Abstract
Background
Accumulating evidence indicates that type 2 diabetes is associated with an increased risk to develop breast cancer. This risk has been attributed to hyperglycemia, hyperinsulinemia and chronic inflammation. As yet, however, the mechanisms underlying this association are poorly understood. Here, we studied the effect of high glucose and insulin on breast cancer-derived cell proliferation, migration, epithelial-mesenchymal transition (EMT) and invasiveness, as well as its relationship to reactive oxygen species (ROS) production and the plasminogen activation system.
Methods
MDA-MB-231 cell proliferation, migration and invasion were assessed using 3-(4, 5-dimethylthiazol-2-yl)-2, 5-diphenyltetrazolium bromide (MTT), scratch-wound and matrigel transwell assays, respectively. ROS production was determined using 2′ 7′-dichlorodihydrofluorescein diacetate. The expression of E-cadherin, vimentin, fibronectin, urokinase plasminogen activator (uPA), its receptor (uPAR) and its inhibitor (PAI-1) were assessed using qRT-PCR and/or Western blotting assays, respectively. uPA activity was determined using gel zymography.
Results
We found that high glucose stimulated MDA-MB-231 cell proliferation, migration and invasion, together with an increased expression of mesenchymal markers (i.e., vimentin and fibronectin). These effects were further enhanced by the simultaneous administration of insulin. In both cases, the invasion and growth responses were found to be associated with an increased expression of uPA, uPAR and PAI-1, as well as an increase in active uPA. An osmolality effect of high glucose was excluded by using mannitol at an equimolar concentration. We also found that all changes induced by high glucose and insulin were attenuated by the anti-oxidant N-acetylcysteine (NAC) and, thus, depended on ROS production.
Conclusions
From our data we conclude that hyperglycemia and hyperinsulinemia can promote breast cancer cell proliferation, migration and invasion. We found that these features were associated with increased expression of the mesenchymal markers vimentin and fibronectin, as well as increased uPA expression and activation through a mechanism mediated by ROS.
Similar content being viewed by others
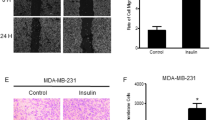
Avoid common mistakes on your manuscript.
1 Introduction
Breast cancer and diabetes increasingly rank among the major health care problems worldwide, due in part to the adoption of sedentary lifestyles and diets that are rich in carbohydrates and lipids [1, 2]. Accumulating evidence indicates a strong association between type 2 diabetes and breast cancer [3–6]. As yet, however, the biological and molecular mechanisms underlying this association are not fully understood. Metabolic (hyperglycemia), hormonal (insulin/insulin like growth factor 1 or IGF-1) and inflammatory anomalies in diabetic patients may act synergistically with other risk factors (diet, environmental exposure) and, by doing so, trigger cellular proliferation and ultimately the development of breast cancer [5].
In recent years, hyperglycemia and hyperinsulinemia have been recognized as key risk factors for the initiation and progression of breast cancer. Hyperglycemia provides a favorable environment for the growth and survival of breast cancer cells and has been considered as a secondary cause based on the fact that glucose is known to be used as an energy source by cancer cells [7, 8]. Moreover, it has been found that the survival of diabetic breast cancer patients with hyperglycemia is reduced compared to patients with an adequate metabolic control [9]. Additionally, high glucose levels have been found to promote epithelial-mesenchymal transition (EMT) [10], which is characterized by the loss of epithelial and the acquisition of mesenchymal features by the cells. This process is associated with a migratory and invasive phenotype [10].
Hyperinsulinemia is a hallmark of insulin resistance. This condition is commonly observed in type 2 diabetes patients and is considered as a primary cause of carcinogenesis. Breast, colon and pancreatic tumors have also been associated with elevated levels of endogenous circulating insulin in non-diabetic patients [11]. The action of insulin is mediated by insulin receptors and/or increasing circulating IGF-1 levels [12]. Up-regulation of insulin and IGF receptors has been associated with cancer cells, which frequently express the fetal isoform A of the insulin receptor (IR-A) [13]. IR-A mediates the mitogenic actions of insulin and IGF-1. Moreover, high levels of insulin have been found to increase the hepatic expression of IGF, thereby stimulating cellular growth [14]. After interactions of IGF and insulin receptors with their ligands, various signaling pathways can be activated through the insulin receptor substrate 1, including the phosphatidylinositol 3 kinase (PI3K)/Akt and Ras/mitogen-activated protein kinase (MAPK) pathways, resulting in cell cycle progression [15]. Additionally, it has been found that insulin can induce the expression and activation of the estrogen receptor, and that an increased sensitivity to estrogens may favor breast carcinogenesis [16]. The actions of high glucose and insulin have also been associated with an increased production of reactive oxygen species (ROS), which are known to mediate various physiological and pathological processes [17, 18]. DNA damage caused by oxidative stress has been found to be closely linked to carcinogenesis [18].
Turnover or degradation of extracellular matrix (ECM) structures is inherent to the acquisition of proliferative, migrative and invasive capacities of cells during cancer development and metastatic spread [19]. The capacity of cells to break down the ECM is, in turn, dependent on the presence of several proteolytic systems, among which the plasminogen activation system. This system participates in the early proteolytic cascades involved in tumor cell invasion where plasminogen activators, such as the urokinase type-plasminogen activator or urokinase (uPA), catalyze the conversion of plasminogen to plasmin. Plasmin can degrade various components of the ECM, such as fibrin and collagen, and participates in the activation of matrix metalloproteinases (MMPs), which are highly efficient in the degradation of the ECM [20–22]. Both plasmin and MMPs degrade ECM proteins, thereby facilitating the proliferation, invasion and metastasis of tumor cells [23]. The complex of uPA with its receptor (uPAR) allows the efficient conversion of plasminogen to plasmin at the cell surface. As such, these proteins are considered key regulators of invasion and metastasis [24–27]. Increased uPA expression has been noted when cancer cells exhibit a more invasive behavior, whereas the expression of uPA and its receptor uPAR have been found to be decreased in cases in which the invasive process is inhibited [28, 29]. In addition, it has been reported that cancer progression can be prevented by the administration of synthetic low-molecular-weight serine protease inhibitors, antibodies directed against uPA or small interfering RNAs targeting uPA [30]. Similarly, it has been found that inhibition of plasmin formation may reduce cancer cell invasion and metastasis [31]. Diabetic conditions such as hyperglycemia and hyperinsulinemia are likely to affect the regulation of the plasminogen activation system and, thus, to contribute to cancer development.
Here, we provide experimental evidence for a role of high glucose and insulin on MDA-MB-231 breast cancer-derived cell proliferation, migration, EMT and invasion. We report that these effects are associated with changes in the expression of members of the plasminogen activation system, possibly mediated by ROS.
2 Materials and methods
2.1 Culture media and reagents
Dulbecco’s Modified Eagle’s Medium (DMEM), bovine insulin, bovine α-casein, bovine plasminogen and N-acetylcysteine (NAC) were purchased from Sigma-Aldrich Chemical Co. (St. Louis, MO, USA). Fetal bovine serum (FBS), antibiotic (penicillin and streptomycin) and trypsin-EDTA solutions were purchased from Invitrogen (Carlsbad, CA, USA). All the other reagents were purchased from Sigma (St. Louis, MO, USA), unless stated otherwise.
2.2 Cell culture and treatment regimens
The MDA-MB-231 breast cancer-derived cells were obtained from the American Type Culture Collection (ATCC; Rockville, MD, USA) and maintained in DMEM containing normal glucose (5.6 mmoles/L), supplemented with 10 % FBS and antibiotics (penicillin 100 U/ml and streptomycin 100 mg/ml) at 37 °C in a humidified 5 % CO2 atmosphere. All experiments were performed with cells from passages 8–15.
The following treatment regimens were applied: a) normal glucose control (NG), 5.6 mmol/L glucose, b) high glucose (HG), 30 mmol/L glucose, c) normal glucose (5.6 mmol/L) plus 40 nmol/L insulin (NG + I) and d) high glucose (30 mmol/L) plus 40 nmol/L insulin (HG + I). Additional assays were performed with the ROS scavenger NAC, 5 mmol/L [32], to assess the role of ROS as mediators of high glucose and insulin action. Treatment with 5.6 mmol/L glucose plus 24.4 mmol/L mannitol was used as an osmolality control.
2.3 Cell viability and growth assays
The percentage of viable cells was determined using a trypan blue exclusion assay according to the procedure described by Freshney [33]. Briefly, 1 × 105 MDA-MB-231 cells were seeded in 24-well culture plates and treated for 48 h with high glucose, insulin and NAC as described above. Upon completion of the incubation, culture media (with floating dead cells) were collected and pooled with the adherent cells that were removed from the plates by trypsinization. Next, the cells were briefly spun down and re-suspended in normal culture medium. Cell viability was assessed by adding 50 μl 0.4 % trypan blue solution in phosphate-buffered saline (PBS) to 50 μl of the cell suspension. After 2 min the number of living cells, which did not retain the dye, was counted using a hemocytometer and compared to the total number of cells (living + dead) to calculate the viability percentage.
The growth of the cell population was evaluated using a 3-(4, 5-dimethylthiazol-2-yl)-2, 5-diphenyltetrazolium bromide (MTT) assay (ATCC, Rockville, MD, USA). The cells were seeded in a 96-well plate at a density of 1 × 104 per well (100 μl) and the above described treatment regimens were applied for 48 h. After this, 10 μl MTT reagent was added to the wells, after which the plate was incubated at 37 °C for 24 h. Next, detergent reagent (100 μl) was added to the wells and mixed to dissolve the dark formazan blue crystals. After the plate was incubated at room temperature in the dark for 2 h to ensure that all crystals were dissolved, the plate was read on a microplate reader (Multiskan EX, Thermo Electron Corporation) at 570 nm. The data are expressed as fold change compared to the control. Given that formazan production from MTT is dependent on the metabolic activity of the cells, this assay depends on cell number, which in our experimental conditions increased in the HG and HG + I treatment regimens. Therefore, the increase in formazan production was measured based on the total number of cells in the assay wells. Similar results were obtained by directly counting the total number of viable cells in the assay wells using a Neubauer chamber (data not shown).
2.4 Detection of reactive oxygen species
For the detection of reactive oxygen species (ROS) cells were seeded in 96-well plates at a density of 1x104 per well (100 μl), and the above described treatment regimens were applied for 48 h. After this, the cells were washed with PBS and incubated in 5 μmol/L of 2′ 7′-dichlorodihydrofluorescein diacetate (DCDF-DA; Molecular Probes, Eugene, OR, USA) in Hank’s solution for 30 min in the dark. The fluorescence emission (530 nm) was measured using a microplate fluorescence reader FRx800 (Bio-Tek Instruments, Inc., Winooski, VT, USA) at 488 nm excitation.
2.5 Cell migration assay
The migratory capacities of the MDA-MB-231 cells were assessed using a scratch-wound migration assay in 24-well plates. After the cells reached 80 to 90 % confluency, the monolayer was scratched with a sterile 1000 μl micropipette tip, washed once with fresh medium, subjected to different treatment regimens and photographed. After 24 h incubation, the cells were stained with Giemsa and photographed again using a CCD camera (Evolution, Media Cybernetics, Rockville, MD, USA) installed on an inverted microscope (Olympus CKX41, Tokyo, Japan). The distance of migration (μm) was measured using Q-Capture Pro7 software (QImaging, Inc., Surrey BC, Canada).
2.6 Cell invasion assay
For invasion assessment 8 μm pore transwells in 24-well plates were used (Greiner Bio-One GMBH, Frickenhausen, Germany). The transwells were coated with 100 μl 10 % Matrigel (BD Biosciences, San Jose, CA, USA) in DMEM and then incubated at 37 °C and 5 % CO2 for 24 h to allow gelation. MDA-MB-231 cells (1 × 105) were seeded on top of the transwell in serum-free DMEM medium using the above described treatment regimens. DMEM was supplemented with 10 % FBS as a chemoattractant in the lower part of the well. After 24 h incubation, the non-invading cells on top of the transwell were removed with a cotton swab. Invading cells that adhered to the lower surface of each filter were fixed with methanol for 10 min and stained with Giemsa for 15 min. The number of cells invading through the Matrigel was imaged and counted in three fields (200× magnification) randomly selected from both central and peripheral regions of the filter using an inverted microscope (CKX41; Olympus, Tokyo, Japan) equipped with a CCD camera (Evolution, Media Cybernetics) in conjunction with Q-Capture Pro7 software (QImaging, Inc., Surrey BC, Canada). The invasiveness of the cells is expressed as the mean number of cells that invaded to the lower side of the filter. The experiments were performed in triplicate.
2.7 RNA extraction and real-time RT-PCR
Total RNA was isolated from samples of 107 cells using Trizol reagent (Invitrogen, Carlsbad, USA). An aliquot of the RNA was dissolved in 100 μl RNAse-free water through incubation at 55 °C for 10 min, and the absorbance was measured at 260 and 280 nm in an UV–VIS Genova spectrophotometer (Jenway, Staffordshire, UK) to determine the concentration and the purity of the RNA.
Total RNA (1 μg) was subjected to M-MuLV Reverse Transcriptase (Fermentas, Thermo-Fisher Scientific, Vilnius, Lithuania) for 60 min at 37 °C using random primers. Then, the enzyme was inactivated at 70 °C for 15 min and real-time PCR was performed using a PCR Rotor Gene Real-Time Apparatus 2004 (Corbett Research, Cambridgeshire, UK) using a Maxima SYBR Green/ROX Master Mix kit (Fermentas, Thermo-Fisher Scientific) following the instructions of the supplier. The following specific primer pairs were used: uPA, sense 5′-CGCTGCTCCCACATTGGCTAAG-3′ and antisense 5′-TGTGCATGGGTGAAGGGAGAGC-3′ [34]; uPAR, sense 5′-CAACGAGGGCCCAATCCT-3′ and antisense 5′-GTAACACTGGCGGCCATTCT-3′ [35]; PAI-1, sense 5′-TGCTGGTGAATGCCCTCTACT-3′ and antisense 5′-CGGTCATTCCCAGGTTCTCTA-3′ [36] and ribosomal protein S18 (RPS18) as a housekeeping (control) gene, sense 5′-GATATGCTCATGTGGTGTTG-3′ and antisense 5′-AATCTTCTTCAGTCGCTCCA-3′ [36]. The amplification reaction included 40 cycles of heat denaturation at 95 °C for 30 s, followed by annealing for 30 s at 51 °C (RPS18), 54 °C (PAI-1), 58 °C (uPAR) or 60 °C (uPA) and extension for 30 s at 72 °C. After the final cycle, the temperature was maintained at 72 °C for 10 min. The relative expression of the genes was determined by the comparative C T method (2-ΔΔCT) using their respective baselines as reference points. The results were expressed as fold change compared to the glucose 5.6 mmol/L group.
2.8 Protein isolation and Western blotting
After treatment, cells were washed twice with PBS, detached using 20 mmol/L EDTA in PBS for approximately 5 min at 37 °C and centrifuged at 1200 g for 5 min. The resulting cell pellets were washed twice with PBS and lysed in ice-cold Pierce RIPA Buffer (Cat. 89900, Thermo Scientific, Rockford, Il, USA) containing a protease inhibitor cocktail (Cat. I3786, Sigma-Aldrich). After an aliquot was obtained for protein quantification by Bradford technique [37] the lysates were stored at −70 ° C until use. The protein samples were mixed with Laemmli buffer, subjected to 10 % sodium dodecyl sulphate-polyacrylamide gel electrophoresis (SDS-PAGE) and transferred to polyvinylidene difluoride (PVDF) membranes (0.8 mA/cm2, 2 h) in 25 mmol/L Tris buffer, 192 mmol/L glycine and 20 % methanol. After blocking nonspecific binding sites with Tris-buffered-saline (TBS) supplemented with 5 % BSA, the membranes were incubated overnight at 4 °C with primary antibodies directed against uPA (H-140), PAI-1, uPAR (10G7), E-cadherin (67A4), β-Actin (C-2) (Santa Cruz Biotechnology, Santa Cruz, CA, USA), Vimentin (clone V9, Dako, Glostrup, Denmark) and Fibronectin (clone FN15, Sigma-Aldrich) in TBS-Tween-20 0.1 % containing 1 % BSA and washed three times with the same buffer. Antibody binding was detected using a secondary antibody labeled with horseradish peroxidase (HRP) in conjunction with a commercial chemiluminescence kit (Clarity Western ECL substrate, Bio-Rad, Hercules, CA, USA) following the supplier’s instructions. Blot image acquisition was performed using a C-Digit Blot Scanner (LI-COR Biotechnology, Lincoln, NE, USA). The band intensities were calculated after background subtraction and normalization to β-Actin using Image Studio 4.0 software (LI-COR Biotechnology).
2.9 Gel zymography
Cell lysates and conditioned media were harvested from cells subjected to the corresponding treatment regimens in serum-free medium. The conditioned media were centrifuged for 5 min at 1500 g to remove cellular material. Next, uPA activity was determined by substrate gel zymography [22, 38]. To this end, aliquots of cell lysates or conditioned media containing 20 μg proteins were resolved by non-denaturing electrophoresis in 10 % SDS-PAGE containing 1 mg/ml bovine α-casein and 10 μg/ml bovine plasminogen. The gels were run at 8 °C. After electrophoresis, the gels were washed twice for 30 min in 2 % (v/v) Triton X-100 to remove SDS and then incubated for 24 h at 37 °C in 100 mmol/L glycine and 10 mmol/L EDTA, pH 8.3. Next, the gels were stained overnight with Coomassie Brilliant Blue R-250 0.5 % in 45 % methanol and 10 % acetic acid and destained in the same solution without dye. Plasminogen-dependent caseinolytic proteins were detected as clear zones of degradation against a blue background. Gels exclusively co-polymerized with casein were used to exclude non-specific plasminogen caseinolytic bands. The zymograms were imaged using a G:Box Gene Documentation System (Syngene, Frederick, MD, USA) and zones of enzyme activity were quantified as integrated density by densitometry analysis using Image Studio 4.0 software (LI-COR Biotechnology, Lincoln, NE, USA).
2.10 Statistical analyses
Values are expressed as the mean ± standard deviation (SD). The results represent three different experiments. All the data were analyzed using the NCSS (Number Cruncher Statistical System) 2007 Statistical Software Package Program (Utah, USA). Statistical comparison was performed using one-way analysis of variance (ANOVA) followed by Tukey’s test.
3 Results
3.1 High glucose and insulin induce breast cancer cell proliferation and oxidative stress
To assess the effect of high glucose and insulin on the viability and proliferative capacity of the breast cancer-derived MDA-MB-231 cells, the percentage of living cells in relation to the total number of cells (living + dead) and the total cell numbers in the assay wells were evaluated using trypan blue exclusion and MTT assays, respectively. In accordance with previously reported data [39], we found that high glucose had a growth promoting effect, as the MTT assay revealed a significant increase in the cell population (1.9 times). This effect was further enhanced (2.7 times) compared to the respective controls when high glucose was used in conjunction with insulin (Fig. 1a).
Induction of MDA-MB-231 cell proliferation by high glucose and insulin is mediated by ROS. MDA-MB-231 cells were incubated with 5.6 mmol/L glucose (normal glucose, NG) or 30 mmol/L glucose (high glucose, HG) for 48 h. Some cultures were treated simultaneously with 40 nmol/L insulin (I) or 5 mmol/L NAC. The total number of viable cells (cell population) was assessed by MTT assay (a), the viability percentage was assessed by trypan blue exclusion assays (b) and ROS production was assessed using 2′ 7′-dichlorodihydrofluorescein diacetate (DCDF-DA) (c). Data in a and c represent the percent changes, with the NG group set at 100 %. In b the percentages of viable cells are shown as means ± SD of 3 independent experiments. * p ≤ 0.01 compared to NG, ¥ p ≤ 0.01 compared to the respective treatment without insulin and p ≤ 0.05 compared to the respective NAC treatment.
To investigate whether the action of high glucose and insulin in MDA-MB-231 cells was associated with the generation of ROS, we next set out to assess ROS levels and to study the effect of the ROS scavenger NAC on the actions of high glucose and insulin. In accordance with the notion that ROS can serve as mediators of high glucose or insulin, ROS levels were found to be increased after treatment with high glucose (1.36 times). This effect was further enhanced by insulin, reaching a value 1.8 times higher than the control (NG) group (Fig. 1c). The increase in ROS induced by high glucose or high glucose plus insulin could partially be prevented by the addition of 5 mmol/L NAC (44 and 31.8 %, respectively). From these data we conclude that high glucose and insulin can significantly increase ROS levels and that NAC treatment can significantly reduce these levels. Additionally, we found that NAC attenuated the increase in cell population induced by high glucose alone or its combination with insulin (24.2 and 31 %, respectively; Fig. 1a).
Based on the trypan blue dye exclusion test, we found that the percentage of viable cells was high in all experimental conditions (86.7 to 100 %). A slight decrease (10 %) in viability was noted in cells treated with high glucose and high glucose plus insulin (14 %) compared to the controls (Fig. 1b). This decrease could partially be prevented by NAC, resulting in viability values of 94.80 ± 0.32 % for cells treated with high glucose and NAC and 90.40 ± 2.11 % for cells treated with high glucose plus insulin and NAC (Fig. 1b). The addition of 24.6 mmol/L mannitol to the media containing 5.6 mmol/L D-glucose did not affect the viability or proliferative capacity of the cells (data not shown).
The slight decrease in cell viability induced by high glucose or high glucose plus insulin is indicative of a low proportion of cell death concomitant with a higher rate of cell proliferation under these circumstances. We conclude that high glucose- or high glucose plus insulin-induced increases in cell population may at least partially be mediated by a mechanism involving oxidative stress.
3.2 Enhanced MDA-MB-231 cell migration and invasion by high glucose and insulin are mediated by ROS
Using cell migration assays, we found that high glucose enhanced the migration of MDA-MB-231 cells (1.3 times) and that treatment with high glucose plus insulin led to a further increase in cell migration (1.6 times). In addition, we found that the use of NAC did not affect the migration of cells treated with high glucose, but reduced the increase in migration induced by treatment with high glucose plus insulin by 33 % (Fig. 2). In Fig. 3 it is shown that the invasive capacity of cells treated with normal glucose is minimal, and that this capacity was only slightly increased by insulin treatment (2.55 ± 0.31 times). The invasiveness was, however, clearly enhanced in cells treated with high glucose (36.83 ± 3.03 times) and high glucose plus insulin (46.16 ± 2.57 times). Subsequent treatment with NAC led to a significant attenuation of these effects (Fig. 3). The addition of 24.6 mmol/L mannitol to the media containing 5.6 mmol/L D-glucose did not affect the migrative and invasive capacities of the cells (data not shown).
Enhancement of MDA-MB-231 cell migration by high glucose and insulin is mediated by ROS. MDA-MB-231 cells were grown in the presence of 5.6 mmol/L glucose (NG) or 30 mmol/L glucose (HG) for 24 h. Some cultures were treated simultaneously with 40 nmol/L insulin (I) or 5 mmol/L NAC. Migration was evaluated by wound healing assay. The upper panels show representative images of MDA-MB-231 cells taken by phase-contrast microscopy (0 h) or after Giemsa staining (24 h) after scratching. Lines indicate the distance not covered by invading cells. The lower panel represents the distance of migration. Data are shown as the means ± SD of 3 independent experiments, * p ≤ 0.05 compared to NG, ¥ p ≤ 0.005 compared to the respective treatment without insulin and p ≤ 0.01 compared to the respective NAC treatment
Enhancement of MDA-MB-231 cell invasion by high glucose and insulin is mediated by ROS. MDA-MB-231 cells were grown in the presence of 5.6 mmol/L glucose (NG) or 30 mmol/L glucose (HG) for 24 h. Some cultures were treated simultaneously with 40 nmol/L insulin (I) or 5 mmol/L NAC. Cell invasion was evaluated by transwell matrigel assay. The upper panels show representative microscopic images and the lower panel represents the number of invading cells by field. Data are shown as the means ± SD of 3 independent experiments, * p ≤ 0.05 compared to the NG group, ¥ p ≤ 0.005 compared to the respective treatment without insulin and p ≤ 0.01 compared to the respective NAC treatment
From these combined data we conclude that the induction of MDA-MB-231 cell invasion by high glucose and high glucose plus insulin may be mediated by ROS, in a manner similar to the promigratory activity of insulin in a high-glucose environment.
3.3 Induction of a mesenchymal phenotype by high glucose and insulin is mediated by ROS
Given that enhanced cell migration and invasion are known to be associated with epithelial-mesenchymal transition (EMT), we next set out to assess changes in E-cadherin, vimentin and fibronectin expression levels. The epithelial marker E-cadherin was not detected in MDA-MB-231 cells grown in the presence of normal glucose, high glucose or high glucose plus insulin. However, E-cadherin was found to be present in MCF-7 cells, which we used as a positive control (Fig. 4a). This observation is in accordance with literature data indicating that MDA-MB-231 cells have lost at least some of their epithelial markers [40]. On the other hand, we found that high glucose treatment led to increases in the expression levels of some mesenchymal markers, including vimentin and fibronectin, which were found to be increased even further by a combined treatment with high glucose and insulin (Fig. 4a, c and d). Subsequent NAC treatment was found to slightly induce the expression of E-cadherin under normal glucose conditions, and to attenuate the increased vimentin and fibronectin levels induced by high glucose and high glucose plus insulin treatments (Fig. 4). In addition, we found that insulin did not affect the EMT markers tested in cells grown in 5.6 mmol/L glucose (Fig. 4). The E-cadherin, vimentin and fibronectin protein levels were not found to be affected in the osmolality control with mannitol (data not shown).
Enhancement of EMT in MDA-MB-231 cells by high glucose and insulin is mediated by ROS. MDA-MB-231 cells were incubated with 5.6 mmol/L glucose (NG) or 30 mmol/L glucose (HG) for 48 h. Some cultures were treated simultaneously with 40 nmol/L insulin (I) or 5 mmol/L NAC. Cell lysates were analyzed by Western blotting (a), followed by densitometry analysis for E-cadherin (b), vimentin (c) and fibronectin (d). Results are expressed as the ratio of E-cadherin, vimentin or fibronectin to β-actin. Representative blots and mean ± SD of 3 independent experiments are shown, * p ≤ 0.05 compared to NG group, ¥ p ≤ 0.05 compared to the respective treatment without insulin and p ≤ 0.05 compared to the respective NAC treatment
From these data we conclude that the increased cell migration and invasion induced by high glucose and insulin are associated with higher expression levels of mesenchymal markers (Figs. 2, 3 and 4) and that NAC can undo the effects of high glucose and high glucose plus insulin on the vimentin and fibronectin expression levels in a manner similar to the prevention of ROS generation by NAC under the same conditions (Figs. 1 and 4).
3.4 Increased uPA, uPAR and PAI-1 expression induced by high glucose and insulin is mediated by ROS
Using real-time RT-PCR and Western blotting assays we found that high glucose and high glucose plus insulin treatments led to increased uPA, uPAR and PAI-1 expression levels at both the mRNA and protein levels in MDA-MB-231 cells (Figs. 5 and 6).
Increased uPA, uPAR and PAI-1 mRNA levels induced by high glucose and insulin are mediated by ROS. MDA-MB-231 cells were grown in the presence of 5.6 mmol/L glucose (NG) or 30 mmol/L glucose (HG) for 48 h. Some cultures were treated simultaneously with 40 nmol/L insulin (I) or 5 mmol/L NAC. mRNA expression levels of uPA (a), uPAR (b) and PAI-1 (c) were evaluated by real-time RT-PCR. The relative mRNA expression levels of uPA, uPAR or PAI-1 were calculated using ribosomal protein S18 (RPS18) as a reference and were expressed as fold changes in relation to glucose 5.6 mmol/L treatment. The means ± SD of 3 independent experiments are shown, * p ≤ 0.05 compared to NG, ¥ p ≤ 0.05 compared to the respective treatment without insulin and p ≤ 0.05 compared to the respective NAC treatment
Increased uPA, uPAR and PAI-1 protein levels induced by high glucose and insulin are mediated by ROS. MDA-MB-231 cells were grown in the presence of 5.6 mmol/L glucose (NG) or 30 mmol/L glucose (HG) for 48 h. Some cultures were treated simultaneously with 40 nmol/L insulin (I) or 5 mmol/L NAC. Cell lysates were analyzed by Western blotting (a), followed by densitometry analysis for pro-uPA (b), chain A of active uPA (c), uPAR (d) and PAI-1 (e). Results are expressed as the ratio of uPA, uPAR or PAI-1 to β-Actin. Representative blots and means ± SD of 3 independent experiments are shown, * p ≤ 0.05 compared to NG, ¥ p ≤ 0.05 compared to the respective treatment without insulin and p ≤ 0.05 compared to the respective NAC treatment
High glucose treatment induced an increase in the uPA mRNA expression level (2.26 ± 0.39 times), and this effect was further enhanced after combination with insulin (3.61 ± 0.75 times). These effects could partially be reduced by NAC (52 and 49 %, respectively) (Fig. 5a). We also found that high glucose treatment induced a relatively lower increase in the uPAR mRNA expression level (1.46 ± 0.73 times), and no significant additional effect was seen when the cells were treated with high glucose and insulin (2.34 ± 0.63 times). NAC was found to partially reduce the effects of high glucose and high glucose plus insulin by 62 and 48 %, respectively (Fig. 5b). Treatment with 30 mmol/L glucose was subsequently found to induce an increase in the PAI-1 mRNA expression level (2.42 ± 0.41 times). The simultaneous treatment with glucose and insulin did not significantly modify the effect of high glucose (3.34 ± 0.53 times). In addition, we found that NAC partially reduced the effects of high glucose and high glucose plus insulin by 59 and 46 %, respectively (Fig. 5c). In cells grown in the presence of 5.6 mmol/L glucose, insulin slightly increased the uPA mRNA level by 1.38-fold (Fig. 5a), whereas mannitol had no effect on the uPA, uPAR and PAI-1 mRNAs levels (data not shown).
3.5 High glucose and insulin induce increased uPA, uPAR and PAI-1 protein levels through a ROS-mediated mechanism
Through Western blot analysis we were able to detect the precursor of single chain pro-uPA and the A chain of the two-chain active uPA (Fig. 6a). Pro-uPA showed an increase in expression of 1.8 times in cells grown with high glucose, and this effect was potentiated in the presence of high glucose plus insulin, reaching an increase of 2.46-fold compared to the normal glucose group. NAC attenuated the effect of high glucose and high glucose plus insulin by 45.7 and 49.35 %, respectively (Fig. 6a and b). The A chain of active uPA increased most profoundly in cells cultured with high glucose and high glucose plus insulin (3.7-fold and 5-fold, respectively). NAC treatment attenuated the increase in the active protein by 63 and 53.5 % upon induction by high glucose and high glucose plus insulin, respectively (Fig. 6a and c). We found that the expression of uPAR increased 1.9-fold in cells grown with high glucose and 2.3-fold in cells grown with high glucose plus insulin. NAC reduced the increase in uPAR induced by high glucose by 58 and by 36 % in cells treated with high glucose plus insulin (Fig. 6a and d). We also found that PAI-1 exhibited a similar trend to the proteins previously analyzed, with a 2-fold increase in cells treated with high glucose and a 2.5-fold increase in cells treated with high glucose plus insulin. Similarly, the effect of high glucose was reduced by NAC by 38.5 %, and the effect of high glucose plus insulin was reduced by 43 % (Fig. 6a and e). Insulin only slightly increased the PAI-1 protein level in cells grown in normal glucose by 1.2-fold (Fig. 6a and e), whereas mannitol had no effect on the uPA, uPAR and PAI-1 protein expression levels (data not shown).
Together, the RT-PCR and Western blot data indicate that the uPA, uPAR and PAI-1 expression levels are up-regulated in response to high glucose and insulin treatment, and that NAC can significantly reduce the effects of high glucose and high glucose plus insulin on the uPA, active uPA, uPAR and PAI-1 mRNA and protein expression levels, in a manner that is similar to the prevention of ROS generation by NAC under the same conditions (Figs. 1, 5 and 6).
3.6 Increased uPA activity induced by high glucose and insulin is mediated by ROS
Next, we set out to assess the proteolytic activity of uPA in cell lysates and conditioned media obtained from MDA-MB-231 cells using casein plus plasminogen gel zymography (Fig. 7). By doing so, we found that treatment of cells with high glucose increased the uPA activity by 2-fold, whereas after treatment with high glucose plus insulin the increase was 1.8-fold. Therefore, we conclude that the uPA activity was not further increased by insulin. After NAC treatment we observed a clear decrease in uPA activity in both high glucose and glucose plus insulin treated cells, reaching values lower than the normal glucose control (between 22 and 35 % of the activity presented by the normal glucose control group). In the conditioned media in the absence of serum, treatment with high glucose alone or high glucose plus insulin induced a similar increase in uPA activity (1.4 and 1.38 times, respectively; Fig. 7b), whereas NAC again induced a complete inhibition in uPA activity in the conditioned media under all conditions, including normal glucose treatment (Fig. 7c).
Stimulation of uPA activity by high glucose and insulin is mediated by ROS. MDA-MB-231 cells were grown in the presence of 5.6 mmol/L glucose (NG) or 30 mmol/L glucose (HG) in serum-free medium for 48 h. Some cultures were treated simultaneously with 40 nmol/L insulin (I) or 5 mmol/L NAC. The activity of uPA was evaluated by plasminogen-casein zymography (a) and densitometry analysis of cell lysates (b) or conditioned media (c). Representative zymograms and means ± SD of 3 independent experiments are shown. NAC decreases the activity of uPA in all treatments. * p ≤ 0.05 compared to NG, ¥ p ≤ 0.05 compared to the respective treatment without insulin and p ≤ 0.05 compared to the respective NAC treatment
4 Discussion
It is well-recognized that hyperglycemia and hyperinsulinemia can promote the development of several cancers, including breast cancer. However, the exact mechanisms involved still remain to be elucidated [4–6, 18]. Here, we show that high concentrations of glucose and insulin can promote the proliferation, migration and invasion of triple-negative MDA-MB-231 breast cancer-derived cells. These actions were found to be mediated by ROS and to be associated with the acquisition of a more pronounced mesenchymal phenotype (EMT transition), as well as with an enhanced expression of components of the proteolytic system of plasminogen activation (i.e., uPA, uPAR and PAI-1).
Previously, mitogenic, pro-migratory and pro-invasive effects of high glucose and insulin have been observed in both normal [17] and cancer cells [7, 15, 18, 41, 42]. In accordance with our results, high glucose and insulin have been found to increase the proliferation of MDA-MB-231 cells [39, 43] and to stimulate the migratory ability of other breast cancer-derived cells (MCF7 and MDA-MB-468) [41]. The increases in cell population induced by high glucose and insulin can be attributed to increased cell proliferation. After glucose or glucose plus insulin treatment, increased numbers of cells were detected by the MTT assay, whereas only slight decreases in the percentages of living cells were noted. Cell proliferation can be promoted by a high availability and consumption of glucose and a pro-mitogenic action of insulin [44]. Glucose metabolism in cancer cells leads to the synthesis of nucleotides, lipids and amino acids precursors [18] and, together with insulin, these conditions may promote DNA synthesis [45] and proliferation [39, 41, 43, 46]. The action of insulin in tumor tissues appears to be important for cell survival and mitogenesis rather than for glucose transport, since it has been found that the transport of glucose can also be affected by insulin-independent mechanisms [47]. Cell proliferation can also be stimulated by uPA and PAI-1, which have in the past been associated with the proliferation of tumor cells, including MDA-MB-231 cells [48, 49]. Here, we found that the expression of these proteins was increased by high glucose and high glucose plus insulin treatment in MDA-MB-231 cells. uPA induces proliferation through interaction of its epidermal growth factor-like domain with uPAR [49], whereas PAI-1 has been found to stimulate progression from the Go to the G1 phase of the cell cycle [48, 50]. The exact roles of uPA and PAI-1 in proliferation induction by high glucose and insulin, however, still remain to be elucidated.
In the past, an icrease in migration induced by high glucose and high glucose plus insulin in MDA-MB-468 breast cancer-derived cells has been reported using a 3D collagen matrix assay [41, 46]. Here, we found similar effects of these treatments in MDA-MB-231 cells using a scratch-wound assay. Additionally, we found that high glucose and insulin increased the invasive activity of MDA-MB-231 cells using a matrigel-transwell assay. The observed enhanced migration and invasion of MDA-MB-231 cells likely involves EMT, based on the observation that these cells incubated with high glucose and high glucose plus insulin exhibited increased vimentin and fibronectin protein expression levels. Up-regulation of these proteins is an indication of EMT and has been correlated with metastasis, also in non-invasive MCF-7 breast cancer-derived cells [40]. Additionally, it was previously found that high glucose levels can activate EMT-associated changes in HK-2 human renal proximal tubular epithelial cells [10]. A role for insulin in the activation of EMT has not been established yet, but it has been found that the insulin receptor IR-A may mediate EMT induced by IGF [51]. IR-A is abundantly expressed in cancer cells and it has been reported that insulin can induce the migratory activity of HepG2 hepatoblastoma cells when these cells are induced to express IR-A [52]. Here, we show for the first time that EMT can be enhanced by insulin in MDA-MB-237 cells grown in a high-glucose environment. Our observations suggest that high glucose and insulin can promote the EMT program in MDA-MB-231 cells.
In order to unravel the mechanism underlying high glucose and insulin-dependent MDA-MB-231 cell migration and invasion, the plasminogen system was studied. The results obtained are consistent with previously reported associations between uPA, uPAR and PAI-1 over-expression and increased invasiveness and metastasis of breast cancer cells, as well as poor prognoses in humans and experimental models [48, 53–59]. These proteins are commonly over-expressed in primary breast cancers and have also been found to be up-regulated in several metastatic cancer cell types [48, 55, 56]. uPA primarily promotes cancer invasion through the conversion of plasminogen to plasmin. This reaction is more efficient when uPA is bound to uPAR at the cell surface. Plasmin is known to contribute to ECM degradation and the activation of various MMPs and growth factors [59, 60]. We observed increased uPA, uPAR and PAI-1 mRNA and protein levels after high glucose and high glucose plus insulin treatments. The coordinated actions of these proteins potentially allow ECM remodeling, thereby promoting increased migratory and invasive activities. Based on its ability to inhibit uPA activity, PAI-1 may suppress cancer progression. Recent studies, however, suggest that PAI-1, at the levels found in tumor extracts, may be involved in mediating cancer progression [48]. It has been found that PAI-1 may e.g. favor cancer cell proliferation and angiogenesis and/or prevent apoptosis [50, 59, 61]. Indeed, direct evidence for a role for PAI-1 in cancer progression was recently obtained when a specific inhibitor of PAI-1 was found to block angiogenesis and tumor progression in an animal model [62]. Additionally, it has been proposed that over-expression of uPAR [56] and the interaction of uPA-uPAR with matrix-bound PAI-1 may lead to amoeboid cell behavior and, as such, to increased tumor cell migration and invasion [63]. Coordinated expression of proteolytic enzymes, their inhibitors and receptors provide a mechanism for fine tuning focal proteolysis to facilitate matrix restructuring and cell motility during metastasis. Interestingly, the active form of uPA was found to be increased in cells cultured in the presence of high glucose as well as in the presence of high glucose plus insulin, indicating that uPA was activated in these pro-migratory and pro-invasive conditions, despite the up-regulation of PAI-1 [48]. These results suggest that the pro-migratory and pro-invasive actions of high glucose and insulin are associated with the activation of uPA despite PAI-1 over-expression. We conclude that uPA, uPAR and PAI-1 are involved in the pro-migratory and pro-invasive activities of high glucose and insulin and that, as such, these proteins may be involved in enhanced tumor development in patients with diabetes. The effect of insulin on the expression of uPA, uPAR and PAI-1 has so far poorly been studied. PAI-1 has been found to be up-regulated by insulin in adipocytes [64] and in some cancer-derived cell lines in culture [65–67]. PAI-1 levels have also been found to be elevated in patients with diabetes type 2, and this elevation has been correlated with complications of diabetes. Therefore, PAI-1 likely mediates carcinogenesis and tumor cell invasion induced by hyperglycemia and hyperinsulinemia. Insulin does not induce uPA activity in a hormone-responsive, noninvasive, epithelial cell line derived from a rat breast adenocarcinoma [68]. Although, we did not find any literature report dealing with the action of insulin on uPA and uPAR, indirect evidence indicates that insulin may up-regulate these proteins given the observation that IGF-1 acts through similar receptors and signaling pathways [69, 70], thereby inducing uPA in human breast cancer-derived MDA-MB-231 cells [54]. On the other hand, the Ets2 transcription factor has been found to be up-regulated by insulin in mouse mammary carcinoma-derived Mvt-1 cells [70] and to activate the uPA gene promoter in response to EGF in invasive breast cancer-derived cell lines [71].
Our findings suggest that the increased cell proliferation and invasion induced by high glucose and insulin in a high-glucose environment in MDA-MB-231 cells are mediated by ROS, and that the up-regulation of uPA, uPAR and PAI-1 mediates the effects of ROS. Both treatment regimens induced increased ROS levels, which could be attenuated by NAC. Additionally, we found that NAC significantly attenuated high glucose and high glucose plus insulin-induced increases in cell proliferation and invasion, and the up-regulation of mesenchymal markers, uPA and PAI-1. High glucose and insulin are known to promote increased ROS generation in normal and cancer cells in culture [32, 72–74] and ROS is known to mediate the proliferation and migration induced by insulin in various cells [17]. Several researchers have reported convincing evidence that ROS can regulate the plasminogen system, and can act as mediators of the action of high glucose. ROS have been found to induce the up-regulation of uPA [32, 75], uPAR [76] and PAI-1 [65, 77] and to mediate the up-regulation of uPA induced by hepatocyte growth factor in human gastric cancer cells [32]. Additionally, ROS has been found to mediate the up-regulation of PAI-1 induced by high glucose in rat white adipocytes [77] and in mesangial cells [65]. Insulin has been found to induce or potentiate the activation of PAI-1 transcription associated with oxidative stress [65–67, 78]. In this context, anti-oxidants may provide protection against tumor invasion and metastasis both in vivo and in vitro. In particular, it has been found that NAC may inhibit the invasiveness of endothelial cells associated with increased expression of uPAR and increased ROS production [76], as well as the proliferation [79] and invasiveness of some cancer-derived cells in vitro [80]. It is noteworthy that the action of insulin was only clearly observed in the presence of high glucose. It is possible that insulin and high glucose act synergistically on the proliferation and invasion of MDA-MB-231 cells, as well as the expression of mesenchymal markers, uPA, uPAR and PAI-1 in these cells. A similar synergistic effect was observed on albumin permeability in cultured rat podocytes [81]. The mechanism by which high glucose sensitizes cells to the insulin action remains to be elucidated.
In conclusion, we found that high glucose and high glucose plus insulin induced proliferative, migrative and invasive activities in MDA-MB-231 cells associated with increased ROS generation, EMT, up-regulation of uPA, uPAR and PAI-1 expression and increased uPA activity. These effects could be attenuated by NAC, indicating that the plasminogen activation system is regulated by ROS during the acquisition of a more invasive phenotype. Anti-oxidants may prevent the acquisition of an invasive cancer phenotype in conditions of hyperglycemia and hyperinsulinemia.
References
A. Jemal, F. Bray, M.M. Center, J. Ferlay, E. Ward, D. Forman, Global cancer statistics. CA Cancer J. Clin. 61, 69–90 (2011)
Y. Chavarría-Guerra, C. Villarreal-Garza, P.E. Liedke, F. Knaul, A. Mohar, D.M. Finkelstein, P.E. Goss, Breast cancer in Mexico: a growing challenge to health and the health system. Lancet Oncol 13, 335–343 (2012)
A.H. Wu, M.C. Yu, C.C. Tseng, F.Z. Stanczyk, M.C. Pike, Diabetes and risk of breast cancer in Asian-American women. Carcinogenesis 28, 1561–1566 (2007)
G. Gezgen, E.C. Roach, M.C. Kizilarslanoglu, I. Petekkaya, K. Altundag, Metabolic syndrome and breast cancer: an overview. J. Buon. 17, 223–229 (2012)
R.L. Westley, F.E. May, A twenty-first century cancer epidemic caused by obesity: the involvement of insulin, diabetes, and insulin-like growth factors. Int. J. Endocrinol. 2013, 632461 (2013)
A.A. Onitilo, R.V. Stankowski, R.L. Berg, J.M. Engel, I. Glurich, G.M. Williams, S.A. Doi, Breast cancer incidence before and after diagnosis of type 2 diabetes mellitus in women: increased risk in the prediabetes phase. Eur. J. Cancer Prev. 23, 76–83 (2014)
M.E. Beckner, M.L. Stracke, L.A. Liotta, E. Schiffmann, Glycolysis as primary energy source in tumor cell chemotaxis. J. Natl. Cancer Inst. 82, 1836–1840 (1990)
K. Goetze, C.G. Fabian, A. Siebers, L. Binz, D. Faber, S. Indraccolo, G. Nardo, U.G.A. Sattler, W. Mueller-Klieser, Manipulation of tumor metabolism for therapeutic approaches: ovarian cancer-derived cell lines as a model system. Cell. Oncol. 38, 377–385 (2015)
C. Villarreal-Garza, R. Shaw-Dulin, F. Lara-Medina, L. Bacon, D. Rivera, L. Urzua, C. Aguila, R. Ramirez-Morales, J. Santamaria, E. Bargallo, A. Mohar, L.A. Herrera, Impact of diabetes and hyperglycemia on survival in advanced breast cancer patients. Exp. Diabetes Res. 2012, 732027 (2012)
D. Li, T. Zhao, J. Meng, Y. Jing, F. Jia, P. He, Procyanidin B2 inhibits high glucose induced epithelial mesenchymal transition in HK 2 human renal proximal tubular epithelial cells. Mol. Med. Rep. 12, 8148–8154 (2015). doi:10.3892/mmr.2015.4445
I.R. Hsu, S.P. Kim, M. Kabir, R.N. Bergman, Metabolic syndrome, hyperinsulinemia, and cancer. Am. J. Clin. Nutr. 86, s867–871 (2007)
D.L. Roberts, C. Dive, A.G. Renehan, Biological mechanisms linking obesity and cancer risk: new perspectives. Annu. Rev. Med. 61, 301–316 (2010)
P. Singh, J.M. Alex, F. Bast, Insulin receptor (IR) and insulin-like growth factor receptor 1 (IGF-1R) signaling systems: novel treatment strategies for cancer. Med. Oncol. 31, 805–818 (2014)
S. Djiogue, A.H. Nwabo Kamdje, L. Vecchio, M.J. Kipanyula, M. Farahna, Y. Aldebasi, P.F. Seke Etet, Insulin resistance and cancer: the role of insulin and IGFs. Endocr. Relat. Cancer 20, R1–R17 (2013)
D.P. Rose, L. Vona-Davis, The cellular and molecular mechanisms by which insulin influences breast cancer risk and progression. Endocr. Relat. Cancer 19, R225–241 (2012)
K.H. Joung, J.W. Jeong, B.J. Ku, The association between type 2 diabetes mellitus and women cancer: the epidemiological evidences and putative mechanisms. Biomed. Res. Int. 2015, 920618–920630 (2015)
S. Abhijit, R. Bhaskaran, A. Narayanasamy, A. Chakroborty, N. Manickam, M. Dixit, V. Mohan, M. Balasubramanyam, Hyperinsulinemia-induced vascular smooth muscle cell (VSMC) migration and proliferation is mediated by converging mechanisms of mitochondrial dysfunction and oxidative stress. Mol. Cell. Biochem. 373, 95–105 (2013)
S. Joshi, M. Liu, N. Turner, Diabetes and its link with cancer: providing the fuel and spark to launch an aggressive growth regime. Biomed. Res. Int. 2015, 390863 (2015)
J.A. Carnegie, O. Cabaca, Extracellular matrix composition and resilience: two parameters that influence the in vitro migration and morphology of rat inner cell mass-derived cells. Biol. Reprod. 48, 287–299 (1993)
F. Mwale, E. Tchetina, C.W. Wu, A.R. Poole, The assembly and remodeling of the extracellular matrix in the growth plate in relationship to mineral deposition and cellular hypertrophy: an in situ study of collagens II and IX and proteoglycan. J. Bone Miner. Res. 17, 275–283 (2002)
L.A. Davidson, R. Keller, D.W. DeSimone, Assembly and remodeling of the fibrillar fibronectin extracellular matrix during gastrulation and neurulation in Xenopus laevis. Dev. Dyn. 231, 888–895 (2004)
M.G. Martinez-Hernández, L.A. Baiza-Gutman, A. Castillo-Trápala, D.R. Armant, Regulation of proteinases during mouse peri-implantation development: urokinase-type plasminogen activator expression and cross-talk with matrix metalloproteinase 9. Reproduction 141, 227–239 (2011)
G. Konecny, M. Untch, A. Pihan, R. Kimmig, M. Gropp, P. Stieber, H. Hepp, D. Slamon, M. Pegram, Association of urokinase-type plasminogen activator and its inhibitor with disease progression and prognosis in ovarian cancer. Clin. Cancer Res. 7, 1743–1749 (2001)
B. Märkl, I. Renk, D.V. Oruzio, H. Jähnig, G. Schenkirsch, C. Schöler, W. Ehret, H.M. Arnholdt, M. Anthuber, H. Spatz, Tumour budding, uPA and PAI-1 are associated with aggressive behavior in colon cancer. J. Surg. Oncol. 102, 235–41 (2010)
K. Malinowsky, C. Böllner, S. Hipp, D. Berg, M. Schmitt, K.F. Becker, uPA and PAI-1 analysis from fixed tissues - new perspectives for a known set of predictive markers. Curr. Med. Chem. 17, 4370–4377 (2010)
J. Jankun, J. Yang, H. Zheng, F.Q. Han, A. Al-Senaidy, E. Skrzypczak-Jankun, Remarkable extension of PAI-1 half-life surprisingly brings no changes to its structure. Int. J. Mol. Med. 29, 61–64 (2012)
L. Tang, X. Han, The urokinase plasminogen activator system in breast cancer invasion and metastasis. Biomed. Pharmacother. 67, 179–182 (2013)
Y. Sun, X. Wang, Q. Zhou, Y. Lu, H. Zhang, Q. Chen, M. Zhao, S. Su, Inhibitory effect of emodin on migration, invasion and metastasis of human breast cancer MDA-MB-231 cells in vitro and in vivo. Oncol. Rep. 33, 338–346 (2015)
F. Dkhissi, H. Lu, C. Soria, P. Opolon, F. Griscelli, H. Liu, P. Khattar, Z. Mishal, M. Perricaudet, H. Li, Endostatin exhibits a direct antitumor effect in addition to its antiangiogenic activity in colon cancer cells. Hum. Gene Ther. 14, 997–1008 (2003)
M. Schmitt, N. Harbeck, N. Brünner, F. Jänicke, C. Meisner, B. Mühlenweg, H. Jansen, J. Dorn, U. Nitz, E.J. Kantelhardt, C. Thomssen, Cancer therapy trials employing level-of-evidence-1 disease forecast cancer biomarkers uPA and its inhibitor PAI-1. Expert. Rev. Mol. Diagn. 11, 617–634 (2011)
C. Holst-Hansen, B. Johannessen, G. Høyer-Hansen, J. Rømer, V. Ellis, N. Brünner, Urokinase-type plasminogen activation in three human breast cancer cell lines correlates with their in vitro invasiveness. Clin. Exp. Metastasis 14, 297–307 (1996)
K.H. Lee, S.W. Kim, J.R. Kim, Reactive oxygen species regulate urokinase plasminogen activator expression and cell invasion via mitogen-activated protein kinase pathways after treatment with hepatocyte growth factor in stomach cancer cells. J. Exp. Clin. Cancer Res. 28, 73–81 (2009)
R.I. Freshney, Culture of animal cells: a manual of basic technique (Alan R. Liss, Inc., New York, 1987), p. 117
T. Watanabe, T. Miura, Y. Degawa, Y. Fujita, M. Inoue, M. Kawaguchi, C. Furihata, Comparison of lung cancer cell lines representing four histopathological subtypes with gene expression profiling using quantitative real-time PCR. Cancer Cell Int. 21, 2 (2010)
K.J. Gregory, B. Zhao, D.R. Bielenberg, S. Dridi, J. Wu, W. Jiang, B. Huang, S. Pirie-Shepherd, M. Fannon, Vitamin D binding protein-macrophage activating factor directly inhibits proliferation, migration, and uPAR expression of prostate cancer cells. PLoS One 5, e13428 (2010)
E.S. Meade, Y.Y. Ma, S. Guller, Role of hypoxia-Inducible transcription factors 1α and 2α in the regulation of plasminogen activator inhibitor-1 expression in a human trophoblast cell line. Placenta 28, 1012–1019 (2007)
M.M. Bradford, A rapid and sensitive method for the quantitation of microgram quantities of protein utilizing the principle of protein-dye binding. Anal. Biochem. 72, 248–254 (1976)
C. Legrand, M. Polette, J.M. Tournier, S. de Bentzmann, E. Huet, M. Monteau, P. Birembaut, uPA/plasmin system-mediated MMP-9 activation is implicated in bronchial epithelial cell migration. Exp. Cell Res. 264, 326–336 (2001)
C. Gupta, K. Tikoo, High glucose and insulin differentially modulates proliferation in MCF-7 and MDA-MB-231 cells. J. Mol. Endocrinol. 51, 119–1129 (2013)
M. Uchino, H. Kojima, K. Wada, M. Imada, F. Onoda, H. Satofuka, T. Utsugi, Y. Murakami, Nuclear β-catenin and CD44 upregulation characterize invasive cell populations in non-aggressive MCF-7 breast cancer cells. BMC Cancer 10, 414 (2010)
K. Masur, C. Vetter, A. Hinz, N. Tomas, H. Henrich, B. Niggemann, K.S. Zänker, Diabetogenic glucose and insulin concentrations modulate transcriptome and protein levels involved in tumor cell migration, adhesion and proliferation. Br. J. Cancer 104, 345–352 (2011)
X. Kang, F. Kong, X. Wu, Y. Ren, S. Wu, K. Wu, Z. Jiang, W. Zhang, High glucose promotes tumor invasion and increases metastasis-associated protein expression in human lung epithelial cells by upregulating heme oxygenase-1 via reactive oxygen species or the TGF-β1/PI3K/Akt signaling pathway. Cell. Physiol. Biochem. 35, 1008–1022 (2015)
C. Gupta, J. Kaur, K. Tikoo, Regulation of MDA-MB-231 cell proliferation by GSK-3β involves epigenetic modifications under high glucose conditions. Exp. Cell Res. 324, 75–83 (2014)
A.J. Stewart, M.D. Johnson, F.E. May, B.R. Westley, Role of insulin-like growth factors and the type I insulin-like growth factor receptor in the estrogen-stimulated proliferation of human breast cancer cells. J. Biol. Chem. 265, 21172–21178 (1990)
M. Pollak, Insulin and insulin-like growth factor signaling in neoplasia. Nat. Rev. Cancer 8, 915–28 (2008)
N.M. Tomas, K. Masur, J.C. Piecha, B. Niggemann, K.S. Zänker, Akt and phospholipase Cγ are involved in the regulation of growth and migration of MDA-MB-468 breast cancer and SW480 colon cancer cells when cultured with diabetogenic levels of glucose and insulin. BMC Res. Notes 5, 214 (2012)
E. Giovannucci, D.M. Harlan, M.C. Archer, R.M. Bergenstal, S.M. Gapstur, L.A. Habel, M. Pollak, J.G. Regensteiner, D. Yee, Diabetes and cancer: a consensus report. Diabetes Care 33, 1674–1685 (2010)
H.C. Kwaan, A.P. Mazar, B.J. McMahon, The apparent uPA/PAI-1 paradox in cancer: more than meets the eye. Semin. Thromb. Hemost. 39, 382–391 (2013)
A. Ahmad, D. Kong, Z. Wang, S.H. Sarkar, S. Banerjee, F.H. Sarkar, Down-regulation of uPA and uPAR by 3,3′-diindolylmethane contributes to the inhibition of cell growth and migration of breast cancer cells. J. Cell. Biochem. 108, 916–925 (2009)
E. Giacoia, M. Miyake, A. Lawton, S. Goodison, C.J. Rosser, PAI-1 leads to G1-phase cell-cycle progression through cyclin D3/cdk4/6 upregulation. Mol. Cancer Res. 12, 322–334 (2014)
R. Malaguarnera, A. Belfiore. The emerging role of insulin and insulin-like growth factor signaling in cancer stem cells. Front Endocrinol (Lausanne). 5 article 10 (2014)
G. Pandini, F. Frasca, R. Mineo, L. Sciacca, R. Vigneri, A. Belfiore, Insulin/insulinlike growth factor I hybrid receptors have different biological characteristics depending on the insulin receptor isoform involved. J. Biol. Chem. 277, 39684–39695 (2002)
B. Wollenberg, N. Jan, R. Jund, S. Chaubal, M. Untch, Urokinase-type plasminogen activator and its inhibitor plasminogen activator inhibitor-1. Oncol. Rep. 4, 853–855 (1997)
S.E. Dunn, J.V. Torres, J.S. Oh, D.M. Cykert, J.C. Barrett, Up-regulation of urokinase-type plasminogen activator by insulin-like growth factor-I depends upon phosphatidylinositol-3 kinase and mitogen-activated protein kinase kinase. Cancer Res. 61, 1367–1374 (2001)
N. Harbeck, R.E. Kates, K. Gauger, A. Willems, M. Kiechle, V. Magdolen, M. Schmitt, Urokinase-type plasminogen activator (uPA) and its inhibitor PAI-I: novel tumor-derived factors with a high prognostic and predictive impact in breast cancer. Thromb. Haemost. 91, 450–456 (2004)
M.D. Sternlicht, A.M. Dunning, D.H. Moore, P.D. Pharoah, D.G. Ginzinger, K. Chin, J.W. Gray, F.M. Waldman, B.A. Ponder, Z. Werb, Prognostic value of PAI1 in invasive breast cancer: evidence that tumor-specific factors are more important than genetic variation in regulating PAI1 expression. Cancer Epidemiol. Biomarkers Prev. 15, 2107–2114 (2006)
E.J. Kantelhardt, M. Vetter, M. Schmidt, C. Veyret, D. Augustin, V. Hanf, C. Meisner, D. Paepke, M. Schmitt, F. Sweep et al., Prospective evaluation of prognostic factors uPA/PAI-1 in node-negative breast cancer: phase III NNBC3-Europe trial (AGO, GBG, EORTC-PBG) comparing 6×FEC versus 3×FEC/3×Docetaxel. BMC Cancer 16(11), 140 (2011)
F. Margheri, C. Luciani, M.L. Taddei, E. Giannoni, A. Laurenzana, A. Biagioni, A. Chillà, P. Chiarugi, G. Fibbi, M. Del Rosso, The receptor for urokinase-plasminogen activator (uPAR) controls plasticity of cancer cell movement in mesenchymal and amoeboid migration style. Oncotarget 5, 1538–1553 (2014)
M.J. Duffy, P.M. McGowan, N. Harbeck, C. Thomssen, M. Schmitt, uPA and PAI-1 as biomarkers in breast cancer: validated for clinical use in level-of-evidence-1 studies. Breast Cancer Res. 16, 428 (2014)
P. Smith, J. Martinez, Epidermal growth factor stimulates urokinase-type plasminogen activator expression in human gingival fibroblasts. Possible modulation by genistein and curcumin. J. Periodontal Res. 39, 380–387 (2004)
H. Fang, V.R. Placencio, Y.A. DeClerck, Protumorigenic activity of plasminogen activator inhibitor-1 through an antiapoptotic function. J. Natl. Cancer Inst. 104, 1470–1484 (2012)
T. Masuda, N. Hattori, T. Senoo, S. Akita, N. Ishikawa, K. Fujitaka, Y. Haruta, H. Murai, N. Kohno, SK-216, an inhibitor of plasminogen activator inhibitor-1, limits tumor progression and angiogenesis. Mol. Cancer Ther. 12, 2378–2388 (2013)
M. Cartier-Michaud, C. Malo, G. Charriere-Bertrand, C. Gadea, A. Anguille, A. Supiramaniam, F. Lesne, G. Delaplace, P. Hutzler, D.A. Roux, G. Lawrence, Barlovatz-Meimon, Matrix-bound PAI-1 supports cell blebbing via RhoA/ROCK1 signaling. PLoS One 7, e32204 (2012)
F. Samad, M. Pandey, P.A. Bell, D.J. Loskutoff, Insulin continues to induce plasminogen activator inhibitor 1 gene expression in insulin-resistant mice and adipocytes. Mol. Med. 6, 680–692 (2000)
A.I. Vulin, F.M. Stanley, A Forkhead/winged helix-related transcription factor mediates insulin-increased plasminogen activator inhibitor-1 gene transcription. J. Biol. Chem. 277, 20169–20176 (2002)
A.I. Vulin, F.M. Stanley, Oxidative stress activates the plasminogen activator inhibitor type 1 (PAI-1) promoter through an AP-1 response element and cooperates with insulin for additive effects on PAI-1 transcription. J. Biol. Chem. 279, 25172–25178 (2004)
U.R. Jag, J. Zavadil, F.M. Stanley, Insulin acts through FOXO3a to activate transcription of plasminogen activator inhibitor type 1. Mol. Endocrinol. 23, 1587–602 (2009)
C.Y. Sasaki, H. Lin, A. Passaniti, Regulation of urokinase plasminogen activator (uPA) activity by E-cadherin and hormones in mammary epithelial cells. J. Cell. Physiol. 181, 1–13 (1999)
O. Escribano, A. Gómez-Hernández, S. Díaz-Castroverde, C. Nevado, G. García, Y.F. Otero, L. Perdomo, N. Beneit, M. Benito, Insulin receptor isoform A confers a higher proliferative capability to pancreatic beta cells enabling glucose availability and IGF-I signaling. Mol. Cell. Endocrinol. 409, 82–91 (2015)
R. Rostoker, S. Abelson, K. Bitton-Worms, I. Genkin, S. Ben-Shmuel, M. Dakwar, Z.S. Orr, A. Caspi, M. Tzukerman, D. LeRoith, Highly specific role of the insulin receptor in breast cancer progression. Endocr. Relat. Cancer 22, 145–157 (2015)
T. Watabe, K. Yoshida, M. Kaya, K. Fujikawa, H. Sato, M. Seiki, S. Ishii, K. Fujinaga, The Ets-1 and Ets-2 transcription factors activate the promoters for invasion-associated urokinase and collagenase genes in response to epidermal growth factor. Int. J. Cancer 77, 128–137 (1998)
E.A. Lee, J.Y. Seo, Z. Jiang, M.R. Yu, M.K. Kwon, H. Ha, H.B. Lee, Reactive oxygen species mediate high glucose-induced plasminogen activator inhibitor-1 up-regulation in mesangial cells and in diabetic kidney. Kidney Int. 67, 1762–1771 (2005)
M. Díaz-Flores, L.A. Baiza-Gutman, M.A. Ibáñez-Hernández, D. Pascoe-Lira, A.M. Guzmán-Greenfel, J. Kumate-Rodríguez, Aspectos moleculares del daño tisular inducido por la hiperglucemia crónica. Gac. Med. Mex. 140, 437–447 (2004)
L.A. Flores-López, M. Díaz-Flores, R. García-Macedo, A. Avalos-Rodríguez, M. Vergara-Onofre, M. Cruz, A. Contreras-Ramos, M. Konigsberg, C. Ortega-Camarillo, High glucose induces mitochondrial p53 phosphorylation by p38 MAPK in pancreatic RINm5F cells. Mol. Biol. Rep. 40, 4947–4958 (2013)
F. Tanaka, N. Ogura, Y. Abiko, Stimulation of plasminogen activator/plasmin system in gingival fibroblast cells by oxygen radicals. Arch. Oral Biol. 42, 263–270 (1997)
P.N. Khoi, J.S. Park, N.H. Kim, Y.D. Jung, Nicotine stimulates urokinase-type plasminogen activator receptor expression and cell invasiveness through mitogen-activated protein kinase and reactive oxygen species signaling in ECV304 endothelial cells. Toxicol. Appl. Pharmacol. 259, 248–256 (2012)
Y. Uchida, K. Ohba, T. Yoshioka, K. Irie, T. Muraki, Y. Maru, Cellular carbonyl stress enhances the expression of plasminogen activator inhibitor-1 in rat white adipocytes via reactive oxygen species-dependent pathway. J. Biol. Chem. 279, 4075–4083 (2004)
M.C. Alessi, F. Anfosso, M. Henry, F. Peiretti, G. Nalbone, I. Juhan-Vague, Up-regulation of PAI-1 synthesis by insulin and proinsulin in HEP G2 cells but not in endothelial cells. Fibrinolysis 9, 237–242 (1995)
A. Amini, S. Masoumi-Moghaddam, A. Ehteda, D.L. Morris, Bromelain and N-acetylcysteine inhibit proliferation and survival of gastrointestinal cancer cells in vitro: significance of combination therapy. J. Exp. Clin. Cancer Res. 12, 33–92 (2014)
S. Kawakami, Y. Kageyama, Y. Fujii, K. Kihara, H. Oshima, Inhibitory effect of N-acetylcysteine on invasion and MMP-9 production of T24 human bladder cancer cells. Anticancer Res. 21, 213–219 (2001)
Piwkowska, D. Rogacka, I. Audzeyenka, S. Angielski, M. Jankowski, Combined effect of insulin and high glucose concentration on albumin permeability in cultured rat podocytes. Biochem. Biophys. Res. Commun. 461, 383–389 (2015)
Acknowledgments
This work was supported by the Programa de Apoyo a Proyectos de Investigación e Innovación Tecnológica (PAPIIT), Dirección General de Asuntos del Personal Académico (DGAPA) grant number IN 223014 and the Programa de Apoyo a los Profesores de Carrera para la Formación de Grupos de Investigación (PAPCA-2007-2008), Facultad de Estudios Superiores Iztacala, Universidad Nacional Autónoma de México (UNAM). LA Flores-López and AR Viedma-Rodríguez are supported by a DGAPA, UNAM postdoctoral grant.
Author information
Authors and Affiliations
Corresponding author
Ethics declarations
Conflict of interest
The authors declare that they have no conflict of interest.
Rights and permissions
About this article
Cite this article
Flores-López, L.A., Martínez-Hernández, M.G., Viedma-Rodríguez, R. et al. High glucose and insulin enhance uPA expression, ROS formation and invasiveness in breast cancer-derived cells. Cell Oncol. 39, 365–378 (2016). https://doi.org/10.1007/s13402-016-0282-8
Accepted:
Published:
Issue Date:
DOI: https://doi.org/10.1007/s13402-016-0282-8