Abstract
Increased N inputs through chronic atmospheric deposition has enriched temperate forest ecosystems, altering critical ecosystem functions such as decomposition and potentially resulting in a shift to P limitation. We used a combination of microbial biomass stoichiometry and enzymatic activity analyses to evaluate the potential for microbial nutrient limitation over the course of a growing season in response to multi-decadal, whole-watershed N enrichments and a one time, plot-scale P addition that occurred in the 22nd year of whole-watershed treatments. The one-time P addition increased microbial biomass threefold and reduced N-acetyl-glucosaminidase (NAG) and acid phosphatase (AP) activity 1 week after application, but there was no interaction with long-term experimental N enrichment to indicate a shift to P limitation. However, both N and P treatments increased C limitation independently of each other over the duration of the study based on measured increases in β-1,4-glucosidase (BG) activity relative to NAG and AP. Microbial biomass stoichiometry and enzyme activity indicated that BBWM is P limited regardless of N status. Our findings highlight the complex interactions between C, N, and P use and limitation in a forested ecosystem subjected to long-term N enrichment.
Similar content being viewed by others
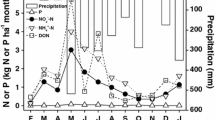
Explore related subjects
Discover the latest articles, news and stories from top researchers in related subjects.Avoid common mistakes on your manuscript.
Introduction
Heterotrophic microbes regulate the availability of nutrients for primary producers through soil biogeochemical processes such as organic matter decomposition, nutrient transformations, and mineral weathering (Banfield and others 1999; Falkowski and others 2008; Sinsabaugh and others 2009). One important driver of microbial organic material mineralization rates is the relative availability of nutrients, particularly nitrogen (N) and phosphorus (P), as they affect the production of organic matter-degrading exoenzymes, potentially determining whether carbon (C) is sequestered or respired (Manzoni and others 2012; Marklein and Houlton 2012). Given the important role of microbes in soil nutrient cycling and C storage, it is critical to understand how they respond to human-driven changes in nutrient loads.
Atmospheric transport and deposition of N is the dominant pathway of anthropogenic N distribution at the global scale (Galloway and others 2008). Long-term elevated N deposition has impacted temperate forest ecosystems by enriching the N content of tree foliage and soil organic matter (Aber and others 1989; Driscoll and others 2001; Lovett and others 2013; Frey and others 2014) and increasing soil C sequestration by suppressing decomposition (Pregitzer and others 2008; Frey and others 2014). Increased N availability due to deposition also reduces tree root exudate production, which can in turn contribute to decreased microbial respiration (Janssens and others 2010). N deposition may further disrupt microbial processes by reducing microbial biomass and shifting functional community composition (Frey and others 2004; Treseder 2008; Leff and others 2015).
Primary production in temperate forest ecosystems is often N limited, but N deposition can shift primary producers to P limitation (Naples and Fisk 2010; Vitousek and others 2010; Crowley and others 2012). Unlike N, which can be biologically fixed from the atmosphere, P availability in soil is ultimately limited by mineral weathering (Schlesinger and Bernhardt 2013). In the absence of N deposition, P limitation in forest soils usually occurs in old, weathered soils such as in the lowland tropics (Porder and others 2007). However, atmospheric N deposition can increase the likelihood of P limitation of primary producers and microbes in North American forest soils by relaxing N limitation (Elser and others 2000; Crowley and others 2012). Furthermore, acidification associated with atmospheric N deposition can further enhance P limitation by increasing P sorption to aluminum (Al) and iron (Fe) oxides (Gallardo and Schlesinger 1994; Norton and others 2006).
The interactive nature of N and P dynamics under atmospheric deposition has the potential to alter C cycling. In trees, N deposition results in reallocation of fixed C from labile root exudates and root biomass to woody biomass (Pregitzer and others 2008). As P limitation increases, trees allocate energy into phosphatase and organic acid for P acquisition rather than the C-rich exudates that encourage microbial symbioses (Hinsinger 2001; Lynch and Ho 2005). N deposition alters microbial community structure and function by suppressing fungal biomass (Frey and others 2004; Treseder 2008) and richness (Eisenlord and others 2013). N deposition also suppresses ligninolytic enzymes and promotes cellulase activity (Carreiro and others 2000; Talbot and Treseder 2012), potentially contributing to a more recalcitrant soil C pool by promoting lignin accumulation (Waldrop and others 2004). As a result, this more recalcitrant C may be stored in soil rather than respired to the atmosphere as CO2 (Nave and others 2009; Lovett and others 2013).
We used a multi-decadal whole-watershed manipulation, the Bear Brook Watershed in Maine (BBWM), to test the idea that chronic, experimentally elevated N and acid deposition may have shifted the stoichiometric balance in soils toward microbial P limitation. Chronic experimental N enrichment and acidification have driven a whole watershed at BBWM to stage two of N saturation (Aber and others 1998), resulting in seasonally high exports of stream nitrate (Fernandez and others 2010; Simon and others 2010), elevated foliar N (Elvir and others 2005; Fernandez and others 2010), increased total N and nitrate in soil solution (Fatemi and others 2012), and higher soil total N (Fernandez and others 2010). At the time of our study, there was no significant difference in extractable phosphate in the organic horizon, but experimental P additions resulted in enhanced immobilization of inorganic N (Salvino 2014). Both Fatemi and others (2016) and Mineau and others (2014) found no evidence of P limitation on enzymatic activities in BBWM soils, but Mineau and others (2014) reported that N enrichments had enhanced P limitation of stream biofilm communities. These studies demonstrated clear differences in whole ecosystem N cycling between watersheds, and mixed evidence for N and P limitations in soil microbial processes.
Therefore, we used microbial biomass stoichiometry and enzymatic activity to evaluate microbial nutrient limitation in response to long-term, whole-watershed N enrichment and a short-term, plot-scale P addition within the treated and reference watersheds. We aimed to either validate the lack of enzymatic response to P reported earlier in soils for this site or find evidence for coupled enzymatic response to nutrient deficiencies. The prevailing hypothesis was that long-term N enrichment-enhanced microbial P limitation in the organic soil horizon, and that short-term P addition would elicit a microbial biomass and enzyme response, and that response would be more pronounced in the N-treated watershed as a result of enhanced P limitation. We also hypothesized that long-term N enrichment and short-term P addition would induce C limitation.
Methods
Site Description
The BBWM is composed of contiguous reference (11.0 ha, East Bear Brook, REF) and treatment (10.3 ha, West Bear Brook, +N) watersheds located on the southeastern slope of Lead Mountain in Maine, USA (44°51′33.9″N, 68°06′19.5″W). The treated watershed had been enriched with ammonium sulfate ((NH4)2SO4) via bimonthly additions by helicopter since November 1989 with an annual loading rate of 25.2 kg N ha−1 y−1 and 28.8 kg S ha−1 y−1. Soils are mostly Typic Haplorthods and Haplohumods with an average depth to bedrock of 1.0 m. The lower elevations are dominated by hardwood stands (HW; American beech, Fagus grandifolia and sugar maple, Acer saccharum), and the higher elevations are dominated by softwood stands (SW; red spruce, Picea rubens, with sparse balsam fir, Abies balsamea).
Experimental Design and Sample Collection
The two watersheds (REF, +N) and vegetation types (HW, SW) formed four experimental compartments. We used five, 15 × 10 m long-term research plots in each compartment. Each plot was subdivided into six 5 × 5 m subplots, and we used 2 subplots arranged 5 m apart laterally along the slope. One subplot was enriched with P (+P), and the other served as a control (No-P). Air and soil temperature were measured at the plot level using HOBO HS Outdoor/Industrial four channel data loggers (Onset Computer Corporation, Bourne, MA) as described in Fernandez and others (2007).
Sodium phosphate monobasic monohydrate (NaH2PO4·H2O) dissolved in stream water from the REF watershed was applied to the +P subplots at a rate equivalent to 100 kg P ha−1 on 1 June 2012. This loading rate was in the range of enrichments used by others in decomposition experiments (67–150 kg P ha−1 y−1; Hobbie and Vitousek 2000; McGroddy and others 2004; Cleveland and others 2006). The solution was sprayed uniformly across the surface of the subplots by multiple passes at right angles with a backpack sprayer. Control subplots were sprayed with an equivalent volume of REF stream water. The sodium (Na) salt form of P was chosen to avoid the addition of essential mineral nutrients such as potassium (K) and calcium (Ca).
Soil samples were collected from both types of subplots at 6, 68, and 136 days after P addition; these will be referred to as June, August, and October, respectively, to be consistent with other works related to this study (Table 1). For each sampling event, three samples of the organic (O) horizon were collected at the start, middle, and end of a 2 m transect within each subplot. Prior to sampling, loose litter not mechanically attached to the upper surface of the O horizon was removed. O horizon materials within a 10 × 10 cm template were excavated down to the mineral soil using clippers and a hand saw to separate the soil block from the surrounding soil. The boundary between the O horizon and mineral soil was typically abrupt and distinct. The soil blocks were placed in Ziploc® bags and held in coolers with icepacks for transport and storage before processing for soil chemistry, microbial biomass stoichiometry, and microbial enzyme activity.
Soil Processing
All analyses were carried out on sieved, homogenized O horizon materials in field moist conditions. Soils were sieved though a 6-mm mesh sieve to separate fine from coarse fragments and roots. The triplicate subsamples taken within each subplot were combined and homogenized to produce one sample per subplot. Roughly 25 g from each bulked sample was transferred by an ethanol-cleaned spatula into 50 ml polypropylene tubes and stored at −65°C until enzymatic analysis. Gravimetric moisture content was determined by weighing subsamples before and after drying at 65°C for 24 h in pre-weighed crucibles. The dried samples were then placed in a muffle furnace at 450°C for 12 h, cooled and weighed to determine loss-on-ignition (LOI).
Soil Chemistry
Extractable phosphate-P (PO4 −-P), total C (TC), and total N (TN) were measured on O horizon materials from three plots in each compartment. For PO4 −-P, 15 g of soil was mixed with 100 ml of 1 N ammonium chloride (NH4Cl) and placed on a mechanical shaker for 1 h. Samples were vacuum filtered through Whatman #42 filter papers into 60 ml, 10% hydrochloric acid (HCl) washed bottles. Three milliliters of HCl was added to acidify the extracts. Extracts were stored at −20°C until analysis on an O.I. Alpkem A/E auto-analyzer (O.I. Analytical, College Station, TX, USA). TC and TN were determined via combustion of O horizon aliquots on a LECO CN-2000 Analyzer (Leco Corporation, St. Joseph, MI, USA).
Microbial Biomass Stoichiometry
Microbial biomass C, N, and P were determined using a sequential extraction procedure modified from Holmes and others (2003). A slurry of approximately 4 g of field moist, homogenized soil with 75 ml of 2 M potassium chloride (KCl) were shaken in a glass Erlenmeyer flask for 1 h. The slurry was filtered through a 0.45 µm Millipore MCE filter, and the filtrate was retained for chemical analysis. The filter and soil were fumigated with ethanol-free chloroform (CHCl3) for 24 h. The soils were extracted again with 2 M KCl, and the filtrates were stored at −20°C in high-density polyethylene bottles.
Microbial biomass C (MBC), N (MBN), and P (MBP) were determined as the total non-purgeable organic carbon (NPOC), total nitrogen (TN), and total P concentrations from the post-chloroform fumigation extractions. NPOC and TN were measured on a Shimadzu TOC-V outfitted with a TNM-1 total nitrogen unit (Shimadzu Corporation, Kyoto, Japan). Microbial biomass C and N were calculated per gram organic matter using correction factors of 0.45 (Joergensen and others 2011) and 0.54 (Brookes and others 1985), respectively. Total P was measured on a Thermo-Electron model iCAP 6300 ICP (Thermo Fisher Scientific, Waltham, MA, USA) at the University of Maine Analytical Laboratory and Maine State Soil Testing Service (Orono, ME, USA). Microbial biomass P was calculated per gram organic matter using a correction coefficient of 0.40 (Brookes and others 1985). Soil labile P is highly operationally defined, and consistent methods are key to allow comparison of relative values within an experiment. Here, we used KCl to determine microbial biomass P as it was simultaneously used in measures of labile microbial biomass N. It should be noted that KCl is less efficient at extracting P than sodium bicarbonate or anion exchange membranes (Brookes and others 1982; Kouno and others 1995), which can be advantageous as an indicator of available soil P because increasingly aggressive extractants use yields from increasingly unavailable soil P phases. Here, we have studied the relative patterns in biomass P across treatments with a consistent methodology, without the intent of direct comparisons with values from other studies using alternative methods.
Enzyme Activity
We measured the activities of three enzymes involved in the hydrolysis of organic compounds to infer C, N, and P limitation of the microbial community including β-1,4-glucosadase (BG), β-1,4-N-acetylglucosaminidase (NAG), and acid phosphatase (AP) (Sinsabaugh and others 2009), with fluorescence substrates 4-MUB-β-glucoside, 4-MUB-N-acetyl-β-d-glucosaminide, and 4-MUB-phosphate, respectively. We did not measure leucine-amino-peptidase (LAP) as test assays resulted in no activity within a 24-h period. LAP activity decreases with soil pH (Sinsabaugh and others 2008), so the lack of activity was likely due to the low pH (range 3.71–4.75) of O horizon soils at BBWM. Frozen soils were thawed at room temperature (~20°C) and moist soil equivalent to approximately 1 g dry mass was sieved and weighed into a clean 50 ml Falcon tube. Slurries were created by adding 30 ml of 50 mM acetate buffer adjusted to pH 5. The slurries were homogenized by vortexing for 1 min and approximately 7 ml of slurry was diluted in an additional 50 ml of buffer and stirred continuously as 200 µl aliquots were removed and added to 96-well plates (7 replicates per sample). Fifty microliters of 1000 µM substrate solution was added to each well, and fluorescence was measured at 25°C on a microplate fluorometer (Fluoroskan Ascent FL, Thermo Fisher Scientific, Waltham, MA, USA). A series of test assays confirmed this substrate concentration saturated enzyme activity (data not shown). Fluorescence was read at 30 min intervals, and activities were calculated from the last linear time point. Activity was normalized to microbial biomass C (μmol g biomass−1 h−1).
To assess changes in microbial nutrient and C limitation, enzyme activities (μmol g biomass−1 h−1) were converted to vectors based on ratios of enzyme activity indicative of C/N (BG/NAG) and C/P (BG/AP) acquisition (sensu Moorhead and others 2013) (Eqs. 1, 2). Vector length, a unit less index of C limitation with longer vectors indicative of increasing C limitation, was calculated as:
Vector angle, in degrees, an index of magnitude of N versus P limitation, was calculated as:
where tan is tangent. Larger vector angles correspond to greater P limitation, whereas smaller angles correspond to greater N limitation.
Statistical Analysis
All analyses were completed in R Version 3.2.2 using RStudio Version 0.99.485 (R Core Team 2015; RStudio Team 2015). Linear mixed effects models were used to detect changes in TC, TN, P-PO4 −, microbial biomass (that is, MBC), biomass stoichiometry (C/N, C/P, and N/P), enzyme activity, vector angle, and vector length in response to short-term P treatment over the course of the experiment using the nlme package Version 3.1-122 (Pinheiro and others 2015). Long-term N treatment (that is, watershed), short-term P treatment, and month were treated as fixed factors. To remove the influence of stand type on covariation among N, P, and seasonal effects, stand type was designated a control variable. Plot was used as a random factor. When there were significant interactions among factors that were not individually significant, we devolved the analysis into one-way ANOVAs to define main effects. Treatments were significantly different at α = 0.05. We were particularly interested in watershed ×+P interactions that would signify that the effect of P enrichment depended on N enrichment. Significant factors were tested using Tukey’s Highly Significant Differences (HSD) test. The effect of main factors on response variable means and p values is presented in the text, whereas a table of mean (±standard deviation) soil chemistry for each combination of main factors is provided in the supplemental material (Table SI 1) along with the outputs of the mixed effects models and ANOVAs (Tables SI 2-8).
Results
Soil Chemistry
Neither N (p = 0.502) nor P treatments (p = 0.571) affected TC. TC did not vary by month (p = 0.312) and was 10% higher in softwood stands than hardwood stands (p = 0.008). Long-term N treatments increased TN in the treated watershed by 7% (p = 0.046). Short-term P addition had no effect on total TN (p = 0.284) and did not interact with N treatment (p = 0.075). TN did not vary over time (p = 0.498). TN was 8% higher in hardwood stands than softwood stands (p = 0.015).
Long-term N treatments did not affect soil PO4 −-P (p = 0.654). P treatment caused a fourfold increase in PO4 −-P (p < 0.001), but this changed over time as indicated by an interaction between P treatment and month (p = 0.008). In June, P addition increased PO4 −-P sevenfold (one-way ANOVA, p < 0.001), and the effect persisted through August, when PO4 −-P was twice as high in P-treated subplots (one-way ANOVA, p = 0.001). By October, there was no difference between No-P and +P subplots (one-way ANOVA, p = 0.409). Stand type did not affect PO4 −-P (p = 0.815).
Microbial Biomass and Enzyme Activity
Microbial biomass, stoichiometry, and N- and P-acquiring enzymes showed a rapid and transient response to short-term P addition independent of experimental N enrichment. MBC increased threefold 1 week after treatment (that is, in June) in both watersheds (Tukey’s HSD, p < 0.001, Figure 1B), but by August average biomass in the P-enriched subplots declined more than threefold (1.11 µg g soil−1) to levels comparable to control subplots (Figure 1B). Short-term P addition did not increase C/N in June (Tukey’s HSD, p = 0.232) or August (Tukey’s HSD, p = 0.999), but in October, C/N in the +P subplots was about twice as high as in the No-P subplots (Tukey’s HSD, p = 0.002, Figure 2). In contrast, C/P followed the same pattern of response to short-term P addition as MBC, with a threefold increase in C/P in June (one-way ANOVA, p = 0.003), but no persistent effect through August (one-way ANOVA, p = 0.461) and October (p = 0.625, Figure 2). Similarly, P addition doubled N/P in June (one-way ANOVA, p = 0.007), but the effect did not persist through August (one-way ANOVA, p = 0.500). Unlike MBC and C/P, in October N/P was four times higher in the No-P subplots compared to the +P subplots (one-way ANOVA, p = 0.014).
Mean ± standard error for C/N vs. C/P. Dashed lines indicate mean C/N and C/P for forest soil microbial biomass (Cleveland and Liptzin 2007): C/N = 8.2 and C/P = 74.0. Solid lines connect points from the same sampling day. Shapes differentiate between N treatments (circle = REF, triangle = + N watershed) and colors differentiate between P treatments (gray = No-P, black = + P).
P addition decreased the activity of the N-acquiring enzyme, NAG, more than 3.5-fold in June (Tukey’s HSD, p < 0.001, Figure 1D), but there was no effect in the following months. P addition reduced the P-acquiring enzyme, AP, in June (threefold decrease, Tukey’s HSD, p < 0.001) and October (1.5-fold decrease, Tukey’s HSD, p = 0.029, Figure 1F) but not August (Tukey’s HSD, p = 0.531).
The only microbial variable consistently responsive to long-term N additions was the C-acquiring enzyme, BG. Mean BG activity was nearly 25% higher in the N-treated watershed compared to the reference watershed across all months (p = 0.038, Figure 1G). BG did not respond to P treatment (p = 0.373, Figure 1H). The long-term N treatment had no effect on MBC (p = 0.245, Figure 1A), C/N (p = 0.747), C/P (p = 0.769), N/P (p = 0.907), NAG (p = 0.942, Figure 1C), or AP (p = 0.111, Figure 1E).
MBC was 1.4 times higher in hardwood stands than softwood stands over all treatments and sampling days (p < 0.001), but there was no effect of stand type on microbial C/N (p = 0.762), C/P (p = 0.760), or N/P (p = 0.812). AP activity was about 1.6 times greater in softwoods stands compared to hardwoods (p < 0.001). There was no difference in BG (p = 0630) or NAG (p = 0.726) between stand types.
Enzyme Vectors
Enzyme vector angles were larger than 45° over all sampling days and treatments, indicating greater P limitation rather than N limitation. Vector angle was not influenced by N (p = 0.357, Figure 3A) or P additions (p = 0.882, Figure 3B). Mean vector angle varied among sampling days (p < 0.001) with the largest vector angle in June (54.6° ± 3.4) and smallest in August (50.6° ± 3.0). Although there was an interaction between day and P addition (p = 0.040), there was no effect of short-term P treatment on vector angle on any day. Vector angle was 3.8% larger in softwoods stands (53.8° ± 3.1) compared to the hardwood stands (51.8° ± 3.6) (p < 0.001).
Enzyme vector angle (A, B) and length (C, D) response to long-term N (open bars) and short-term P (striped bars) additions. Larger vector angle indicates greater P limitation relative to N, and larger vector length indicates greater C limitation. Bars are mean ± standard error. Asterisks indicate significant differences at α = 0.05.
Long-term N treatments increased enzyme vector length, an index of C limitation, by 4.2% (p = 0.031, Figure 3C) over all months. Short-term P addition increased mean enzyme vector length by a similar magnitude (5.6%) over all months (p = 0.006, Figure 3D). Mean vector length varied by month and was >9% greater in October compared to June and August (Tukey’s HSD, p < 0.001, p = 0.002, respectively). There was no difference in vector length between stand types (p = 0.074).
Discussion
Long-Term N Enrichment-Enhanced C Limitation
We expected that long-term experimental N deposition would reduce or alleviate N limitation and enhance P limitation. However, microbial biomass and enzyme activity indicate that soil microbes at BBWM are in a P and C limitation state independently of N status. Furthermore, we found very few interactions between long-term N enrichment and short-term P addition that would have signaled enhanced P limitation under experimental N additions. Long-term N additions at BBWM have increased the soil N pool relative to C, yet there was no effect of N enrichment on microbial biomass, stoichiometry, or N- and P-acquiring enzymes. One of the impacts of atmospheric N deposition is suppression of soil microbial biomass, particularly fungal biomass, in response to reduced fine root and exudate production by plants (Aber and others 1989; Wallenstein and others 2006; Treseder 2008). Phospholipid fatty analysis of soils collected in August 2012 indicated that N additions decreased fungal biomarkers but not total biomarker mass (Tatariw 2016), so it is possible that our fumigation-based biomass carbon measurements, which measure total microbial biomass, do not a reflect a change in fungal biomass.
Mean O horizon microbial C/N was 9.2:1.0 (±5.4), which is slightly higher than the predicted soil microbial C/N of 8.6 (Cleveland and Liptzin 2007). We expected that N deposition would reduce microbial N limitation, resulting in larger vector angles, but although microbes maintained a constant C/N under an increased N regimen, there was no concomitant decrease in NAG activity or increase in AP activity in the treated watershed to indicate a reduction in N acquisition or enhanced P limitation. Although the lack of NAG response is consistent with Mineau and others (2014) and Fatemi and others (2016), who found that long-term N enrichment did not alter microbial nutrient acquisition strategies at BBWM, our findings differ as both BG activity and vector length indicate that long-term N enrichment has enhanced microbial C limitation. BG is associated with cellulose decomposition (Sinsabaugh and Liptak 1997), the breakdown of which, unlike lignin, is N limited (Fog 1988; Berg and Matzner 1997). Enhanced C limitation may also explain the lack of NAG response observed in this and previous (Mineau and others 2014; Fatemi and others 2016) studies at BBWM. Although NAG activity is used as an index of N limitation (for example, Sinsabaugh and others 2009), microbes also use NAG to acquire C from chitin degradation (Geisseler and others 2010).
One of the consequences of an N-driven shift to a more recalcitrant soil pool is an increase in soil C storage which serves as a potential sink for atmospheric CO2 (Janssens and others 2010; Frey and others 2014). The changes in C acquisition strategies that we observed can alter soil C composition in ways that influence soil C storage. For example, increases in BG activity associated with N deposition have been shown to increase lignin relative to cellulose in organic soils (Carreiro and others 2000; Sinsabaugh and others 2005; Talbot and Treseder 2012). Plant response to increased available soil N may also alter the soil carbon pool by reducing labile exudates from rhizosphere priming (Dijkstra and others 2013), further contributing to microbial C limitation. We observed increased C limitation as a result of long-term N enrichments that suggests a more recalcitrant C pool, though direct measurements of organic C availability and rhizosphere C are necessary to confirm the mechanisms of C utilization and limitation at BBWM.
N Enrichment Did Not Enhance P Limitation
The threefold increase in microbial biomass coupled with a threefold decrease in AP activity following P enrichment in both watersheds indicates that microbial communities were P limited independently of long-term N status, possibly due to a combination of biotic and abiotic constraints on P availability such as plant uptake (Yanai 1992) or sorption by secondary soil aluminum and iron hydroxides (Norton and others 2006). It is worth nothing that our enzyme and biomass responses to P addition did not persist throughout the growing season, even though extractable soil PO4 −-P remained elevated for 2 months after treatment. Similarly, plot-scale P fertilization at the Hubbard Brook Experimental Forest (HBEF) increased resin-available P levels, there was no increase in microbial biomass, respiration, or C mineralization two months after treatment (Groffman and Fisk 2011; Minick and others 2011). These findings support the idea that plant uptake and/or abiotic sorption are significant drivers of long-term P availability in northern temperate forest soils.
We did not see a change in vector angle immediately following short-term P addition (that is, June). The C/N ratio of exoenzymes is much lower than that of the microbial biomass (Schimel and Weintraub 2003), so it is possible that at the time of P addition, microbes at BBWM were investing energy into N acquisition to produce AP. This is supported by the fact that NAG activity decreased concomitantly with AP activity and biomass N increased slightly following short-term P addition, as decreased allocation of N toward AP production would lower utilization of cellular N (Olander and Vitousek 2000; Marklein and Houlton 2012; Fisk and others 2014).
In both watersheds, P addition altered microbial biomass stoichiometry by increasing biomass C relative to N and P. It is possible that the reduction in AP and NAG production following P addition decreased microbial use of cellular C (Schimel and Weintraub 2003; Dijkstra and others 2013) and triggered microbial storage of plant exudate C as lipids in biomass (for example, Mooshammer and others 2014). We saw a similar, though smaller, response in biomass stoichiometry and AP activity in the +P subplots in October, possibly due to recycling of added P through litter following senescence (See and others 2015). Although we did not have measurements of litterfall chemistry, the microbial response to P immediately following P addition indicates that P availability is a control on C storage in microbial biomass.
We observed longer vector lengths in +P subplots, suggesting increased microbial demand for C. Phosphorus limits dissolved organic matter mineralization by limiting protein synthesis (Cleveland and others 2003; Hartman and Richardson 2013). For example, Bradford and others (2008) found that P additions increased organic matter decomposition in experimental mesocosms because microbes could produce more decomposing enzymes and ATP, subsequently increasing utilization of recalcitrant C. Increased allocation of cellular P to protein and enzyme synthesis could explain why microbial biomass P did not increase relative to C and N in response to P addition.
Deposition-associated acidification can increase available P in the organic soil horizon by solubilizing aluminum in mineral soil, releasing P which is cycled into organic soil horizons via tree uptake and senescence (SanClements and others 2010). Although experimental acidification had not increased O horizon P at the time of this study, our P addition indicated that an increase in P availability can potentially accelerate microbial nutrient cycling. Estimates of microbial biomass turnover (that is, biomass loss divided by average biomass, McGill and others 1986) show that in addition to increasing total biomass, P addition tripled turnover rates between June and August (0.2 month−1 in No-P vs. 0.6 month−1 in +P). Microbial community turnover is an important source of nutrients for soil organisms and plants (Coleman 1994; Schmidt and others 2007), so P availability at BBWM is potentially an important driver on the timing and availability of microbially derived pools of labile nutrients.
Conclusions
Ecological stoichiometric theory dictates that plasticity in microbial biomass is driven by substrate C/N/P composition and that exogenous enzyme production reflects microbial demand for those nutrients. While there is a clear relationship between substrate stoichiometry, microbial nutrient content, and nutrient acquisition globally (for example, Cleveland and Liptzin 2007; Sinsabaugh and others 2009; Xu and others 2013), regional variation in soil microbial stoichiometry and activity may be more dependent on the quality and timing of C delivery than quantity (Stevenson and others 2016). Measurements of microbial biomass stoichiometry and enzyme activity suggest that long-term N additions have enhanced microbial C limitation, possibly by shifting the soil C pool to a more recalcitrant form. In contrast, P limitation was driven by factors other than N enrichment in the acidic O horizon soils at BBWM. N deposition increases soil C sequestration in northern temperate forests (Nave and others 2009), but long-term storage may be affected due to interacting effects such as P availability (Peñuelas and others 2013), climate change (de Vries and others 2014), and microbial community response to changes in C quality (Bardgett and others 2008; Lehmann and Kleber 2015). Given the complexity of these interactions, it is critical to understand the role of microbial response to changing nutrient availability and the subsequent impact on soil processes in catchments subjected to chronic chemical and physical change.
References
Aber J, Mcdowell W, Nadelhoffer K, Magill A, Berntson G, Kamakea M, Mcnulty S, Currie W, Rustad L, Fernandez I. 1998. Nitrogen saturation in temperate forest ecosystems: hypotheses revisited. BioScience 48:921–34.
Aber J, Nadelhoffer K, Steudler P, Melillo JM. 1989. Nitrogen saturation in northern forest ecosystems: excess nitrogen from fossil fuel combustion may stress the biosphere. BioScience 39:378–86.
Banfield JF, Barker WW, Welch SA, Taunton A. 1999. Biological impact on mineral dissolution: application of the lichen model to understanding mineral weathering in the rhizosphere. Proceedings of the National Academy of Sciences of the United States of America 96:3404–11.
Bardgett RD, Freeman C, Ostle NJ. 2008. Microbial contributions to climate change through carbon cycle feedbacks. Isme J 2:805–14.
Berg B, Matzner E. 1997. Effect of N deposition on decomposition of plant litter and soil organic matter in forest systems. Environmental Reviews 5:1–25.
Bradford MA, Fierer N, Reynolds JF. 2008. Soil carbon stocks in experimental mesocosms are dependent on the rate of labile carbon, nitrogen and phosphorus inputs to soils. Functional Ecology 22:964–74.
Brookes PC, Landman A, Pruden G, Jenkinson DS. 1985. Chloroform fumigation and the release of soil nitrogen: A rapid direct extraction method to measure microbial biomass nitrogen in soil. Soil Biology and Biochemistry 17:837–42.
Brookes PC, Powlson DS, Jenkinson DS. 1982. Measurement of microbial biomass phosphorus in soil. Soil Biology and Biochemistry 14:319–29.
Carreiro MM, Sinsabaugh RL, Repert DA, Parkhurst DF. 2000. Microbial enzyme shifts explain litter decay responses to simulated nitrogen deposition. Ecology 81:2359–65.
Cleveland CC, Liptzin D. 2007. C:N: P stoichiometry in soil: is there a “Redfield ratio” for the microbial biomass? Biogeochemistry 85:235–52.
Cleveland CC, Reed SC, Townsend AR. 2006. Nutrient regulation of organic matter decomposition in a tropical rain forest. Ecology 87:492–503.
Cleveland C, Townsend A, Schmidt S. 2003. Phosphorus limitation of microbial processes in moist tropical forests: Evidence from short-term laboratory inclubations and field studies. Ecosystems 5:680–91.
Coleman DC. 1994. The microbial loop concept as used in terrestrial soil ecology studies. Microbial Ecology 28:245–50.
Crowley KF, McNeil BE, Lovett GM, Canham CD, Driscoll CT, Rustad LE, Ollinger E, Pardo LH, Schaberg PG, Stoddard JL, Weand MP, Weathers KC. 2012. Do nutrient limitation patterns shift from nitrogen toward phosphorus with increasing nitrogen deposition across the northeastern United States? Ecosystems 15:940–57.
Dijkstra FA, Carrillo Y, Pendall E, Morgan JA. 2013. Rhizosphere priming: A nutrient perspective. Frontiers in Microbiology 4:1–8.
Driscoll CT, Lawrence GB, Bulger AJ, Thomas JB, Cronan CS, Eagar CR, Lambert KF, Likens GE, Stoddard JL, Weathers KC. 2001. Acidic deposition in the Northeastern United States: Sources and inputs, ecosystem effects, and management strategies. BioScience 51:180–98.
Eisenlord SD, Freedman Z, Zak DR, Xue K, He Z, Zhou J. 2013. Microbial mechanisms mediating increased soil C storage under elevated atmospheric N deposition. Applied and Environmental Microbiology 79:1191–9.
Elser JJ, Sterner RW, Gorokhova E, Fagan WF, Markow TA, Cotner JB, Harrison JF, Hobbie SE, Odell GM, Weider LW. 2000. Biological stoichiometry from genes to ecosystems. Ecology Letters 3:540–50.
Elvir JA, Rustad L, Wiersma GB, Fernandez I, White AS, White GJ. 2005. Eleven-year response of foliar chemistry to chronic nitrogen and sulfur additions at the Bear Brook Watershed in Maine. Canadian Journal of Forest Research 35:1402–10.
Falkowski PG, Fenchel T, Delong EF. 2008. The microbial engines that drive earth’s biogeochemical cycles. Science 320:1034–9.
Fatemi FR, Fernandez IJ, Simon KS, Dail DB. 2016. Nitrogen and phosphorus limitations to soil enzyme activities in acid forest soils. Soil Biology and Biochemistry 98:171–9.
Fatemi FR, Fernandez IJ, Szillery J, Norton SA, Rustad LE. 2012. Soil solution chemical response to two decades of experimental acidification at the Bear Brook Watershed in Maine. Water, Air, & Soil Pollution 223:6171–86.
Fernandez IJ, Adams MB, SanClements MD, Norton SA. 2010. Comparing decadal responses of whole-watershed manipulations at the Bear Brook and Fernow experiments. Environmental Monitoring and Assessment 171:149–61.
Fernandez IJ, Karem JE, Norton SA, Rustad LE. 2007. Temperature, soil moisture, and streamflow at the Bear Brook Watershed in Maine (BBWM). Maine Agricultural and Forest Expeirment Station Technical Bulletin 196.
Fisk MC, Ratliff TJ, Goswami S, Yanai RD. 2014. Synergistic soil response to nitrogen plus phosphorus fertilization in hardwood forests. Biogeochemistry 118:195–204.
Fog K. 1988. The effect of added nitrogen on the rate of decomposition of organic matter. Biological Reviews 63:433–62.
Frey SD, Knorr M, Parrent JL, Simpson RT. 2004. Chronic nitrogen enrichment affects the structure and function of the soil microbial community in temperate hardwood and pine forests. Forest Ecology and Management 196:159–71.
Frey SD, Ollinger S, Nadelhoffer K, Bowden R, Brzostek E, Burton A, Caldwell BA, Crow S, Goodale CL, Grandy AS, Finzi A, Kramer MG, Lajtha K, LeMoine J, Martin M, McDowell WH, Minocha R, Sadowsky JJ, Templer PH, Wickings K. 2014. Chronic nitrogen additions suppress decomposition and sequester soil carbon in temperate forests. Biogeochemistry 121:305–16.
Gallardo A, Schlesinger WH. 1994. Factors limiting microbial biomass in the mineral soil and forest floor of a warm-temperate forest. Soil Biology and Biochemistry 26:1409–15.
Galloway JN, Townsend AR, Erisman JW, Bekunda M, Cai Z, Freney JR, Martinelli LA, Seitzinger SP, Sutton MA. 2008. Transformation of the nitrogen cycle: recent trends, questions, and potential solutions. Science 320:889–92.
Geisseler D, Horwath WR, Joergensen RG, Ludwig B. 2010. Pathways of nitrogen utilization by soil microorganisms: A review. Soil Biology and Biochemistry 42:2058–67.
Groffman PM, Fisk MC. 2011. Phosphate additions have no effect on microbial biomass and activity in a northern hardwood forest. Soil Biology and Biochemistry 43:2441–9.
Hartman WH, Richardson CJ. 2013. Differential nutrient limitation of soil microbial biomass and metabolic quotients (qCO2): Is there a biological stoichiometry of soil microbes? PloS One 8:1–14.
Hinsinger P. 2001. Bioavailability of soil inorganic P in the rhizosphere as affected by root-induced chemical changes: a review. Plant and Soil 237:173–95.
Hobbie S, Vitousek P. 2000. Nutrient limitation of decomposition in Hawaiian forests. Ecology 81:1867–77.
Holmes WE, Zak DR, Pregitzer KS, King JS. 2003. Soil nitrogen transformations under Populus tremuloides, Betula papyrifera and Acer saccharum following 3 years exposure to elevated CO2 and O3. Global Change Biology 9:1743–50.
Janssens IA, Dieleman W, Luyssaert S, Subke J-A, Reichstein M, Ceulemans R, Ciais P, Dolman AJ, Grace J, Matteucci G, Papale D, Piao SL, Schulze E-D, Tang J, Law BE. 2010. Reduction of forest soil respiration in response to nitrogen deposition. Nature Geoscience 3:315–22.
Joergensen RG, Wu J, Brookes PC. 2011. Measuring soil microbial biomass using an automated procedure. Soil Biology and Biochemistry 43:873–6.
Kouno K, Tuchiya Y, Ando T. 1995. Measurement of soil microbial biomass phosphorus by an anion exchange membrane method. Soil Biology and Biochemistry 27:1353–7.
Leff JW, Jones SE, Prober SM, Barberán A, Borer ET, Firn JL, Harpole WS, Hobbie SE, Hofmockel KS, Knops JMH, McCulley RL, La Pierre K, Risch AC, Seabloom EW, Schütz M, Steenbock C, Stevens CJ, Fierer N. 2015. Consistent responses of soil microbial communities to elevated nutrient inputs in grasslands across the globe. Proceedings of the National Academy of Sciences 112:10967–72.
Lehmann J, Kleber M. 2015. The contentious nature of soil organic matter. Nature 528:60–8.
Lovett GM, Arthur MA, Weathers KC, Fitzhugh RD, Templer PH. 2013. Nitrogen addition increases carbon storage in soils, but not in trees, in an eastern US deciduous forest. Ecosystems 16:980–1001.
Lynch JP, Ho MD. 2005. Rhizoeconomics: Carbon costs of phosphorus acquisition. Plant and Soil 269:45–56.
Manzoni S, Taylor P, Richter A, Porporato A, Ågren GI. 2012. Environmental and stoichiometric controls on microbial carbon-use efficiency in soils. New Phytologist 196:79–91.
Marklein AR, Houlton BZ. 2012. Nitrogen inputs accelerate phosphorus cycling rates across a wide variety of terrestrial ecosystems. New Phytologist 193:696–704.
McGill WB, Cannon KR, Robertson JA, Cook FD. 1986. Dynamics of soil microbial biomass and water-soluble organic C in Breton L after 50 years of cropping to two rotations. Canadian Jouranl of Soil Science 66:1–19.
McGroddy M, Silver W, Cosme de Oliveira R Jr. 2004. The effect of phosphorus availability on decomposition dynamics in a seasonal lowland Amazonian forest. Ecosystems 7:172–9.
Mineau MM, Fatemi FR, Fernandez IJ, Simon KS. 2014. Microbial enzyme activity at the watershed scale: response to chronic nitrogen deposition and acute phosphorus enrichment. Biogeochemistry 117:131–42.
Minick KJ, Fisk MC, Groffman PM. 2011. Calcium and phosphorus interact to reduce mid-growing season net nitrogen mineralization potential in organic horizons in a northern hardwood forest. Soil Biology and Biochemistry 43:271–9.
Moorhead DL, Rinkes ZL, Sinsabaugh RL, Weintraub MN. 2013. Dynamic relationships between microbial biomass, respiration, inorganic nutrients and enzyme activities: Informing enzyme-based decomposition models. Frontiers in Microbiology 4:1–12.
Mooshammer M, Wanek W, Zechmeister-Boltenstern S, Richter A. 2014. Stoichiometric imbalances between terrestrial decomposer communities and their resources: Mechanisms and implications of microbial adaptations to their resources. Frontiers in Microbiology 5:1–10.
Naples BK, Fisk MC. 2010. Belowground insights into nutrient limitation in northern hardwood forests. Biogeochemistry 97:109–21.
Nave LE, Vance ED, Swanston CW, Curtis PS. 2009. Impacts of elevated N inputs on north temperate forest soil C storage, C/N, and net N-mineralization. Geoderma 153:231–40.
Norton S, Fernandez IJ, Amirbahman A, Coolidge K, Navrátil T. 2006. Aluminum, phosphorus, and oligotrophy–Assuembling the pieces of the puzzle. Verhandlungen der Internationalen Vereinigung für Theoretische und Angewandte Limnologie 29:1877–86.
Olander LP, Vitousek PM. 2000. Regulation of soil phosphatase and chitinase activity by N and P availability. Biogeochemistry 49:175–90.
Peñuelas J, Poulter B, Sardans J, Ciais P, van der Velde M, Bopp L, Boucher O, Godderis Y, Hinsinger P, Llusia J, Nardin E, Vicca S, Obersteiner M, Janssens IA. 2013. Human-induced nitrogen-phosphorus imbalances alter natural and managed ecosystems across the globe. Nature communications 4:2934.
Pinheiro J, Bates D, DebRoy S, Sarkar D, R. C. Team. 2015. nlme: Linear and Nonlinear Mixed Effects Models. R Foundation for Statistical Computing.
Porder S, Vitousek PM, Chadwick OA, Chamberlain CP, Hilley GE. 2007. Uplift, erosion, and phosphorus limitation in terrestrial ecosystems. Ecosystems 10:158–70.
Pregitzer KS, Burton AJ, Zak DR, Talhelm AF. 2008. Simulated chronic nitrogen deposition increases carbon storage in Northern Temperate forests. Global Change Biology 14:142–53.
R Core Team. 2015. R: A language and environment for statistical computing. Vienna: R Foundation for Statistical Computing.
RStudio Team. 2015. RStudio: Integrated Development for R. Boston, MA: RStudio Inc.
Salvino CJ. 2014. Is nitrogen cycling and bioavailability limited by phosphorus in northern New England forest soils?. Orono: University of Maine.
SanClements MD, Fernandez IJ, Norton SA. 2010. Phosphorus in soils of temperate forests: Linkages to acidity and aluminum. Soil Science Society of America Journal 74:2175.
Schimel JP, Weintraub MN. 2003. The implications of exoenzyme activity on microbial carbon and nitrogen limitation in soil: a theoretical model. Soil Biology and Biochemistry 35:549–63.
Schlesinger WH, Bernhardt ES. 2013. The global cycles of nitrogen and phosphorus Biogeochemistry an analysis of global change. 3rd edn. Waltham (MA): Academic Press, Inc. pp 445–67.
Schmidt SK, Costello EK, Nemergut DR, Cleveland CC, Reed SC, Weintraub MN, Meyer AF, Martin AM. 2007. Biogeochemical consequences of rapid microbial turnover. Ecology 88:1379–85.
See CR, Yanai RD, Fisk MC, Vadeboncoeur MA, Quintero BA, Fahey TJ. 2015. Soil nitrogen affects phosphorus recycling: foliar resorption and plant-soil feedbacks in a northern hardwood forest. Ecology 96:2488–98.
Simon KS, Chadwick MA, Huryn AD, Valett HM. 2010. Stream ecosystem response to chronic deposition of N and acid at the Bear Brook Watershed, Maine. Environmental Monitoring and Assessment 171:83–92.
Sinsabaugh RL, Gallo ME, Lauber C, Waldrop MP, Zak DR. 2005. Extracellular enzyme activities and soil organic matter dynamics for northern hardwood forests receiving simulated nitrogen deposition. Biogeochemistry 75:201–15.
Sinsabaugh RL, Hill BH, Follstad Shah JJ. 2009. Ecoenzymatic stoichiometry of microbial organic nutrient acquisition in soil and sediment. Nature 462:795–8.
Sinsabaugh RL, Lauber CL, Weintraub MN, Ahmed B, Allison SD, Crenshaw C, Contosta AR, Cusack D, Frey S, Gallo ME, Gartner TB, Hobbie SE, Holland K, Keeler BL, Powers JS, Stursova M, Takacs-Vesbach C, Waldrop MP, Wallenstein MD, Zak DR, Zeglin LH. 2008. Stoichiometry of soil enzyme activity at global scale. Ecology Letters 11:1252–64.
Sinsabaugh RL, Liptak M. 1997. Enzymatic conversion of plant biomass. In: Soderstrom B, Wicklow DT, Eds. The Mycota: Environmental and Microbial Relationships. Berlin, Germany: Springer. p 347–57.
Stevenson BA, Sarmah AK, Smernik R, Hunter DWF, Fraser S. 2016. Soil carbon characterization and nutrient ratios across land uses on two contrasting soils: Their relationships to microbial biomass and function. Soil Biology and Biochemistry 97:50–62.
Talbot JM, Treseder KK. 2012. Interactions among lignin, cellulose, and nitrogen drive litter chemistry-decay relationships. Ecology 93:345–54.
Tatariw C. 2016. The impact of anthropogenic disturbance on soil microbial community composition and activity: Implications for ecosystem function. Orono: University of Maine.
Treseder KK. 2008. Nitrogen additions and microbial biomass: A meta-analysis of ecosystem studies. Ecology Letters 11:1111–20.
Vitousek PM, Porder S, Houlton BZ, Chadwick OA. 2010. Terrestrial phosphorus limitation: mechanisms, implications, and nitrogen: phosphorus interactions. Ecological Applications 20:5–15.
de Vries W, Du E, Butterbach-Bahl K. 2014. Short and long-term impacts of nitrogen deposition on carbon sequestration by forest ecosystems. Current Opinion in Environmental Sustainability 9:90–104.
Waldrop MP, Zak DR, Sinsabaugh RL, Gallo M, Lauber C. 2004. Nitrogen deposition modifies soil carbon storage through changes in microbial enzymatic activity. Ecological Applications 14:1172–7.
Wallenstein MD, McNulty S, Fernandez IJ, Boggs J, Schlesinger WH. 2006. Nitrogen fertilization decreases forest soil fungal and bacterial biomass in three long-term experiments. Forest Ecology and Management 222:459–68.
Xu X, Thornton PE, Post WM. 2013. A global analysis of soil microbial biomass carbon, nitrogen and phosphorus in terrestrial ecosystems. Global Ecology and Biogeography 22:737–49.
Yanai RD. 1992. Phosphorus Budget of a 70-Year-Old Northern Hardwood Forest. Biogeochemistry 17:1–22.
Acknowledgements
We thank Cheryl Spencer for her invaluable assistance coordinating soil collection and processing. Derek Rothenheber, Tyler Coleman, and Nick Lawson were instrumental in acquiring biomass and enzyme assay data. Drs. Farrah Fatemi, Madeliene Mineau, and Thomas Parr provided guidance for optimizing the enzyme protocol. This research was funded by NSF DEB-1119709 and DEB-1056692 awarded to I.J.F., J.D.M, and K.S. Additional laboratory analyses were provided by the University of Maine Analytical Laboratory and Maine Soil Testing Service. We thank the 2 anonymous reviewers for their constructive and insightful feedback. This project was supported by the USDA National Institute of Food and Agriculture, Hatch project #ME0-41507. This is a Maine Agricultural and Forest Experiment Station Publication.
Author information
Authors and Affiliations
Corresponding author
Electronic supplementary material
Below is the link to the electronic supplementary material.
Rights and permissions
About this article
Cite this article
Tatariw, C., MacRae, J.D., Fernandez, I.J. et al. Chronic Nitrogen Enrichment at the Watershed Scale Does Not Enhance Microbial Phosphorus Limitation. Ecosystems 21, 178–189 (2018). https://doi.org/10.1007/s10021-017-0140-1
Received:
Accepted:
Published:
Issue Date:
DOI: https://doi.org/10.1007/s10021-017-0140-1