Abstract
Lakes and reservoirs (impoundments) are often viewed as a sink for nutrients within the river continuum. To date, most studies on nutrient retention within impoundments are derived from the temperate climate zones of Europe and North America, only consider one nutrient, and are often short-term (1–2 years). Here, we present a long-term (17 year) data set and nutrient (nitrogen, phosphorus and silica) budget for two connected semi-arid lakes (the Lower Lakes) at the terminus of the River Murray, Australia. Most of the filterable reactive phosphorus and nitrate entering the lakes were retained (77 and 92%, respectively). Total phosphorus (TP) was also strongly retained (55% of the annual TP load on average) and the annual TP retention rates could be predicted as a function of the areal hydraulic loading rate (annual lake outflow/lake surface area). On average, there was a slight net retention (7%) of the annual total nitrogen (TN) load but a slight net export (6% of the load) of organic N. TN retention as function of the areal hydraulic loading rate was lower than expected from existing models, possibly because of high nitrogen fixation rates in the Lower Lakes. Silica was retained (39%) at similar rates to those observed in previous studies. There was also a marked increase in the TN:TP and TN:Si ratios within the lake (TN:TP~30 and TN:Si~0.67) compared to those entering (TN:TP~15, TN:Si~0.45), as a consequence of the relatively low net retention of nitrogen.
Similar content being viewed by others
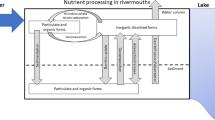
Explore related subjects
Discover the latest articles, news and stories from top researchers in related subjects.Avoid common mistakes on your manuscript.
Introduction
Increased nutrient loading from anthropogenic sources and subsequent eutrophication are one of the greatest threats to aquatic ecosystems. The relationship between algal biomass and nutrient loading is now well established for estuarine and freshwater ecosystems (Howarth and Marino 2006). Anthropogenic activities also tend to modify the relative amounts of nutrients exported from catchments. In general, increased anthropogenic activity leads to a decrease in both the total nitrogen to total phosphorus (TP:TN) ratio (Caraco 1995; Howarth et al. 1995) and the dissolved silica to dissolved inorganic nitrogen (Si:DIN) ratio (Turner 2002). At TN:TP loading ratios <16 (mol:mol) there is an increased tendency for the development of blooms of cyanobacteria capable of nitrogen (N) fixation (Downing et al. 2001; Howarth et al. 1988a; Schindler et al. 2008; Smith and Bennett 1999). Similarly, Si:DIN ratios have been shown to exert an important control on the dominance of diatoms, with Si:DIN ratios <1 tending to favour the growth of flaggelates over diatoms within coastal waters (Turner 2002).
Lakes and artificial impoundments (hereafter referred to as impoundments) are recognised as ‘hot spots’ within riverine systems for the trapping and cycling of nutrients. Virtually all studies to date have clearly shown that N, P and Si are strongly retained by impoundments and a number of models have been developed to describe their retention. Early work throughout the 1970s focused on P retention, reflecting the recognition that P was the limiting nutrient in most freshwater ecosystems (Howarth and Marino 2006). Numerous models of P retention have been developed, starting with the classic work of Vollenweider (Vollenweider 1969) and followed by numerous other studies recently summarised by Brett and Benjamin (Brett and Benjamin 2008). The more recent realisation that N plays a critical role in the eutrophication of coastal waters (Howarth and Marino 2006) has led to increased interest in the retention of N in impoundments (David et al. 2006; Harrison et al. 2009; Saunders and Kalff 2001). Relatively little work has been undertaken on Si retention by comparison to N and P, however, it has been clearly shown that a substantial fraction of Si can also be retained within impoundments (Humborg et al. 2006; Koszelnik and Tomaszek 2008; Triplett et al. 2008). The trapping of N and P by impoundments can generally be viewed as a positive attribute in the context of eutrophication, although we note that nutrient export from rivers, particularly in arid zones is crucial for the maintenance of coastal production (e.g., Gillanders and Kingsford 2002). The trapping of Si is generally viewed as a negative attribute because it is thought to lead to the preferential growth of flagellates over diatoms in coastal waters (Humborg et al. 2000). With the increasing recognition of nutrient stoichiometry in ecological function (Sterner and Elser 2002), it is surprising very few studies have simultaneously considered the retention of N, P and Si within impoundments (Garnier et al. 1999).
Given that the TN:TP loading ratio can exert a strong influence over the abundance of N fixing cyanobacteria (Schindler et al. 2008), it seems reasonable to expect that the TN:TP loading ratio should also exert an influence on the N budget of impoundments. Numerous studies have shown that N fixation may be an important input of N to impoundments, particularly in arid and tropical systems (Ganf and Horne 1975; Horne and Galat 1985; Horne and Goldman 1972; Howarth et al. 1988b). To date, only one study has shown that lakes with high populations of cyanobacteria may actually be a net exporter of N, and this was in a subtropical reservoir with a low N:P (~1:1 mol:mol) loading ratio (Ashton 1981). Despite a relatively large number of nutrient budgets for temperate impoundments (Harrison et al. 2009; Saunders and Kalff 2001; Seitzinger et al. 2002; Tomaszek and Koszelnik 2003), there are no reports of net N export, even in those with blooms of N fixing cyanobacteria (Noges et al. 1998) and N:P loading ratios below 10 mol:mol (Galicka 1992; Galicka and Penczak 1989). It has been observed that areal N fixation rates tend to be higher in arid and subtropical aquatic ecosystems, than in temperate ecosystems, owing to the relatively short growing seasons in temperate regions (Grimm and Petrone 1997; Howarth et al. 1988b). We hypothesise that in arid and subtropical climates N fixation will be an important part of N budgets of riverine impoundments, N fixation will be high at low TN:TP loading ratios (<16) and ultimately lead to reduced N retention in impoundments.
Here we present a long term data set from a shallow semi-arid set of lakes at the terminus of Australia’s largest river system, the River Murray. We use this data to construct budgets for N, P and Si and to assess their relative retention and whether a lake in a semi-arid climate retains N to the same extent as that documented in temperate climates.
Methods
Study site
A detailed description of the Lower Lakes has previously been given in the literature (Geddes 1984; Geddes 1988). The Lower Lakes are situated at the terminus of the Murray-Darling Basin and the River Murray, Australia’s largest drainage basin (1,063,000 km2) and one of the world’s longest rivers (Newman 2000). Due to diversions for irrigation, annual runoff from the river is now <27% of that pre-European settlement, peak runoff is in early summer (when peak demands for irrigation occur) and the frequency and duration of small and medium floods has decreased significantly (Jolly 1996). The Lower Lakes are large (mean area ~8.0 × 108 m2), shallow lakes (maximum depth of 4.1 m) and the temperature ranges between an average minimum of ~10°C in winter up to an average maximum of 22°C in summer (Sims and Muller 2004). Although several local streams discharge into Lake Alexandrina (Fig. 1), their overall contribution to total annual flow are only considered to be significant during periods of low River Murray inputs (Anon 2007). To prevent sea water intrusions in the Lower Lakes, five dams (hereafter referred to as ‘barrages’) were constructed between 1935 and 1940 to maintain the lakes as a permanent freshwater supply for irrigation and human use (Fig. 1).
Lake Alexandrina and Lake Albert have suffered from the regular occurrence of cyanobacterial blooms (including species capable of N fixation), particularly during periods of low flow and low turbidity (P. Baker, Australian Water Quality Centre, “personal communication”). In fact, it was the site of the first record of a toxic bloom of cyanobacteria (Nodularia spumigena) in Australia (Francis 1878). Furthermore, pico-cyanobacterial (e.g. Aphanocapsa, Planktolyngbya) blooms now occur annually, blooms of Nodularia spumigena, Anabaena circinalis Aphanizomenon/Anabaena are common and the first recorded bloom of Cylindrospermopsis raciborskii occurred in the lakes in 2006 (P. Baker, “personal communication”).
Sample collection and analysis
Surface water grab samples from the Lower Lakes (Milang) and the River Murray (Tailem Bend, Fig. 1) were typically collected 1–4 times per month by the Australian Water Quality Centre (AWQC). Water samples from ephemeral tributaries to the Lower Lakes in the Eastern Mount Lofty Ranges were collected sporadically by the Environment Protection Authority (EPA, South Australia) and the South Australian Department of Water, Land and Biodiversity Conservation (DWLBC).
All water samples were analysed by the AWQC, a National Association of Testing Authorities accredited laboratory using standard colorimetric methods. The parameters considered in this study were, nitrate + nitrite (NO x ), silica (Si, molybdenum reactive fraction), total Kjeldahl nitrogen (TKN), filterable reactive phosphorus (FRP) and total phosphorus (TP) because they were the parameters consistently covered at both sites. Total nitrogen (TN) concentrations were calculated as TKN + NO x . Only the years 1979–1996 were considered as this was the most comprehensive period of water quality data collection. Where monthly data were missing (occasionally at Milang in the years 1979–81) the concentration for the missing months was taken as an average of the preceding and subsequent months. This was justified on the basis that there was little variability in the analytes up to and subsequent to the missing data. Analytes below their detection limits (NO x , FRP, and Si) were assumed to have half the concentration of the detection limit for budgeting purposes. Detection limits for nutrients were NO x 0.4 μM, FRP 0.1 μM, TP 0.1 μM, TKN 3.5 μM and Si (silica) 35 μM. Samples for NO x , FRP and Si were filtered through 0.45 μm membrane filters upon collection and stored frozen, and samples for TP and TKN remained unfiltered and were stored frozen before digestion.
Load calculations and lake budget
Annual nutrient retention in the Lower Lakes was calculated as
where M ret is annual retention, M in is the annual load into the lakes from the River Murray and the small streams draining the Eastern Mt Lofty Ranges, M atm is annual atmospheric deposition, M bar is the annual flow over the barrages, and M irr is the annual load withdrawn by irrigation.
Estimates of the monthly inflows via the River Murray into the lakes at Wellington and outflows from the barrages were obtained from the Murray-Darling Basin Authority (MDBA) using the Murray-Darling Basin hydrological model BIGMOD (J. Davis, MDBA, “personal communication”). Nutrient loads into the lakes from the River Murray were calculated by multiplying the average monthly analyte concentration at Tailem Bend by the monthly inflow volume. Monthly loads were summed to obtain annual loads. A comparison of the loads obtained in this manner with more sophisticated approaches such as Kendall’s flow regime stratified ratio method (Kendall et al. 1983), gave identical results. Nutrient loads out of the Lower Lakes were calculated by multiplying the monthly outflow volume by the average monthly concentration of the analyte measured at Milang. Monthly loads were summed to obtain annual loads.
Nutrient concentration data were only sporadically available for the Finniss River, Bremer River and Currency Creek, which together account for 70% of the gauged flow from local streams (DWLBC, “unpublished data”). Consequently, loads were calculated from daily flow measurements and relationships obtained from flow-load regressions where adequate nutrient data existed. The final budget showed contribution of TN, TP and Si from these sources was the insignificant (~1–2%) compared to total inputs to the Lower Lakes. As such, we made no attempt to scale up these estimates for all local stream inputs. This is consistent with the small amount of runoff produced by the local catchment when compared with River Murray inflows.
Irrigation withdrawal loads from the lakes were calculated by multiplying the mean annual concentration of the analyte at Milang by the estimated annual volume of water withdrawn for irrigation. Only annual data were available for irrigation withdrawals from the Lower Lakes spanning 1994–2004. Irrigation withdrawals were therefore estimated by taking the average of the annual withdrawals for the years 1994–2004, which was 31,000 ML year−1 (1 ML = 1000 m3) with a standard deviation of 9000 ML year−1 (DWLBC, “unpublished”).
Rates of atmospheric deposition were calculated from concentrations of NO x , TKN, and FRP in rainwater measured at Adelaide airport (Wilkinson et al. 2006), multiplied by the surface area of the lake and annual average rainfall at Meningie (i.e. constant from year to year). This was justified on the basis of a strong dependence of nutrient deposition on times between rainfall events (i.e., more nutrients were deposited in rainfall events after a long dry period (Wilkinson et al. 2006)). Rates of dry deposition were not included for budgeting purposes for NO x and FRP since these were unknown. Dry deposition rates for TKN were estimated to be 50% of wet deposition rates (Wilkinson et al. 2006). There is no information on TP deposition rates in the region, so we estimated a total annual atmospheric deposition rate of 1 kg ha−1 year−1, which is in the middle of the range of atmospheric deposition rates previously reported (Newman 1995). Inputs to the Lower Lakes from groundwater were ignored for the purposes of this budget since little relevant data exists for these potential inputs. The implications of not including this potential source are discussed later.
Results
Analyte concentrations in the River Murray and the Lower Lakes
Inorganic nutrient (NO x , FRP and Si) concentrations were generally highest in the River Murray and decreased within the Lower Lakes, particularly NO x and FRP (Fig. 2). Si concentrations showed a similar pattern, although this was less apparent. A drought-breaking inflow in late 1983 through to 1984 resulted in consistently high concentrations of all inorganic nutrients in the River Murray, which was also reflected in the Lower Lakes. Indeed, concentrations of Si and FRP remained elevated in the Lower Lakes and the River Murray for several years. By contrast, NO x was rapidly removed from the water column, becoming undetectable within ~1 year. The years 1989–1993 had consistently high flows, but elevated inorganic nutrient concentrations within the lake were only observed in 1990. NH4 + was not determined on a routine basis, however, the limited data set available suggests it was always below the detection limit in the Lower Lakes and comprised a variable fraction (generally insignificant, but up to 50%) of inorganic N in the River Murray.
Total phosphorus concentrations were consistently highest in the River Murray and the highest concentrations in both the river and the Lower Lakes were associated with the drought-breaking inflow event of 1983, as for the inorganic nutrients (Fig. 3). In contrast to TP and the inorganic nutrients, TKN concentrations (essentially organic N because of the low NH4 + concentrations) were consistently highest in the Lower Lakes. The TKN:TP ratio was generally in excess of 20, increasing to in excess of 100 in 1983 and 1991 coincident with large blooms of Nodularia spumigena within the Lower Lakes (Geddes (1988); AWQC, “unpublished data”). There were also less pronounced peaks in the TKN:TP ratio coincident with smaller Anabaena circinalis and Nodularia blooms in 1990, 1991 and 1993–1994 (based on cell counts, data not shown). In general, there was a clear increase in the TN:TP ratio from ~15 in the River Murray, to ~30 within the Lower Lakes (Fig. 4).
Average monthly concentrations of TP (total phosphorus, top panel), TKN (total Kjeldahl nitrogen, upper middle panel), TKN:TP ratio (lower middle panel) and turbidity (bottom panel) at Tailem Bend on the River Murray and Milang in the Lower Lakes. River discharge is also shown on the top panel, units are in megalitres (1 ML = 1000 m3) per month. Marked areas indicate the occurrence of cyanobacterial blooms recorded within the Lower Lakes from 1990 onwards (Peter Baker, AWQC “personal communication”). The 1981 bloom was observed by Geddes (1988)
Plots of NO x versus FRP (filterable reactive phosphorus) and TN (total nitrogen) versus TP (total phosphorus) for Tailem Bend on the River Murray and Milang on the Lower Lakes. Solid lines denote N:P ratios of 20 and 50 which denote the ratios below and above which N and P limitation are expected to occur respectively (Guildford and Hecky 2000)
Turbidity in both the River Murray and the Lower Lakes was highly variable, but generally higher in the Lower Lakes than the River Murray. As was observed for the nutrients, the highest turbidity in the Lower Lakes was associated with the drought breaking 1983 inflow event. High turbidity in the Lower Lakes generally coincided with high turbidity in the inflowing water from the River Murray, with the notable exceptions of the austral summers of 1982/1983, 1986/1987 and 1992/1993, when high turbidity may have been caused by wind driven sediment re-suspension. The periods of lowest turbidity were always associated with periods of low flow, except for the occasional high value, as observed during the 1991 Nodularia bloom.
Nutrient retention in the Lower Lakes
Inorganic nutrients were generally strongly retained in the Lower Lakes with a net retention of NO x , FRP and Si occurring virtually every year (Table 1). Highest absolute retention generally occurred in the highest flow years, whilst lowest absolute retention rates occurred in the lowest flow years. Retention of NO x (normalised to the mean lake surface area) ranged between 19 and 200 mmol m−2 year−1 and generally, 90–100% of the NO x entering the Lower Lakes was retained. For FRP, retention rates varied between 2.1 and 33 mmol m−2 year−1, with between 50 and 100% of FRP entering the Lower Lakes retained. Silica retention in the Lower Lakes was more variable, ranging from a slight source through to being a sink of 2300 mmol m−2 year−1. This represented an export equivalent to 39% of the incoming load and a retention of up to 92% of the incoming load. Retention of TKN was more variable, ranging from a net export of up to 340 mmol m−2 year−1 (25% of incoming load) through to a net retention of 190 mmol m−2 year−1 (35% of incoming load). There was a slight net export of TKN over the study period equivalent to 6% of the total incoming load. TN retention showed a very similar pattern to TKN, but there was a net balance in retention and export over the study period owing to the high retention efficiency of NO x . TP was always retained, ranging between 6 mmol m−2 year−1 in the lowest flow year of 1982, up to 66 mmol m−2 year−1 in the high flow year of 1990. Retention of TP ranged between 27 and 98% of the incoming load, with an overall retention of 55% of the incoming TP over the 17 year study period. In general atmospheric deposition was <20% of the Lower Lakes nutrient load, with the exceptions of the drought years 1982 and 1994, when atmospheric deposition was equivalent to 50 and 108% of the incoming TN and TP loads from the River Murray (data not shown).
Discussion
Whilst numerous nutrient mass balance studies of lakes have been carried out to date, this study is unique in a number of key respects. Firstly, this study reviewed nutrient retention within a semi-arid impoundment, which are hydrologically and ecologically significantly different from the more frequently studied temperate systems. Secondly, the patterns in N, P and Si retention were considered simultaneously. Finally, owing to a relatively long monitoring period, the patterns in nutrient retention between flood and drought periods in the River Murray could be compared. This is an important consideration because semi-arid rivers tend to have larger extremes in their water regime when compared to temperate river systems (Croke and Jakeman 2001). A significant fraction of the P and Si inputs were retained within the Lower Lakes. However, virtually no net N retention was observed over the 17 year study period and the lakes could be a small source of organic N. The potential hydrological and biogeochemical mechanisms responsible for this pattern in nutrient retention are now discussed.
Phosphorus
Phosphorus retention within lakes is most commonly described either as a function of the areal hydraulic loading rate (lake outflow/lake surface area) or the lake hydraulic residence time (lake volume/lake outflow). A recent comprehensive review of TP retention models showed that models formulated on hydraulic residence time are able to most accurately predict TP concentrations within lakes (Brett and Benjamin 2008). However, because hydraulic residence time models are weaker at predicting TP load retention (Kirchner and Dillon 1975), an areal hydraulic loading model was used here to describe N, P and Si retention in the Lower Lakes in common with other studies of waterbodies (e.g., Kadlec and Wallace 2009; Seitzinger et al. 2002).
There was a moderate relationship between the proportion of TP retained and the areal hydraulic load (q s) for the Lower Lakes, with a logarithmic function describing 54% of the variance (Fig. 5). For comparison, we have also shown a previously derived model for TP retention as a function of q s (Dillon and Kirchner 1975):
where R p is the proportion of P retained within the lake and v is the removal rate constant (often referred to as settling velocity). Using the average settling velocity of 13.2 m year−1 expected for North American and European lakes (Dillon and Kirchner 1975), this model tends to slightly over predict TP retention at low-intermediate q s (<7 m year−1), with better conformity at intermediate to high q s (>7 m year−1, Fig. 5). The lower than expected TP retention rates at q s < 7 are probably caused by the shallowness of the lakes, which makes them prone to sediment re-suspension (Geddes 1984).
The proportions of total nitrogen (TN, top panel), total phosphorus (TP, middle panel) and Silica (Si, bottom panel) retained on an annual basis as a function of the areal water loading rate defined as the total annual outflow volume of the lake divided by the surface area. The dashed lines show a previously derived empirical model for TN retention (Seitzinger et al. 2002) and the equation %TP retained = v/(v + q s), where v = 13.2 m year−1 for TP retention (Dillon & Kirchner 1975). The solid lines show the best fit to the data using a logarithmic function
The amount of TP retained within the lake between 1979 and 1996 amounted to an average of 27 mmol m−2 year−1. Based on an average sediment TP content of 250 mg kg−1 dry weight and a sediment porosity of 0.65 (Aldridge et al. 2009), this amounts to an average sedimentation rate of ~4 mm year−1 over the lake. This is in good agreement with a sedimentation rate of 3 mm year−1 calculated by Herczeg et al. (2001) in the Lower Lakes using 210Pb. As well as sedimentation, another possible sink for P is removal in the form of fish biomass by the commercial fisheries on the Lower Lakes. Using total fish catch data from the years 1985–1996 (South Australian Research and Development Institute, “unpublished data”) and assuming that P comprises 25 g kg−1 dry fish biomass, we estimate that fish biomass removal accounts for 2% of the total retention over the entire period 1985–1996. However, considerable variation was apparent, with calculated values ranging between 1 and 60%. The high proportion of 60% arose in the drought year 1994, when the mass of TP retained in the lake was negligible due to low TP inputs. The average estimated rate of P removal in fish biomass is somewhat lower than a rate of 10% estimated for Lake Donghu in China (Tang and Ping 2000).
Nitrogen
The retention of TN within the Lower Lakes was generally much lower than that observed in other studies (Seitzinger et al. 2002), particularly at areal hydraulic loading rates of 5–20 m year−1. In fact, there was often an observed net export of N from the Lower Lakes (Fig. 5). This is quite unusual, with the only other reported instance of net annual N export that we could find occurring in the Rietvlei dam in South Africa (Ashton 1981). In that instance, high rates of N fixation were directly measured in the dam and were of a similar magnitude to the net export of N. While there are no published rates of N fixation from the Lower Lakes, it seems reasonable to assume N fixation rates will be substantial, given the presence of several species of N fixing cyanobacteria. Further evidence for the occurrence of N fixation comes from inspection of water column data collected at Milang. There was often a continued drawdown of FRP after NO x became undetectable in the water column (Fig. 2), suggesting severe N limitation, which one would normally expect to lead to a decrease in the TN:TP ratio to below 20 (Guildford and Hecky 2000). Despite this, the TKN:TP ratio (in this case TKN≈TN, because NO x was always low or undetectable) virtually always remained above 20, and was often in excess of 100 when NO x was undetectable (Fig. 3). The largest peaks in TKN:TP in 1980 and 1991 also coincided with documented blooms of Nodularia spumigena (Fig. 3). The net effect of this additional source of N was to substantially increase the TN:TP ratio in the Lower Lakes compared to that entering from the River Murray (Fig. 4). This observation is consistent with fertilisation experiments on Lake 227, where despite TN:TP loading ratios being reduced to <10 (mol:mol), the TN:TP ratio within the lake remained >40 as a consequence of N fixation by cyanobacteria (Schindler et al. 2008).
Based on the previously estimated sedimentation rate and a sediment TN content of 0.25% dry mass (Herczeg et al. 2001), we calculate a TN loss to the sediment of 620 mmol m−2 year−1 for the Lower Lakes over the study period. Denitrification is also likely to be a sink for N within the Lower Lakes, however, we have no long term or widespread measurements of denitrification rates in this system. A synthesis of denitrification as a function of TN loading rate in a range of aquatic systems found that TN loading was a strong predictor of the denitrification rate (r 2 = 0.77) and that ~25% of the TN load was denitrified (Seitzinger et al. 2006). Based on this relationship and the measured annual average TN loading rate of 720 mmol m−2 year−1 (Table 1), we calculate an average denitrification rate of ~180 mmol m−2 year−1. There is, however, considerable uncertainty around this estimate of denitrification for several reasons. Firstly, the regression that this estimate is based on, is derived predominantly from North American and European systems, in which, NO x generally comprises >40% of TN loads (David et al. 2006; Galicka and Penczak 1989; Howarth et al. 1996; Noges et al. 1998) compared to 13% of TN loads to the Lower Lakes. Given that NO x concentrations exert a strong control over denitrification (Seitzinger 1988), one would expect much higher removal rates of N in impoundments where NO x is the dominant fraction of TN. In this regard, our estimate may be an over estimate. Secondly, as discussed below, there is likely to be a substantial amount of extra N loading to the Lower Lakes through N fixation, which is not taken into account in the above estimate of denitrification. In this regard our estimate of denitrification may be an underestimate.
A mass balance of TN losses (export, burial and denitrification) with known TN sources (river import and atmospheric deposition) is complicated by the fact that we have a highly uncertain estimate of denitrification. Assuming no denitrification takes place, leaves a minimum deficit of 570 mmol m−2 year−1. Including our above estimate of denitrification leaves a deficit of ~750 mmol m−2 year−1. We, therefore, conservatively estimate a minimum TN deficit in the range 570–750 mmol m−2 year−1 which we ascribe to N fixation as previously discussed (Fig. 6). A comparison of this deficit with previously reported N fixation rates shows it is well above the rate of ~120–140 mmol m−2 year−1 previously reported for desert lakes (Horne and Galat 1985; Horne and Goldman 1972). It also overlaps the high end of the range of 360–650 mmol m−2 year−1 previously reported for eutrophic tropical and subtropical lakes (Ashton 1981; Ganf and Horne 1975; Horne and Viner 1971). Viewed another way, the deficit of 570–750 mmol m−2 year−1 is roughly equivalent to the TN load into the Lower Lakes from the River Murray which is consistent with previous estimates of N fixation contributing on the order of 50% to total inputs to lakes in arid and tropical systems (Ashton 1981; Horne and Galat 1985; Horne and Goldman 1972; Horne and Viner 1971). Thus, while the rates of N fixation required to meet our budget deficit are high, they are plausible based on previous studies.
An approximate budget for N and P in the Lower Lakes spanning the years 1979–1996. Quantities shown are the average annual rate normalised to the surface area of the lake over the 17 year study period with units in mmol m−2 year−1. TN denotes total nitrogen, TP denotes total phosphorus, FRP denotes filterable reactive phosphorus, TKN denotes total Kjeldahl nitrogen, Den denotes denitrification and Nfix denotes nitrogen fixation. Please refer to discussion section ‘nitrogen’ for details of how nitrogen fixation and denitrification were estimated
The finding that the Lower Lakes consumed NO x and were a slight source of TKN contrasts with a review of the biogeochemistry of N and P in Australian catchments (Harris 2001). One of the main conclusions of that study was that Australian lakes and reservoirs consume TKN and DON and release NO x . The mechanism behind our observations was most likely the assimilation of NO x by phytoplankton to form biomass (TKN) as well as loss through denitrification. We ascribe this discrepancy to the fact that the lakes reviewed by Harris (2001) were deeper and that the water released was generally bottom water. As such, these waters would have been much more aphotic than those in the current study, leading to the dominance of organic matter mineralisation rather than production through photosynthesis.
Silicon
In contrast to N and P, the proportion of Si retained within the Lower Lakes showed no clear relationship with the areal water loading (Fig. 5). There was a substantial retention of Si within the Lower Lakes over the study period, amounting to a total of ~40% of the incoming load over the study period, which is consistent with a range of 40–60% estimated in previous studies (Garnier et al. 1999; Humborg et al. 2006).
Stoichiometry of nutrient retention within the Lower Lakes
There is much interest in the interaction between nutrient ratios and ecological processes (Sterner and Elser 2002) and findings of this study provide a clear example of this on a landscape scale. The extremely low TN:TP loading ratio (TN:TP = 15 mol:mol) to the Lower Lakes, in combination with warm temperatures, were probably the major factors favouring the dominance of N fixing cyanobacteria (Downing et al. 2001; Howarth et al. 1988a; Schindler et al. 2008; Smith and Bennett 1999). Ensuing high rates of N fixation resulted in a large increase in the TN:TP ratio of material leaving the lower lakes (TN:TP = 30, Fig. 6). These findings are consistent with the conclusion of Schindler et al. (2008) that any deficit in N availability relative to P will be made up through N fixation and that P loading was the ultimate control on algal biomass in this system. As such, we ascribe the low retention of N relative to P within the lower Lakes to the low TN:TP loading to the system. As a consequence, future models of N retention within impoundments may need to include the TN:TP loading ratio, particularly in tropical and arid regions to make them more globally applicable.
Si was also retained within the Lower Lakes much more efficiently than TN, resulting in the ratio of TN:Si ratio entering from the River Murray being increased from 0.45 to 0.67 over the entire study period (Fig. 6). It has been suggested that the trapping of Si by impoundments leads to a relative depletion in Si because anthropogenic activities replenish N and P, but not Si. The end result of this is an increase in flagellate blooms at the expense of diatoms (which have a high Si requirement) in coastal waters (Humborg et al. 2000). Whilst the Lower Lakes did reduce the amount of Si being released compared to TN, the TN:Si ratio exported from the Lower Lakes was still less than the ratio of ~1 required for balanced diatom growth (Turner 2002). As such, even if all TN were to become bioavailable upon leaving the Lower Lakes (which is highly unlikely), Si would be unlikely to limit diatom growth within the river plume.
Budget approach and validity
One shortcoming of this study is that the concentrations used to calculate the loads out of the Lower Lakes were measured at Milang which is ~20 km from the barrages. To assess the potential bias introduced by this we compared data from Milang with simultaneously collected data from Goolwa, located near one of the barrages (monthly, n = 85, years 1999–2006). Unfortunately, nutrients sampled at sites other than Milang were limited to NO x and TKN, so our comparison is restricted to N. NO x remained below the detection limit virtually at all times at Goolwa, confirming the uniform and rapid consumption of NO x throughout the lakes. TKN concentrations were more variable with concentrations being slightly higher at Milang (83 μM) than Goolwa (77 μM). However, these concentrations were not significantly different (t-test; p > 0.05). Furthermore, as previously discussed there was excellent agreement between our estimates of TP retention over the study period and an independent estimate based on sediment P content and previously measured sedimentation rates. We are therefore confident that our budget approach yielded an accurate estimate of nutrient retention within the lakes.
Given the lack of spatial concentration data within the Lower Lakes, we also neglected changes in nutrient storage within the lakes. This may be a problem when interpreting data for a single year budget because a change in storage may be a significant proportion of the budget. Over the longer term, changes in storage are less of a problem, because years of net release will be tempered against years of net storage. We note that the water level in the Lower Lakes was kept at a relatively constant height and as such changes in volume from year to year were insignificant, except in the drought years 1982 and 1995, when the volume dropped by 15%. Thus, changes in storage will arise primarily as a consequence of changes in internal concentrations rather than changes in lake volume.
One possible source of N and P to the Lower Lakes that we have not considered in detail is groundwater since there is limited information available for the region. The discharge from the Angus Bremer Aquifer to the west is on the order of 3000 ML per year (Telfer et al. 2004). This is equivalent to 0.3% of the discharge of the River Murray in the lowest flow year, 1983, when the relative contribution from groundwater is likely to be highest. This aquifer is likely to be the source of the vast majority of groundwater discharge to the Lower Lakes, because it is recharged from the Mt Lofty ranges which have the highest annual rainfall in the region (~600–800 mm per year compared to <400 mm on the surrounding plains; Australian Bureau of Meteorology). We assume this aquifer accounts for half the groundwater discharge into the Lower Lakes. There is no TN and TP concentration data available for the aquifers surrounding the Lower Lakes, however, the agricultural intensity is low and there is no reason to believe the concentrations of these nutrients are elevated. Using data from contaminated aquifers under regions of more intensive agriculture 250 km to the South East (South Australian EPA, “unpublished data”), we calculate a TN and TP delivery equivalent to ~6% and ~1%, respectively, of the TN and TP entering the Lower Lakes from all other sources in the lowest flow year 1983. At this time the relative contribution from groundwater would be at it’s highest. Therefore, groundwater is unlikely to make a significant contribution to the TN and TP budget of the Lower Lakes.
Summary and conclusions
The Lower Lakes were a substantial sink for NO x , FRP, TP and Si, however there was relatively little retention of TN (Fig. 6). Consequently, there was a marked change in the TN:TP and TN:Si ratios of the loads exported from the Lower Lakes (TN:TP = 30, TN:Si = 0.67) compared to those entering (TN:TP = 15, TN:Si = 0.45). TP retention generally conformed to existing models derived from temperate lakes in Europe and North America. N, by contrast, showed much lower retention rates than expected from previously derived models in Europe and North America. We suggest the low N retention within the Lower Lakes was caused by high rates of N fixation by cyanobacteria, in agreement with previous studies of arid and subtropical lakes. Models for N retention in lakes outside the temperate regions for which most current models are derived, should therefore be based on empirical data derived from the region of interest. The models should also consider the N:P loading ratios which may exert a strong influence over N retention within lakes when the TN:TP loading ratio drops below ~16 (mol:mol).
References
Aldridge KT, Deegan BM, Lamontagne S, Bissett A, Brookes JD (2009) Spatial and temporal changes in water quality and sediment character in Lake Alexandrina and Lake Albert during a period of rapid water level drawdown. CSIRO: Water for a Healthy Country National Research Flagship, Canberra
Anon (2007) The Lower Lakes, Coorong and Murray Mouth icon site environmental management plan 2006–2007. Murray-Darling Basin Commission, Canberra, Australian Capital Territory
Ashton PJ (1981) Nitrogen-fixation and the nitrogen budget of a eutrophic impoundment. Water Res 15:823–833
Brett MT, Benjamin MM (2008) A review and reassessment of lake phosphorus retention and the nutrient loading concept. Freshw Biol 53:194–211
Caraco N (1995) Influence of human populations on P transfers to aquatic systems: a regional scale study using large rivers. In: Tiessen H (ed) Phosphorus in the global environment: transfers cycles and management. John Wiley & Sons, Chichester, pp 235–244
Croke BFW, Jakeman AJ (2001) Predictions in catchment hydrology: an Australian perspective. Mar Freshw Res 52:65–79
David MB, Wall LG, Royer TV, Tank JL (2006) Denitrification and the nitrogen budget of a reservoir in an agricultural landscape. Ecol Appl 16:2177–2190
Dillon PJ, Kirchner WB (1975) Reply. Water Resour Res 11:1035–1036
Downing JA, Watson SB, McCauley E (2001) Predicting Cyanobacteria dominance in lakes. Can J Fish Aquat Sci 58:1905–1908
Francis G (1878) Poisonous Australian lake. Nature 2:11–12
Galicka W (1992) Total nitrogen and phosphorus budgets in the lowland Sulejow Reservoir for the hydrological years 1985–1988. Archiv Fur Hydrobiologie 159–169
Galicka W, Penczak T (1989) Total nitrogen and phosphorus budgets in the lowland Sulejow Reservoir. Arch Hydrobiol 117:177–190
Ganf GG, Horne AJ (1975) Diurnal stratification, photosynthesis and nitrogen fixation in a shallow, equatorial lake (Lake George, Uganda). Freshw Biol 5:13–39
Garnier J, Leporcq B, Sanchez N, Philippon X (1999) Biogeochemical mass-balances (C, N, P, Si) in three large reservoirs of the Seine Basin (France). Biogeochemistry 47:119–146
Geddes MC (1984) Limnology of Lake Alexandrina, River Murray, South Australia, and the effects of nutrients and light on the phytoplankton. Aust J Mar Freshw Res 35:399–415
Geddes MC (1988) The role of turbidity in the limnology of Lake Alexandrina, River Murray, South-Australia––comparisons between clear and turbid phases. Aust J Mar Freshw Res 39:201–209
Gillanders BM and Kingsford MJ (2002) Impact of changes in flow of freshwater on estuarine and open coastal habitats and the associated organisms. Oceanograph Marine Biol 40:233–309
Grimm NB, Petrone KC (1997) Nitrogen fixation in a desert stream ecosystem. Biogeochemistry 37:33–61
Guildford SJ, Hecky RE (2000) Total nitrogen, total phosphorus, and nutrient limitation in lakes and oceans: is there a common relationship? Limnol Oceanogr 45:1213–1223
Harris GP (2001) Biogeochemistry of nitrogen and phosphorus in Australian catchments, rivers and estuaries: effects of land use and flow regulation and comparisons with global patterns. Mar Freshw Res 52:139–149
Harrison JA, Maranger RJ, Alexander RB, Giblin AE, Jacinthe PA, Mayorga E, Seitzinger SP, Sobota DJ, Wollheim WM (2009) The regional and global significance of nitrogen removal in lakes and reservoirs. Biogeochemistry 93:143–157
Herczeg AL, Smith AK, Dighton JC (2001) A 120 year record of changes in nitrogen and carbon cycling in Lake Alexandrina, South Australia: C:N, delta N-15 and delta C-13 in sediments. Appl Geochem 16:73–84
Horne AJ, Galat DL (1985) Nitrogen-fixation in an oligotrophic, saline desert lake––Pyramid Lake, Nevada. Limnol Oceanogr 30:1229–1239
Horne AJ, Goldman CR (1972) Nitrogen-fixation in Clear-Lake, California. 1. Seasonal-variation and role of heterocysts. Limnol Oceanogr 17:678–692
Horne AJ, Viner AB (1971) Nitrogen fixation and its significance in tropical Lake George, Uganda. Nature 232:417–418
Howarth RW, Marino R (2006) Nitrogen as the limiting nutrient for eutrophication in coastal marine ecosystems: evolving views over three decades. Limnol Oceanogr 51:364–376
Howarth RW, Marino R, Cole JJ (1988a) Nitrogen fixation in freshwater, estuarine, and marine ecosystems. 2. Biogeochemical controls. Limnol Oceanogr 33:688–701
Howarth RW, Marino R, Lane J, Cole JJ (1988b) Nitrogen fixation in freshwater, estuarine and marine ecosystems. I. Rates and importance. Limnol Oceanogr 33:669–687
Howarth RW, Jensen HS, Marino R, Postma H (1995) Transport to and processing of P in near-shore and oceanic waters. In: Tiessen H (ed) Phosphorus in the global environment. John Wiley and Sons, Chichester
Howarth RW, Billen G, Swaney D, Townsend A, Jaworski N, Lajtha K, Downing JA, Elmgren R, Caraco N, Jordan T, Berendse F, Freney J, Kudeyarov V, Murdoch P, Zhu ZL (1996) Regional nitrogen budgets and riverine N&P fluxes for the drainages to the North Atlantic Ocean: natural and human influences. Biogeochemistry 35:75–139
Humborg C, Conley DJ, Rahm L, Wulff F, Cociasu A, Ittekkot V (2000) Silicon retention in river basins: far-reaching effects on biogeochemistry and aquatic food webs in coastal marine environments. Ambio 29:45–50
Humborg C, Pastuszak M, Aigars J, Siegmund H, Morth CM, Ittekkot V (2006) Decreased silica land-sea fluxes through damming in the Baltic Sea catchment––significance of particle trapping and hydrological alterations. Biogeochemistry 77:265–281
Jolly ID (1996) The effects of river management on the hydrology and hydroecology of arid and semi-arid floodplains. In: Anderson MG, Walling DE, Bates PD (eds) Floodplain processes. Wiley, New York, pp 577–609
Kadlec RH, Wallace SD (2009) Treatment wetlands. CRC Press, Boca Raton
Kendall A, Stuart A, Ord JK (1983) Advanced theory of statistics. Griffin, London
Kirchner WB, Dillon PJ (1975) An empirical method of estimating the retention of phosphorus in lakes. Water Resour Res 11:182–183
Koszelnik P, Tomaszek JA (2008) Dissolved silica retention and its impact on eutrophication in a complex of mountain reservoirs. Water Air Soil Pollut 189:189–198
Newman EI (1995) Phosphorus inputs to terrestrial ecosystems. J Ecol 83:713–726
Newman B (2000) Hydrology of the Lower Lakes and Coorong. In: Jensen A, Good M, Tucker P, Tucker P, Long M (eds) River Murray barrages environmental flows. Murray-Darling Basin Commission, Canberra, pp 14–22
Noges P, Jarvet A, Tuvikene L, Noges T (1998) The budgets of nitrogen and phosphorus in shallow eutrophic Lake Vortsjarv (Estonia). Hydrobiologia 363:219–227
Saunders DL, Kalff J (2001) Nitrogen retention in wetlands, lakes and rivers. Hydrobiologia 443:205–212
Schindler DW, Hecky RE, Findlay DL, Stainton MP, Parker BR, Paterson MJ, Beaty KG, Lyng M, Kasian SEM (2008) Eutrophication of lakes cannot be controlled by reducing nitrogen input: results of a 37-year whole-ecosystem experiment. Proc Natl Acad Sci USA 105:11254–11258
Seitzinger SP (1988) Denitrification in freshwater and coastal marine ecosystems: ecological and geochemical significance. Limnol Oceanogr 33:702–724
Seitzinger SP, Styles RV, Boyer EW, Alexander RB, Billen G, Howarth RW, Mayer B, Van Breemen N (2002) Nitrogen retention in rivers: model development and application to watersheds in the northeastern USA. Biogeochemistry 57:199–237
Seitzinger S, Harrison JA, Bohlke JK, Bouwman AF, Lowrance R, Peterson B, Tobias C, Van Drecht G (2006) Denitrification across landscapes and waterscapes: a synthesis. Ecol Appl 16:2064–2090
Sims T, Muller K (2004) A fresh history of the lakes: Wellington to the Murray Mouth 1800s to 1935. River Murray Catchment Water Management Board, Berri, South Australia
Smith VH, Bennett SJ (1999) Nitrogen:phosphorus supply ratios and phytoplankton community structure in lakes. Arch Hydrobiol 146:37–53
Sterner RW, Elser JJ (2002) Ecological stoichiometry: the biology of elements from molecules to the biosphere. Princeton University Press, Princeton
Tang H, Ping X (2000) Budgets and dynamics of nitrogen and phosphorus in a shallow hypereutrophic lake in China. J Freshw Ecol 15:505–514
Telfer A, Hopkins B and Santich M (2004) Angas Bremer prescribed wells area. A review of hydrogeology. Phase 2 River Murray Catchment Board, Adelaide
Tomaszek JA, Koszelnik P (2003) A simple model of nitrogen retention in reservoirs. Hydrobiologia 504:51–58
Triplett LD, Engstrom DR, Conley DJ, Schellhaass SM (2008) Silica fluxes and trapping in two contrasting natural impoundments of the upper Mississippi River. Biogeochemistry 87:217–230
Turner RE (2002) Element ratios and aquatic food webs. Estuaries 25:694–703
Vollenweider RA (1969) Moeglichkeiten un Grenzen elementarer modelle der Stoffbilanz von Seen. Arch Hydrobiol 66:1–36
Wilkinson J, Bestland E, Smyth L and White N (2006) The loads of particulate matter and atmospheric nitrogen deposited from wet and dryfall to Adelaide metropolitan coastal waters. ACWS technical report no. 17 prepared for the Adelaide Coastal Waters Study Steering Committee. Flinders University of South Australia, Adelaide
Acknowledgements
This work was supported by Land and Water Australia. We thank the Murray-Darling Basin Authority, Department of Water, Land and Biodiversity Conservation and Environment Protection Authority (South Australia) for access to their water quality data. We also thank Caroline Fazekas and Phil Thomas from the Australian Water Quality Centre for providing the water quality data and advice on them, as well as Joe Davis from the MDBA for supplying the flow data.
Author information
Authors and Affiliations
Corresponding author
Rights and permissions
About this article
Cite this article
Cook, P.L.M., Aldridge, K.T., Lamontagne, S. et al. Retention of nitrogen, phosphorus and silicon in a large semi-arid riverine lake system. Biogeochemistry 99, 49–63 (2010). https://doi.org/10.1007/s10533-009-9389-6
Received:
Accepted:
Published:
Issue Date:
DOI: https://doi.org/10.1007/s10533-009-9389-6