scavenging enzymes. BioMed Res Int 2016:6284547
Hamdia MAE-S, Shaddad M, Doaa MM (2004) Mechanisms of salt tolerance and interactive effects of Azospirillum brasilense inoculation on maize cultivars grown under salt stress conditions. Plant Growth Regul 44(2):165–174
Han S, Yu B, Wang Y, Liu Y (2011) Role of plant autophagy in stress response. Protein Cell 2(10):784–791
Hill C, Guarner F, Reid G, Gibson GR, Merenstein DJ, Pot B, Morelli L, Canani RB, Flint HJ, Salminen S (2014) Expert consensus document: The International Scientific Association for Probiotics and Prebiotics consensus statement on the scope and appropriate use of the term probiotic. Nat Rev Gastroenterol Hepatol 11(8):506
Jewell MC, Campbell BC, Godwin ID (2010) Transgenic plants for abiotic stress resistance. In: Transgenic crop plants. Springer, pp 67–132
Jha Y, Subramanian R, Patel S (2011) Combination of endophytic and rhizospheric plant growth promoting rhizobacteria in Oryza sativa shows higher accumulation of osmoprotectant against saline stress. Acta Physiol Plant 33(3):797–802
Kang S-M, Khan AL, Hamayun M, Hussain J, Joo G-J, You Y-H, Kim J-G, Lee I-J (2012) Gibberellin-producing Promicromonospora sp. SE188 improves Solanum lycopersicum plant growth and influences endogenous plant hormones. J Microbiol 50(6):902
Kang S-M, Khan AL, Waqas M, You Y-H, Kim J-H, Kim J-G, Hamayun M, Lee I-J (2014) Plant growth-promoting rhizobacteria reduce adverse effects of salinity and osmotic stress by regulating phytohormones and antioxidants in Cucumis sativus. J Plant Interact 9(1):673–682
Karlidag H, Esitken A, Yildirim E, Donmez MF, Turan M (2010) Effects of plant growth promoting bacteria on yield, growth, leaf water content, membrane permeability, and ionic composition of strawberry under saline conditions. J Plant Nutr 34(1):34–45
Karuppasamy K, Nagaraj S, Kathiresan K (2011) Stress tolerant rhizobium enhances the growth of Samanea saman (JECQ) Merr. Afr J Basic Appl Sci 3(6):278–284
Khalid M, Bilal M, Hassani D, Iqbal HM, Wang H, Huang D (2017) Mitigation of salt stress in white clover (Trifolium repens) by Azospirillum brasilense and its inoculation effect. Bot Stud 58(1):5
Kim K, Jang Y-J, Lee S-M, Oh B-T, Chae J-C, Lee K-J (2014) Alleviation of salt stress by Enterobacter sp. EJ01 in tomato and Arabidopsis is accompanied by up-regulation of conserved salinity responsive factors in plants. Mol Cells 37(2):109
Kloepper J, Schroth M (1981) Relationship of in vitro antibiosis of plant growth-promoting rhizobacteria to plant growth and the displacement of root microflora. Phytopathology 71(10):1020–1024
Kohler J, Hernández JA, Caravaca F, Roldán A (2009) Induction of antioxidant enzymes is involved in the greater effectiveness of a PGPR versus AM fungi with respect to increasing the tolerance of lettuce to severe salt stress. Environ Exp Bot 65(2):245–252
Krishnan R, Menon RR, Busse H-J, Tanaka N, Krishnamurthi S, Rameshkumar N (2017) Novosphingobium pokkalii sp nov, a novel rhizosphere-associated bacterium with plant beneficial properties isolated from saline-tolerant pokkali rice. Res Microbiol 168(2):113–121
Lau JA, Lennon JT (2012) Rapid responses of soil microorganisms improve plant fitness in novel environments. Proc Natl Acad Sci 109(35):14058–14062
Lippert K, Galinski EA (1992) Enzyme stabilization be ectoine-type compatible solutes: protection against heating, freezing and drying. Appl Microbiol Biotechnol 37(1):61–65
Ma W, Guinel FC, Glick BR (2003) Rhizobium leguminosarum biovar viciae 1-aminocyclopropane-1-carboxylate deaminase promotes nodulation of pea plants. Appl Environ Microbiol 69(8):4396–4402
Mahajan S, Tuteja N (2005) Cold, salinity and drought stresses: an overview. Arch Biochem Biophys 444(2):139–158
Mapelli F, Marasco R, Rolli E, Barbato M, Cherif H, Guesmi A, Ouzari I, Daffonchio D, Borin S (2013) Potential for plant growth promotion of rhizobacteria associated with Salicornia growing in Tunisian hypersaline soils. BioMed Res Int 2013:248078
Marulanda A, Azcón R, Chaumont F, Ruiz-Lozano JM, Aroca R (2010) Regulation of plasma membrane aquaporins by inoculation with a Bacillus megaterium strain in maize (Zea mays L.) plants under unstressed and salt-stressed conditions. Planta 232(2):533–543
Mayak S, Tirosh T, Glick BR (2004a) Plant growth-promoting bacteria confer resistance in tomato plants to salt stress. Plant Physiol Biochem 42(6):565–572
Mayak S, Tirosh T, Glick BR (2004b) Plant growth-promoting bacteria that confer resistance to water stress in tomatoes and peppers. Plant Sci 166(2):525–530
Miller KJ, Wood JM (1996) Osmoadaptation by rhizosphere bacteria. Annu Rev Microbiol 50(1):101–136
Mishra A, Tanna B (2017) Halophytes: potential resources for salt stress tolerance genes and promoters. Front Plant Sci 8:829
Mukhtar S, Ishaq A, Hassan S, Mehnaz S, Mirza MS, Malik KA (2017) Comparison of microbial communities associated with halophyte (Salsola stocksii) and non-halophyte (Triticum aestivum) using culture-independent approaches. Pol J Microbiol 66(3):353–364
Munns R, Gilliham M (2015) Salinity tolerance of crops–what is the cost? New Phytol 208(3):668–673
Munns R, Tester M (2008) Mechanisms of salinity tolerance. Annu Rev Plant Biol 59:651–681
Nabti E, Sahnoune M, Ghoul M, Fischer D, Hofmann A, Rothballer M, Schmid M, Hartmann A (2010) Restoration of growth of durum wheat (Triticum durum var. waha) under saline conditions due to inoculation with the rhizosphere bacterium Azospirillum brasilense NH and extracts of the marine alga Ulva lactuca. J Plant Growth Regul 29(1):6–22
Nabti E, Bensidhoum L, Tabli N, Dahel D, Weiss A, Rothballer M, Schmid M, Hartmann A (2014) Growth stimulation of barley and biocontrol effect on plant pathogenic fungi by a Cellulosimicrobium sp. strain isolated from salt-affected rhizosphere soil in northwestern Algeria. Eur J Soil Biol 61:20–26
Nadeem SM, Zahir ZA, Naveed M, Asghar HN, Arshad M (2010) Rhizobacteria capable of producing ACC-deaminase may mitigate salt stress in wheat. Soil Sci Soc Am J 74(2):533–542
Nadeem SM, Zahir ZA, Naveed M, Nawaz S (2013) Mitigation of salinity-induced negative impact on the growth and yield of wheat by plant growth-promoting rhizobacteria in naturally saline conditions. Ann Microbiol 63(1):225–232
Narula N, Deubel A, Gans W, Behl R, Merbach W (2006) Paranodules and colonization of wheat roots by phytohormone producing bacteria in soil. Plant Soil Environ 52(3):119
Naz I, Bano A, Ul-Hassan T (2009) Isolation of phytohormones producing plant growth promoting rhizobacteria from weeds growing in Khewra salt range, Pakistan and their implication in providing salt tolerance to Glycine max L. Afr J Biotechnol 8(21):5762–5768
Netondo GW, Onyango JC, Beck E (2004) Sorghum and salinity. Crop Sci 44(3):797–805
Nia SH, Zarea MJ, Rejali F, Varma A (2012) Yield and yield components of wheat as affected by salinity and inoculation with Azospirillum strains from saline or non-saline soil. J Saudi Soc Agric Sci 11(2):113–121
Nishma K, Adrisyanti B, Anusha S, Rupali P, Sneha K, Jayamohan N, Kumudini B (2014) Induced growth promotion under in vitro salt stress tolerance on solanum lycopersicum by fluorescent pseudomonads associated with rhizosphere. IJASER 3:422–430
Orhan F (2016) Alleviation of salt stress by halotolerant and halophilic plant growth-promoting bacteria in wheat (Triticum aestivum). Braz J Microbiol 47(3):621–627
Palacio-Rodríguez R, Coria-Arellano JL, López-Bucio J, Sánchez-Salas J, Muro-Pérez G, Castañeda-Gaytán G, Sáenz-Mata J (2017) Halophilic rhizobacteria from Distichlis spicata promote growth and improve salt tolerance in heterologous plant hosts. Symbiosis 73(3):179–189
Patel D, Jha CK, Tank N, Saraf M (2012) Growth enhancement of chickpea in saline soils using plant growth-promoting rhizobacteria. J Plant Growth Regul 31(1):53–62
Piernik A, Hrynkiewicz K, Wojciechowska A, Szymańska S, Lis MI, Muscolo A (2017) Effect of halotolerant endophytic bacteria isolated from Salicornia europaea L. on the growth of fodder beet (Beta vulgaris L.) under salt stress. Arch Agron Soil Sci 63(10):1404–1418
Podmore C (2009) Irrigation salinity–causes and impacts. Primefact 937(1):1–4
Príncipe A, Alvarez F, Castro MG, Zachi L, Fischer SE, Mori GB, Jofré E (2007) Biocontrol and PGPR features in native strains isolated from saline soils of Argentina. Curr Microbiol 55(4):314–322
Qin S, Zhang Y-J, Yuan B, Xu P-Y, Xing K, Wang J, Jiang J-H (2014) Isolation of ACC deaminase-producing habitat-adapted symbiotic bacteria associated with halophyte Limonium sinense (Girard) Kuntze and evaluating their plant growth-promoting activity under salt stress. Plant Soil 374(1–2):753–766
Qurashi AW, Sabri AN (2013) Osmolyte accumulation in moderately halophilic bacteria improves salt tolerance of chickpea. Pak J Bot 45:1011–1016
Raheem A, Ali B (2015) Halotolerant rhizobacteria: beneficial plant metabolites and growth enhancement of Triticum aestivum L. in salt-amended soils. Arch Agron Soil Sci 61(12):1691–1705
Rahnama A, James RA, Poustini K, Munns R (2010) Stomatal conductance as a screen for osmotic stress tolerance in durum wheat growing in saline soil. Funct Plant Biol 37(3):255–263
Rajkumar M, Ae N, Prasad MNV, Freitas H (2010) Potential of siderophore-producing bacteria for improving heavy metal phytoextraction. Trends Biotechnol 28(3):142–149
Ramadoss D, Lakkineni VK, Bose P, Ali S, Annapurna K (2013) Mitigation of salt stress in wheat seedlings by halotolerant bacteria isolated from saline habitats. SpringerPlus 2(1):6
Raymond J, Siefert JL, Staples CR, Blankenship RE (2004) The natural history of nitrogen fixation. Mol Biol Evol 21(3):541–554
Roder A, Hoffmann E, Hagemann M, Berg G (2005) Synthesis of the compatible solutes glucosylglycerol and trehalose by salt-stressed cells of Stenotrophomonas strains. FEMS Microbiol Lett 243(1):219–226
Rojas-Tapias D, Moreno-Galván A, Pardo-Díaz S, Obando M, Rivera D, Bonilla R (2012) Effect of inoculation with plant growth-promoting bacteria (PGPB) on amelioration of saline stress in maize (Zea mays). Appl Soil Ecol 61:264–272
Roy SJ, Negrão S, Tester M (2014) Salt resistant crop plants. Curr Opin Biotechnol 26:115–124
Sadeghi A, Karimi E, Dahaji PA, Javid MG, Dalvand Y, Askari H (2012) Plant growth promoting activity of an auxin and siderophore producing isolate of Streptomyces under saline soil conditions. World J Microbiol Biotechnol 28(4):1503–1509
Santoyo G, Moreno-Hagelsieb G, del Carmen Orozco-Mosqueda M, Glick BR (2016) Plant growth-promoting bacterial endophytes. Microbiol Res 183:92–99
Saravanakumar D, Samiyappan R (2007) ACC deaminase from Pseudomonas fluorescens mediated saline resistance in groundnut (Arachis hypogea) plants. J Appl Microbiol 102(5):1283–1292
Saslis-Lagoudakis CH, Hua X, Bui E, Moray C, Bromham L (2014) Predicting species’ tolerance to salinity and alkalinity using distribution data and geochemical modelling: a case study using Australian grasses. Ann Bot 115(3):343–351
Seckin B, Sekmen AH, Türkan I (2009) An enhancing effect of exogenous mannitol on the antioxidant enzyme activities in roots of wheat under salt stress. J Plant Growth Regul 28(1):12
Shin D-S, Park M-S, Jung S, Lee M-S, Lee K-H, Bae K-S, Kim S-B (2007) Plant growth-promoting potential of endophytic bacteria isolated from roots of coastal sand dune plants. J Microbiol Biotechnol 17(8):1361–1368
Shrivastava P, Kumar R (2015) Soil salinity: a serious environmental issue and plant growth promoting bacteria as one of the tools for its alleviation. Saudi J Biol Sci 22(2):123–131
Siddikee MA, Chauhan PS, Anandham R, Han G-H, Sa T (2010) Isolation, characterization, and use for plant growth promotion under salt stress, of ACC deaminase-producing halotolerant bacteria derived from coastal soil. J Microbiol Biotechnol 20(11):1577–1584
Siddikee MA, Glick BR, Chauhan PS, Jong Yim W, Sa T (2011) Enhancement of growth and salt tolerance of red pepper seedlings (Capsicum annuum L.) by regulating stress ethylene synthesis with halotolerant bacteria containing 1-aminocyclopropane-1-carboxylic acid deaminase activity. Plant Physiol Biochem 49(4):427–434
Štajner D, Kevrešan S, Gašić O, Mimica-Dukić N, Zongli H (1997) Nitrogen and Azotobacter chroococcum enhance oxidative stress tolerance in sugar beet. Biol Plant 39(3):441
Stutz EW, Défago G, Kern H (1986) Naturally occurring fluorescent pseudomonads involved in suppression of black root rot of tobacco. Phytopathology 76(2):181–185
Suárez R, Wong A, Ramírez M, Barraza A, Orozco MC, Cevallos MA, Lara M, Hernández G, Iturriaga G (2008) Improvement of drought tolerance and grain yield in common bean by overexpressing trehalose-6-phosphate synthase in rhizobia. Mol Plant-Microbe Interact 21(7):958–966
Suarez C, Cardinale M, Ratering S, Steffens D, Jung S, Montoya AMZ, Geissler-Plaum R, Schnell S (2015) Plant growth-promoting effects of Hartmannibacter diazotrophicus on summer barley (Hordeum vulgare L.) under salt stress. Appl Soil Ecol 95:23–30
Szymańska S, Piernik A, Hrynkiewicz K (2013) Metabolic potential of microorganisms associated with the halophyte Aster tripolium L. in saline soils. Ecol Quest 18(1):9–19
Taghavi S, Garafola C, Monchy S, Newman L, Hoffman A, Weyens N, Barac T, Vangronsveld J, van der Lelie D (2009) Genome survey and characterization of endophytic bacteria exhibiting a beneficial effect on growth and development of poplar trees. Appl Environ Microbiol 75(3):748–757
Tank N, Saraf M (2010) Salinity-resistant plant growth promoting rhizobacteria ameliorates sodium chloride stress on tomato plants. J Plant Interact 5(1):51–58
Tewari S, Arora NK (2014) Multifunctional exopolysaccharides from Pseudomonas aeruginosa PF23 involved in plant growth stimulation, biocontrol and stress amelioration in sunflower under saline conditions. Curr Microbiol 69(4):484–494
Tiwari S, Singh P, Tiwari R, Meena KK, Yandigeri M, Singh DP, Arora DK (2011) Salt-tolerant rhizobacteria-mediated induced tolerance in wheat (Triticum aestivum) and chemical diversity in rhizosphere enhance plant growth. Biol Fertil Soils 47(8):907
Upadhyay SK, Singh DP, Saikia R (2009) Genetic diversity of plant growth promoting rhizobacteria isolated from rhizospheric soil of wheat under saline condition. Curr Microbiol 59(5):489–496
Upadhyay SK, Singh JS, Saxena AK, Singh DP (2012) Impact of PGPR inoculation on growth and antioxidant status of wheat under saline conditions. Plant Biol 14(4):605–611
Van Loon L (2007) Plant responses to plant growth-promoting rhizobacteria. Eur J Plant Pathol 119(3):243–254
Vannier N, Mony C, Bittebière A-K, Vandenkoornhuyse P (2015) Epigenetic mechanisms and microbiota as a toolbox for plant phenotypic adjustment to environment. Front Plant Sci 6:1159
Welsh DT (2000) Ecological significance of compatible solute accumulation by micro-organisms: from single cells to global climate. FEMS Microbiol Rev 24(3):263–290
Yamaguchi T, Blumwald E (2005) Developing salt-tolerant crop plants: challenges and opportunities. Trends Plant Sci 10(12):615–620
Yang J, Kloepper JW, Ryu C-M (2009) Rhizosphere bacteria help plants tolerate abiotic stress. Trends Plant Sci 14(1):1–4
Yao L, Wu Z, Zheng Y, Kaleem I, Li C (2010) Growth promotion and protection against salt stress by Pseudomonas putida Rs-198 on cotton. Eur J Soil Biol 46(1):49–54
Yildirim E, Turan M, Ekinci M, Dursun A, Cakmakci R (2011) Plant growth promoting rhizobacteria ameliorate deleterious effect of salt stress on lettuce. Sci Res Essays 6(20):4389–4396
Yuan Z, Druzhinina IS, Labbé J, Redman R, Qin Y, Rodriguez R, Zhang C, Tuskan GA, Lin F (2016) Specialized microbiome of a halophyte and its role in helping non-host plants to withstand salinity. Sci Rep 6:32467
Yue H, Mo W, Li C, Zheng Y, Li H (2007) The salt stress relief and growth promotion effect of Rs-5 on cotton. Plant Soil 297(1–2):139–145
Zahir ZA, Arshad M, Frankenberger WT (2004) Plant growth promoting rhizobacteria: applications and perspectives in agriculture. Adv Agron 81:98–169
Zahir ZA, Ghani U, Naveed M, Nadeem SM, Asghar HN (2009) Comparative effectiveness of Pseudomonas and Serratia sp. containing ACC-deaminase for improving growth and yield of wheat (Triticum aestivum L.) under salt-stressed conditions. Arch Microbiol 191(5):415–424
Zarea M, Hajinia S, Karimi N, Goltapeh EM, Rejali F, Varma A (2012) Effect of Piriformospora indica and Azospirillum strains from saline or non-saline soil on mitigation of the effects of NaCl. Soil Biol Biochem 45:139–146
Zhou N, Zhao S, Tian CY (2017) Effect of halotolerant rhizobacteria isolated from halophytes on the growth of sugar beet (Beta vulgaris L.) under salt stress. FEMS Microbiol Lett 364(11). https://doi.org/10.1093/femsle/fnx091
Zhu J-K (2002) Salt and drought stress signal transduction in plants. Annu Rev Plant Biol 53(1):247–273
Acknowledgment
CSIR-CSMCRI Communication No.: PRIS-42/2018.
Author information
Authors and Affiliations
Corresponding authors
Editor information
Editors and Affiliations
Rights and permissions
Copyright information
© 2019 Springer Nature Singapore Pte Ltd.
About this chapter
Cite this chapter
Alexander, A., Mishra, A., Jha, B. (2019). Halotolerant Rhizobacteria: A Promising Probiotic for Saline Soil-Based Agriculture. In: Kumar, M., Etesami, H., Kumar, V. (eds) Saline Soil-based Agriculture by Halotolerant Microorganisms. Springer, Singapore. https://doi.org/10.1007/978-981-13-8335-9_3
Download citation
DOI: https://doi.org/10.1007/978-981-13-8335-9_3
Published:
Publisher Name: Springer, Singapore
Print ISBN: 978-981-13-8334-2
Online ISBN: 978-981-13-8335-9
eBook Packages: Biomedical and Life SciencesBiomedical and Life Sciences (R0)