Abstract
Overcrowded urban areas are exposed to quotidian loads of toxic metals and PAHs. Simultaneous analysis of mentioned pollutants in surface soil samples of a crowded urban area in Tehran City is considered in this study. A total number of 112 samples were collected from pollution hotspots including gas stations, construction sites, bus terminal and waste recovery centers in summer 2016. Total, anthropogenic and bioavailable concentrations of metals, cadmium, cobalt, chromium, copper, manganese, nickel, lead and zinc, as well as ecological risk index (RI) values were calculated. Rather than Cd, the concentration of other metals was not remarkably different from reference values. The descending order of anthropogenic and bioavailable fractions in metals was proved to be as follows: Cd (87.87%) > Cr (40.14%) > Ni (38.64%) > Pb (37.40%) > Co (8.42%) > Cu (7.98%) > Zn (5.57%) > Mn (3.94%) and Cu (6.84%) > Zn (6.47%) > Pb (3.98%) > Ni (3.97%) > Cr (1.99%) > Cd (1.98%) > Mn (1.45%) > Co (1.39%), respectively. The results of the RI values also indicated that around 80% of total samples lie within a considerable ecological risk level. The total concentration of 16 EPA-approved PAHs was detected to be within the range of 0.62 and 3.51 mg/kg. The majority of PAHs detected in all samples was those with relatively higher molecular weights (higher number of aromatic rings). Accordingly, the major source of PAHs in surface soils was determined to be petroleum/gas combustion due to traffic loads and heating systems. The synergy observed in spatial distribution of toxic metals and PAHs confirms relatively identical pollution sources and at the same time exacerbates the adverse effects on human and ecological health.
Article Highlights
-
The combined approach of toxic metals and PAHs in urban soil analysis is considered.
-
The source detection of PAHs in urban soils is determined.
-
The source detection of toxic metals in urban soils is investigated.
-
The fractionation of toxic metals in bulk, anthropogenic and bioavailable fractions is performed.
Similar content being viewed by others
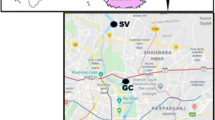
Explore related subjects
Discover the latest articles, news and stories from top researchers in related subjects.Avoid common mistakes on your manuscript.
Introduction
Industrialization and urbanization may impose adverse effects on concentration, mobility and bioavailability of toxic metals in soils (Afkhami et al. 2013; Karbassi et al. 2014; Nasrabadi et al. 2016). Soil pollution in metropolitan cities occurs as a result of various human activities that can be somehow considered complex. The degree of soil contamination might be tracked when the measurements are continuously compared with those of the previous years (Galitskova and Murzayeva 2016). Increase in the elemental (Pb, Zn, Cu and Cd) contents of soils in the Eastern district of Moscow correlated with the growth of industrial and vehicles’ emission over a time span of about 21 years (Kosheleva and Nikiforova 2016). The elemental concentration in urban soil may have adverse health effects on children and therefore it is proposed to find suitable playgrounds by means of GIS (Delahaye et al. 1998). An investigation of the hazardous metal contents of soil in 13 parks of urban areas revealed that shaded areas within parks contained higher concentrations than the open areas (Galušková et al. 2011). Furthermore, bioaccessibility and human health risk from heavy metals in exposed lawn soils from 28 urban parks in southern China’s largest city, Guangzhou, were evaluated in a series of studies (Gu et al. 2016a, b; Gu and Gao 2018). Many studies have shown that the metal contents of soils within the urban area are higher in the vicinity of industrial parks than at other sites; nevertheless, wind could disperse the pollution within the whole area (Tume et al. 2008; Li et al. 2013; Lü et al. 2018). Some studies have reported the use of various methods including chemical stabilization, phytoremediation and microbial techniques to control pollution within soil columns (Saffari 2018; Usman et al. 2018; Adetitun et al. 2018; Abbaslou and Bakhtiari 2017). Treatment of soil pollution should take into account various aspects such as reducing health risk, furnishing cultivable soil and so on. These in turn depend on the chemistry and physical characteristics of soil (Wuana and Okieimen 2011).
In terms of organic pollutants, polycyclic aromatic hydrocarbons (PAHs) are among the most notorious compounds found in urban areas. Due to their hydrophobicity, PAHs have a high tendency to get adsorbed by particles and consequently be transported to remote distances from their source of generation (Tu et al. 2018). Being persistent due to their low biodegradation potential (Feng et al. 2016), a certain number of PAHs are also proved as carcinogens/mutagens (Kim et al. 2013). They may be easily exposed to humans directly through dermal (dusts in urban areas, soils in playgrounds, etc.) and inhalation routes. Indirect exposure is also inevitable through the digestion route when considering the entry of PAHs into the food chain (Li et al. 2015; Xia et al. 2015). Concerning concentration of PAHs have recently been detected in urban areas due to oil/coal combustion, traffic-related emissions, waste combustion and urban wastewater drainage in Kaohsiung City, Taiwan (Tu et al. 2018); Aracaju City, Brazil (Souza et al. 2018); Shanghai, China (Liu et al. 2016; Huang et al. 2017) and Guangzhou, China (Ke et al. 2017).
The main golas of this study are (1) to determine the total, bioavailable and anthropogenic concentrations of toxic metals, as well as 16 PAHs in surface soil samples collected from hotspots of an intensified urban district in Tehran City and (2) to find any probable coherence between the distribution pattern of toxic metals and PAHs.
Study Area
District 6 of Tehran municipality covers some of the most overcrowded areas in central to northern parts of the city. More than 8% of total urban trips evolve in this district whose surface area is around 3% of the total Tehran urban areas. Intensive road networks as well as administrative buildings, educational centers, commercial buildings, hospital and military land use are considered as the main reasons for such remarkable everyday commute demand (Fazeli et al. 2018). To consider the worst scenario of urban soil pollution due to traffic load, the sampling locations are selected in a way to have the maximal exposure. Accordingly, 14 sampling stations were selected, namely Valfajr CNG station (1), Yousefabad gas station (2), Beyhaghi bus terminal (3), Beyhaghi municipal waste recovery center (4), Beyhaghi CNG station (5), Valiasr St., Saee Park gas station (6), Chamraan and Ale-ahmad highways junction (7), Mirzaye Shirazi St., gas station (8), North Karegar St. gas station (9), Valiasr, Zartosht St., gas station (10), Valiasr Square, subway construction site (11), vicinity of Imam Khomeini hospital (12), Enghelab St., gas station (13) and Gharani St., gas station (14). Location details of sampling points as well as the location of District 6 in Tehran City and Iran are shown in Fig. 1.
Materials and Methods
Sampling Campaign
A total number of 112 surface soil samples (56 samples for detection of toxic metals and 56 samples for detection of PAHs) were collected from 14 stations. Soil samples were collected by a hand auger to a maximum depth of 50 cm. Four sub-samples (Z grid) from each station were collected and mixed to generate 28 composite samples for further analysis. Sampling campaign was performed in summer 2016. All plant and anthropogenic leftovers were removed before sampling was done by spiral auger. Samples for metal detection were kept in plastic bags, while those for PAHs detection were wrapped in aluminum foils.
Toxic Metal Detection
Samples for detection of metals were dried at room temperature and sieved through 63 μm mesh size for further analysis. To detect the total concentration of metals, 0.5 g of the sample was digested with a mixture of aqua regia (HNO3 and HCl with a volumetric ration of 3:1) and HClO4 (Chester and Hughes 1967). After filtration, the final volume was calibrated to 50 mL.
To determine the bioavailable and anthropogenic share of total metal concentrations, single step extraction schemes were considered (Hosseini Alhashemi et al. 2011). For anthropogenic share detection, 2 g of sieved samples was digested by 15 mL of 0.53 N HCl for half an hour and made to a final volume of 50 mL after filtration. To quantify the bioavailable share 10 g of sieved samples was digested for 30 min by a mixture of NaOH (1 N) and acetic acid at pH 5 and refluxed for a whole day. The metal contents (total, bioavailable and anthropogenic) in relevant solutions were analyzed by ICPAES. Duplicate/triplicate samples as well as certified reference material (MESS-1) measurement were performed to check the QC/QA of the procedures. The relative standard deviation of the results was less than 10%.
PAHs Detection
To detect PAHs, soil samples were freeze-dried and sieved to achieve the fraction < 63 μm. PAHs extraction scheme in this study is explained in detail by Liu et al. (2016). Concisely, 2 g of sieved samples was digested by a mixture of acetone and DCM (1:1, v/v) for 18 h using a Soxhlet apparatus at 65 °C. Purification was performed by a self-packet silica–alumina column. The final eluent was concentrated to 1 mL. The PAHs content in soil samples was analyzed by a Varian 3900 GC coupled with a Varian Ion Trap Saturn 2000 MS, with helium as the carrier gas at 1 mL/min flow. Quality assurance and control were checked according to the procedure suggested by Liu et al. (2016); two blanks were analyzed through which target compounds were determined to be under detection limits. Furthermore, a mixture of 16 EPA PAHs as a standard was added to each sample prior to extraction. The average recovery range of added standard was measured to be between 74.5% and 115%. The following 16 PAHs approved by USEPA were considered for analysis in this research: acenaphthene (AC), acenaphthylene (ACE), antheracene (AN), benzo[a]anthracene (BaA), benzo[b]fluoranthene (BbF), benzo[k]fluoranthene (BkF), benzo[a]pyrene (BaP), benzo[g,h,i]perylene (BP), chrysene (CH), dibenzo[a,h]anthracene (DA), fluorine (FL), fluoranthene (FLU), indeno[1,2,3-cd]pyrene (IP), phenanthrene (PH), naphthalene (NA) and pyrene (PY).
Results
The metals considered for evaluation of toxicity in urban soil in this study are cadmium, cobalt, chromium, copper, manganese, nickel, lead and zinc. The concentration of each metal accompanied by comparison with earth crust, shale and background mean values is presented in Table 1. In comparison with background values (mean values of Tehran City circumference), all metals except for Ni showed higher contents. When compared with shale, apart from Co and Zn, other metals’ mean values exceeded the reference values. In comparison with the mean earth crust values, however, only Cd and Pb had higher concentrations. Cd rather than other metals showed extremely high values (around 36, 17 and 8 times greater than those of mean earth crust, shale and background values, respectively).
The total metal concentration in soil samples consists of lithogenic (minerals content) and anthropogenic (caused by human impacts) fractions. Anthropogenic concentrations of metals are measured and shown in Table 2. Anthropogenic fractions may be classified into three distinct levels of high (Cd by more than 87%), medium (Cr, Ni and Pb by around 40%) and low (Co, Cu, Zn and Mn by less than 10%). Fossil fuel combustion derived from traffic and heating systems, material usage and abrasion/corrosions, lights, batteries, medical materials and domestic wastes are considered among anthropic sources of metal pollution in urban areas (Wu et al. 2019).
Both lithogenic and anthropogenic metal concentrations may contain bioavailable fractions which are easily uptaken by biota. Accordingly, the higher the bioavailable fraction of a specific metal, the higher is the expected risk level of that metal. The bioavailable fractions of the total metal concentrations are shown in Table 3. Cu (6.84%) and Zn (6.47%) show the highest bioavailable fractions, while Mn (1.45%) and Co (1.39%) are considered as metals showing minimal values.
Index Analysis
To evaluate the toxicity level of metal pollutants in soil samples, ecological risk index (RI) is used. The formula for RI is as follows:
where E ir is the relevant risk factor for contaminant (i) and is calculated as:
where T ir is the toxic response factor for contaminant (i) and is defined by dividing the metal concentration in soil by the background value. C if is the contamination factor of each contaminant (30 for Cd, 5 for Pb, Cu and Ni, 2 for Cr and 1 for Zn). The higher the value of Cf, the higher is the risk exposed to the ecological environment by the contaminant. The classification of different RI values and relevant risk levels is shown in Table 4.
The values of toxic response factor (Tr), risk factors (Er) and ecological risk index (RI) of different surface soil samples are calculated and shown in Table 5. According to the classification ladder (Hakanson 1980), 3 samples (around 21% of the whole area) are categorized in the considerable risk level, while the other 11 samples remained within the moderate class.
As typical and ubiquitous pollutants in urban areas, PAHs were also considered for analysis in this study. The concentrations of 16 EPA-approved PAHs as well as the contents of carcinogenics (Monaco et al. 2017) including benzo[a]pyrene (carcinogenic), benzo[a]anthracene (probably carcinogenic to humans), benzo[b]fluoranthene, benzo[k]fluoranthene, chrysene, dibenzo[a,h]anthracene, indeno[1,2,3-cd]pyrene and naphthalene (probably carcinogenic to humans) were provided. To track the pollution sources, a couple of supplementary metrics were also calculated including the concentration of low molecular weight (LMW, Σ2–3 rings) and high molecular weight (HMW, Σ4–6 rings) PAHs and the ratio (LMW/HMW). The results are shown in Table 6.
PAHs in the environment are categorized into two distinct classes of petrogenics and pyrolytics. Petrogenics are those containing two or three rings with relatively lower molecular weights. With higher molecular weights, pyrolytics have four to six rings in their chemical structure (Yang et al. 2016). The major source of petrogenics is considered to be crude oil or petroleum spill/leakage, while detection of pyrolytics is often attributed to biomass and fossil fuel combustion (Katsoyiannis and Breivik 2014).
To track the source of PAHs more specifically, a variety of parameters are suggested by researchers; ratios of fluoranthene (FLU)/[FLU + pyrene (PY)] values lower than 0.4, between 0.4 and 0.5, and higher than 0.5 indicate crude oil/petroleum components, petroleum combustion and coal/biomass combustion, respectively (Franco et al. (2017). Ratio of BaA/(BaA + Chr) as well as InP/(InP + BghiP) values lower than 0.2 refers to petroleum, while higher values are considered as combustion-derived products (Wang et al. 2015). The same rule works for the ratio of Ant/(Ant + Phe); however, the limit here is proved to be 0.1 (Qiao et al. 2006). Furthermore, if the ratio (LMW/HMW) is higher than unit, the source of PAHs is attributed to petroleum products while lower than one values are due to petroleum combustion (Tu et al. 2018).
According to Figs. 2, 3 and 4, it seems that the main source of PAHs in the majority of samples is petroleum combustion. Additionally, low values of LMW/HMW (between 0.12 and 0.31) also confirm the source apportionment of PAHs within the study area (Fig. 5).
To compare the distribution pattern of major inorganic (toxic metals) and organic (PAHs) pollutants in this urban district, the values of risk index (RI) and ΣPAHs concentrations are illustrated in a symbolic way to show the probable similarities (Fig. 6).
As it is seen, all minimal, maximal and intermediate values for both metrics are coincided indicating a kind of similarity in their source and even transport scheme.
Conclusions
In this study, the adverse effects of urbanization on soil quality are investigated. The case study was selected in such a way s to cover an overcrowded district in the megacity of Tehran, where a variety of urban land use is observed. To have a thorough overview of soil pollution, both toxic metals and PAHs were considered for analysis. Furthermore, supplementary information over anthropogenic and bioavailable fractions of total metal concentration as well as source apportionment of PAHs was taken into consideration. Hotspots including some gas stations, construction sites, bus terminal, municipal waste recovery center and hospital were selected as the final 14 stations for composite sampling. Rather than Cd which shows remarkably high concentrations, the total concentration of other metals were not at all or at least slightly higher than the reference values. The descending order of anthropogenic fraction in metals was calculated as follows: Cd (87.87%) > Cr (40.14%) > Ni (38.64%) > Pb (37.40%) > Co (8.42%) > Cu (7.98%) > Zn (5.57%) > Mn (3.94%). Such order places an emphasis on attributing extremely high values of Cd to heavy vehicle traffic as suggested by Dias-Ferreira et al. (2016) in Portugal, Horváth et al. (2015) in Hungary and Qing et al. (2015) in China. Bioavailable concentration of metals in soil was classified as: Cu (6.84%) > Zn (6.47%) > Pb (3.98%) > Ni (3.97%) > Cr (1.99%) > Cd (1.98%) > Mn (1.45%) > Co (1.39%). Relatively considerable mobility of Pb and Ni (around 4%) as well as Cd and Cr (around 2%) may cause subsequent concerns of surface and groundwater pollution. Such potential hazard was confirmed by the results of RI calculation; around 80% of total samples were proved to locate at a considerable level of ecological risk.
In terms of PAHs, the majority of the detected components were those with higher molecular weights, indicating the pyrolytic source. Petroleum combustion due to heavy traffic load as well as gas combustion due to heating systems is considered the main source of such pollution as a result of a variety of metrics including the ratios of Flu/(Flu + Py), BaA/(BaA + Chr), InP/(InP + BghiP), Ant/(Ant + Phe) and LMW/HMW. A kind of synergy is observed in the spatial distribution pattern of PAHs and toxic metals, which may be further studied in detail regarding human health issues in megacities like Tehran.
References
Abbaslou H, Bakhtiari S (2017) Phytoremediation potential of heavy metals by two native pasture plants (Eucalyptus grandis and Ailanthus altissima) assisted with AMF and fibrous minerals in contaminated mining regions. Pollution 3(3):471–486
Adetitun D, Akinmayowa V, Atolani O, Olayemi A (2018) Biodegradation of jet fuel by three Gram negative bacilli isolated from kerosene contaminated soil. Pollution 4(2):291–303
Afkhami F, Karbassi AR, Nasrabadi T, Vosoogh A (2013) Impact of oil excavation activities on soil metallic pollution, case study of an Iran southern oil field. Environ Earth Sci 70(3):1219–1224
Alloway BJ (1995) Heavy metals in soils, 2nd edn. Blackie Academic & Professional, London
Chester R, Hughes RM (1967) A chemical technique for the separation of ferro-manganese minerals, carbonate minerals and adsorbed trace elements from pelagic sediment. Chem Geol 2:249–262
Delahaye D, Folligne P, Guermond Y, Vapaille JP, Zambeaux R (1998) Soil pollution in an urban area: a GIS approach. Cybergeo Eur J Geogr. http://journals.openedition.org/cybergeo/1575. https://doi.org/10.4000/cybergeo.1575
Dias-Ferreira C, Pato RL, Varejão JB, Tavares AO, Ferreira AJ (2016) Heavy metal and PCB spatial distribution pattern in sediments within an urban catchment—contribution of historical pollution sources. J Soils Sediments 16(11):2594–2605
Eghbal N, Nasrabadi T, Karbassi AR, Taghavi L (2019) Evaluating the potential of plants (leaves) in removal of toxic metals from urban soils (case study of a district in Tehran city). Pollution 5(2):387–394
Fazeli G, Karbassi AR, Khoramnejadian Sh, Nasrabadi T (2018) Anthropogenic share of metal contents in soils of urban areas. Pollution 4(4):697–706
Feng F, Harper RL, Reynolds PN (2016) BMPR2 gene delivery reduces mutation related PAH and counteracts TGF-b-mediated pulmonary cell signalling. Respirology 21:526–532
Franco CFJ, de Resende MF, de Almeida Furtado L, Brasil TF, Eberlin MN, Netto ADP (2017) Polycyclic aromatic hydrocarbons (PAHs) in street dust of Rio de Janeiro and Niteroi, Brazil: particle size distribution, sources and cancer risk assessment. Sci Total Environ 599:305–313
Galitskova YM, Murzayeva AI (2016) Urban soil contamination. Procedia Eng 153:162–166
Galušková I, Borůvka L, Drábek O (2011) Urban soil contamination by potentially risk elements. Soil Water Res 6(2):55–60
Gu YG, Gao YP (2018) Bioaccessibilities and health implications of heavy metals in exposed-lawn soils from 28 urban parks in the megacity Guangzhou inferred from an in vitro physiologically-based extraction test. Ecotoxicol Environ Saf 148:747–753
Gu YG, Gao YP, Lin Q (2016a) Contamination, bioaccessibility and human health risk of heavy metals in exposed-lawn soils from 28 urban parks in southern China’s largest city, Guangzhou. Appl Geochem 67:52–58
Gu YG, Lin Q, Gao YP (2016b) Metals in exposed-lawn soils from 18 urban parks and its human health implications in southern China’s largest city, Guangzhou. J Clean Prod 115:122–129
Hakanson L (1980) An ecological risk index for aquatic pollution control—a sedimentological approach. Water Res 14:975–1001
Horváth A, Szűcs P, Bidló A (2015) Soil condition and pollution in urban soils: evaluation of the soil quality in a Hungarian town. J Soils Sediments 15(8):1825–1835
Hosseini Alhashemi AS, Karbassi AR, Hassanzadeh Kiabi B, Monavari SM, Nabavi SMB (2011) Accumulation and bioaccessibility of trace elements in wetland sediments. Afr J Biotechnol 10(9):1625–1636
Huang Y, Liu M, Wang R, Khan SK, Gao D, Zhang Y (2017) Characterization and source apportionment of PAHs from a highly urbanized river sediments based on land use analysis. Chemosphere 184:1334–1345
Karbassi A, Nasrabadi T, Rezai M, Modabberi S (2014) Pollution with metals (As, Sb, Hg, Zn) in agricultural soil located close to Zarshuran gold mine, Iran. Environ Eng Manag J 13(1):115–122
Katsoyiannis A, Breivik K (2014) Model-based evaluation of the use of polycyclic aromatic hydrocarbons molecular diagnostic ratios as a source identification tool. Environ Pollut 184:488–494
Ke CL, Gu YG, Liu Q (2017) Polycyclic aromatic hydrocarbons (PAHs) in exposed-lawn soils from 28 urban parks in the megacity Guangzhou: occurrence, sources, and human health implications. Arch Environ Contam Toxicol 72(4):496–504
Kim K-H, Jahan SA, Kabir E, Brown RJ (2013) A review of airborne polycyclic aromatic hydrocarbons (PAHs) and their human health effects. Environ Int 60:71–80
Kosheleva NE, Nikiforova EM (2016) Long-term dynamics of urban soil pollution with heavy metals in Moscow. Appl Environ Soil Sci. 2016:5602795. https://doi.org/10.1155/2016/5602795
Li X, Liu L, Wang Y, Luo G, Chen X, Yang X, He X (2013) Heavy metal contamination of urban soil in an old industrial city (Shenyang) in Northeast China. Geoderma 192:50–58
Li J, Dong H, Zhang D, Han B, Zhu C, Liu S, Liu X, Ma Q, Li X (2015) Sources and ecological risk assessment of PAHs in surface sediments from Bohai Sea and northern part of the Yellow Sea, China. Mar Pollut Bull 96:485–490
Liu S, Liu X, Liu M, Yang B, Cheng L, Li Y, Qadeer A (2016) Levels, sources and risk assessment of PAHs in multi-phases from urbanized river network system in Shanghai. Environ Pollut 219:555–567
Lü H, Mo CH, Zhao HM, Xiang L, Katsoyiannis A, Li YW, Wong MH (2018) Soil contamination and sources of phthalates and its health risk in China: a review. Environ Res 164:417–429
Monaco D, Chianese E, Riccio A, Delgado-Sanchez A, Lacorte S (2017) Spatial distribution of heavy hydrocarbons, PAHs and metals in polluted areas. The case of “Galicia”, Spain. Mar Pollut Bull 121(1–2):230–237
Nasrabadi T, Ruegner H, Sirdari ZZ, Schwientek M, Grathwohl P (2016) Using total suspended solids (TSS) and turbidity as proxies for evaluation of metal transport in river water. Appl Geochem 68:1–9
Qiao M, Wang C, Huang S, Wang D, Wang Z (2006) Composition, sources, and potential toxicological significance of PAHs in the surface sediments of the Meiliang Bay, Taihu Lake, China. Environ Int 32:28–33
Qing X, Yutong Z, Shenggao L (2015) Assessment of heavy metal pollution and human health risk in urban soils of steel industrial city (Anshan), Liaoning, Northeast China. Ecotoxicol Environ Saf 120:377–385
Saffari M (2018) Chemical stabilization of some heavy metals in an artificially multi-elements contaminated soil, using rice husk biochar and coal fly ash. Pollution 4(4):547–562
Souza MR, Santos E, Suzarte JS, Carmo LO, Frena M, Damasceno FC, Alexandre MR (2018) Concentration, distribution and source apportionment of polycyclic aromatic hydrocarbons (PAH) in Poxim River sediments, Brazil. Mar Pollut Bull 127:478–483
Tu YT, Ou JH, Tsang DCW, Dong CD, Chen CW, Kao CM (2018) Source identification and ecological impact evaluation of PAHs in urban river sediments: a case study in Taiwan. Chemosphere 194:666–674
Tume P, Bech J, Sepulveda B, Tume L, Bech J (2008) Concentrations of heavy metals in urban soils of Talcahuano (Chile): a preliminary study. Environ Monit Assess 140(1–3):91–98
Turekian KK, Wedepohl KH (1961) Distribution of the elements in some major units of Earth’s crust. Bull Geol Soc Am 72:175–192
Usman M, Waseem M, Mani N, Andiego N (2018) Optimization of soil aquifer treatment by chemical oxidation with hydrogen peroxide addition. Pollution 4(3):369–379
Wang M, Wang C, Hu X, Zhang H, He S, Lv S (2015) Distributions and sources of petroleum, aliphatic hydrocarbons and polycyclic aromatic hydrocarbons (PAHs) in surface sediments from Bohai Bay and its adjacent river, China. Mar Pollut Bull 90:88–94
Wu S, Zhou S, Bao H, Chen D, Wang C, Li B, Tong G, Yuan Y, Xu B (2019) Improving risk management by using the spatial interaction relationship of heavy metals and PAHs in urban soil. J Hazard Mater 364:108–116
Wuana RA, Okieimen FE (2011) Heavy metals in contaminated soils: a review of sources, chemistry, risks and best available strategies for remediation. Isrn Ecol 2011:402647. https://doi.org/10.5402/2011/402647
Xia X, Xia N, Lai Y, Dong J, Zhao P, Zhu B, Li Z, Ye W, Yuan Y, Huang J (2015) Response of PAH-degrading genes to PAH bioavailability in the overlying water, suspended sediment, and deposited sediment of the Yangtze River. Chemosphere 128:236–244
Yang R, Xie T, Li A, Yang H, Turner S, Wu G, Jing C (2016) Sedimentary records of polycyclic aromatic hydrocarbons (PAHs) in remote lakes across the Tibetan Plateau. Environ Pollut 214:1–7
Author information
Authors and Affiliations
Corresponding author
Ethics declarations
Conflict of interest
The authors declare that they have no conflict of interest.
Rights and permissions
About this article
Cite this article
Fazeli, G., Karbassi, A., Khoramnejadian, S. et al. Evaluation of Urban Soil Pollution: A Combined Approach of Toxic Metals and Polycyclic Aromatic Hydrocarbons (PAHs). Int J Environ Res 13, 801–811 (2019). https://doi.org/10.1007/s41742-019-00206-8
Received:
Revised:
Accepted:
Published:
Issue Date:
DOI: https://doi.org/10.1007/s41742-019-00206-8