Abstract
The toxicity of three antifoulants (Sea-Nine, Irgarol, and TBT) was determined individually and in mixtures in two tests with microalgae. Effects on periphyton community photosynthesis and reproduction of the unicellular green algae Scenedesmus vacuolatus were investigated. The tested antifoulants were highly toxic in both tests. Observed mixture toxicities were compared with predictions derived from two concepts: Independent Action (IA), assumed to be more relevant for the tested mixtures that were composed of dissimilarly acting substances, and Concentration Addition (CA), regarded as a reasonable worst-case approach in predictive mixture hazard assessment. Despite the corresponding mechanistic basis, IA failed to provide accurate predictions of the observed mixture toxicities. Results show the same pattern in both assays. Mixture effects at high concentrations were slightly overestimated and effects at low concentrations were slightly underestimated. Maximum observed deviations between observed and IA-predicted concentrations amount to a factor of 4. The suggested worst-case approach using CA was protective only in effect regions above 20%. Nevertheless, the application of any concept that accounts for possible mixture effects is more realistic than the present chemical-by-chemical assessment.
Similar content being viewed by others
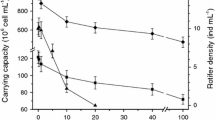
Explore related subjects
Discover the latest articles, news and stories from top researchers in related subjects.Avoid common mistakes on your manuscript.
Antifouling agents are used in paint to prevent the growth of fouling organisms on ship hulls. Marine biofouling can be defined as the undesirable accumulation of microrganisms, such as bacteria and microalgae, plants and invertebrates on artificial surfaces submerged in seawater (Yebra et al. 2004). The active ingredients in the paints leach from the coating and thereby become available to the fouling organisms, but may also escape from the treated surface and contaminate the aquatic environment. A variety of biocides are currently used in boat bottom paints, e.g., TBT, Irgarol 1051, diuron, Sea-Nine 211, cuprous oxide, chlorothalonil, zinc pyrithione, and dichlofluanid (Konstantinou and Albanis 2004). Thus, in areas with high boating activities, many of these substances occur as mixtures (Biselli et al. 2000, Martinez et al. 2000, 2001, Thomas 2001, Thomas et al. 2000, 2001, 2003; Lambropolou et al. 2002, Lamoree et al. 2002, Mezuca et al. 2002, Sakkas et al. 2002).
TBT (tri-butyl-tin), Irgarol (2-tert-butylamino)-4-(cyclopropylamino)-6-(methylthio)-1,3,5-triazine), and Sea-Nine (4,5-dichloro-2-n-octyl-4-isothiazolin-3-one; DCOIT) were selected for testing because they have been detected in coastal environments, and are highly toxic to algae. Algal communities are affected by the individual compounds at subnanomolar concentrations as demonstrated for TBT (Molander et al. 1992, Blanck and Dahl 1996, Dahl and Blanck 1996a, Fent 1996), Irgarol (Dahl and Blanck 1996b, Grönvall et al. 2001, Berard et al. 2003), and Sea-Nine (Larsen et al. 2003; Arrhenius, unpublished data). Due to low degradation rates in sediments and prolonged use until 2008 (IMO 2001), TBT is found in high concentrations in the environment: e.g., 18 nmol/g were measured in sediments (Thomas et al. 2000), and up to 10 nM in coastal waters (Basheer et al. 2002). The herbicide Irgarol was introduced after the restrictions on using TBT on pleasure boats and is mainly used in combination with copper. Increasing concentrations of Irgarol in coastal waters, up to 12–17 nM (Basheer et al. 2002), have been reported. Sea-Nine was introduced as an environmentally friendlier alternative mainly due to its reported rapid degradation. Published half-lives range from less than one hour (Shade et al. 1993) to more than a week (Callow and Finlay 1995; Callow et al. 1996). Nevertheless, fairly high concentrations of Sea-Nine have been analysed in coastal waters with peaks of about 10 nM (Martinez et al. 2001).
The mechanisms of action of the selected antifouling agents in algae are different. Irgarol is the only specifically phytotoxic compound and inhibits photosystem II by binding to the D1 protein (Hall et al. 1999). TBT interferes with energy metabolism in chloroplasts and mitochondria but it is also shown to interact with proteins and membranes and binds to or interacts with any protein containing free sulfhydryl groups (Fent 1996). The mechanism of action of the isothiazolinone Sea-Nine is still not clearly established. However, other, more water-soluble representatives from the so-called Kathon group of biocides quickly penetrate cell membranes and inhibit specific enzymes in the cell by reacting with intracellular thiols (Collier et al. 1990a, b, 1991). Sea-Nine also seems to be able to affect more than one thiol group by generating a cascade of intracellular radicals (Diehl et al. 1999).
In the present study, we intended to assess the possible hazard of antifoulants under environmentally realistic settings. For this purpose, the effects of TBT, Irgarol, and Sea-Nine on microalgal communities were studied both individually and in mixtures. Marine microalgal communities living attached to glass discs, so-called periphyton (Blanck and Wängberg 1988, Dahl and Blanck 1996a, Arrhenius et al. 2004), were used as test organisms. These discs (1.5 cm2) may be colonized by about 30 (or more) different algal species each and are generally dominated by diatoms (Arrhenius, unpublished data). Short-term inhibition of community photosynthesis using radiolabelled carbonate (14C) was used as effect indicator. This test detects acute effects on the photosynthetic processes only. Since TBT and Sea-Nine are broader in their effect spectrum, other processes than photosynthesis may be relevant for the toxicity. To improve detection of such effects, the short-term periphyton test was complemented with an algal reproduction test (Faust et al. 2001) with a more integrative endpoint.
As the occurrence of antifouling agents in the marine environment varies both qualitatively and quantitatively, the experimental testing of every mixture is simply impossible. Consequently, modelling approaches for prediction of mixture toxicity are needed. Two major concepts, Concentration Addition (Loewe and Muischnek 1926, Loewe 1927) and Independent Action (Bliss 1939), are available to describe the mixture toxicity on the basis of known toxicities of the individual components in the mixture (Greco et al. 1992; Altenburger et al. 2003). The general idea is that the mixture toxicity of substances with a common target site and a similar mechanism of action can be described by the concept of Concentration Addition (CA) and substances with dissimilar mechanisms of action and different target sites by the concept of Independent Action (IA). Thus, both concepts are a priori suitable for a predictive assessment of mixture toxicity. The concepts are reported in the literature also under various other names such as bliss independence, effect multiplication, and response addition for IA, and Loewe additivity for CA (Greco et al. 1992, Altenburger et al. 2003).
Both concepts describe a “non-interactive” type of joint action (Plackett and Hewlett 1952), i.e., they presume that neither toxicokinetic nor toxicodynamic interactions between the mixture components are present. More advanced versions of CA and IA may overcome these conceptual limitations, e.g., by modelling specific interactions. These cases are usually classified as “interactive” joint action (Plackett and Hewlett 1967). However, these advanced models are of limited use for a general predictive mixture toxicity assessment, as they require profound knowledge of the type of interaction in the studied mixture, which is not available for the tested antifouling compounds.
CA and IA have been applied successfully in mixture toxicity studies with single species tests. CA was shown to accurately predict the mixture toxicity of similarly acting substances, e.g., with the marine bacterium Vibrio fischeri (Altenburger et al. 2000, Backhaus et al. 2000b), the unicellular green freshwater alga Scenedesmus vacuolatus (Faust et al. 2001), the water flea Daphnia magna (Hermens et al. 1984) and guppies (Poecilia reticulata) (Könemann 1980, 1981; Hermens and Leeuwangh 1982, Hermens 1984). IA has been demonstrated to accurately predict the mixture toxicity of dissimilarly acting substances in tests with Vibrio fischeri and Scenedesmus vacuolatus (Backhaus et al. 2000a; Faust et al. 2003).
First attempts to apply CA and IA at the community level show that the concepts are indeed applicable also at this higher level of biological complexity: CA accurately predicted the toxicity of 12 congeneric similar-acting phenylurea herbicides to marine periphyton and epipsammon (biota living more or less attached to grains of sand) algal communities (Arrhenius et al. 2004). However, limits of IA became obvious in a mixture study with dissimilarly acting substances and epipsammon (Backhaus et al. 2004). Two of the mixture components, as well as the mixture, showed hormesis-like effects, which could not be adequately described by IA (nor CA) due to inherent limitations of the concepts.
As the mechanisms of action of the antifouling agents in this study are dissimilar, we expect IA to be more accurate for the prediction of mixture toxicity. However, in order to explore the suggested application of CA as a reasonable worst-case approach in predictive mixture hazard assessment (Boedeker et al. 1993, Backhaus et al. 2000a, b, Faust et al. 2000, 2003), CA was also evaluated.
In the present report, we set out to (1) experimentally test the individual and mixture toxicity of three dissimilarly acting antifouling agents in two algal tests on different levels of biological complexity, and (2) compare the observed mixture toxicity with the predicted mixture toxicity according to IA and CA. Implications for predictive hazard assessment of mixtures of antifouling agents will be discussed.
Materials and Methods
Test Chemicals, Solutions, and Chemical Analyses
The purity, CAS registry numbers, molecular weight, and the source of the antifouling biocides TBT, Irgarol, and Sea-Nine are given in Table 1. Stock solutions of each toxicant were prepared in acetone (for the periphyton community test) or methanol (for the algal reproduction test) and stored at −20°C. For the periphyton community test, aqueous test solutions were prepared in filtered (Whatman GF/F) seawater with acetone as a co-solvent (final concentration 25 μL/L). For the algal reproduction test, aliquots of the organic stock solutions were first placed under a stream of nitrogen gas to evaporate the organic solvent. Aqueous stock solutions were then prepared by re-dissolving the test substance in algal growth medium (Grimme and Boardman 1972) under vigorous stirring at room temperature overnight.
Concentrations of Irgarol and Sea-nine in water were checked by reversed-phase high performance liquid chromatography (rp-HPLC) using an UV detector at wavelengths of 226 nm (Irgarol) and 283 nm (Sea-Nine). A LiChrosphere RP 18e (Merck, Darmstadt, Germany) column (125 mm length, 4 mm inner diameter, 5 μm particle size) was used as stationary phase. The mobile phases consisted of acetonitrile/water mixtures; 50/50% (v/v) for Irgarol and 60/40% (v/v) for Sea-Nine. Irgarol and Sea-Nine proved to be stable under the test conditions of the algal reproduction assay (data not shown).
TBT chloride concentrations could not be checked analytically by using UV-detection. In order to minimise the likelihood of different exposure conditions in single substance and mixture tests, the same single aqueous stock solution was used for all tests. Additionally, we conducted re-tests after 24 h incubation under test conditions. As the observed toxicity after re-testing was comparable to the initial toxicity (data not shown), we conclude that the solutions were stable over the testing period.
As no significant degradation of the substances was proven in the algal reproduction test that was run for 24 h, no significant degradation was expected in the periphyton community test that was run for only 45 min. Furthermore, all test conditions in both assays were the same during the single substance and mixture tests. We, therefore, assume an identical exposure of the algae to the substances in the single substance and mixture experiments. Hence, the mixture predictions should be valid irrespective of degradation or any other chemical characteristics that would influence the concentration of the substances.
Inhibition of Periphyton Community Photosynthesis
The periphyton studies were conducted at Kristineberg Marine Research Station (58°15′N, 11°27′E) by the Gullmar fjord on the Swedish west coast. Periphyton were sampled from the Kalvhagefjorden site (station no 5 in Blanck and Dahl, 1996), using artificial substrata (circular glass discs, 1.5 cm2) mounted on polyethylene holders (Blanck and Wängberg 1988). Discs were gently cleaned on all but the colonised side and atypical ones were excluded from further testing. In this way, a more homogenous population of glass discs was obtained.
The inhibition of photosynthesis was measured using 14C-technique as described previously (Blanck and Wängberg 1988; Arrhenius et al. 2004). In brief, the algal communities were incubated at ambient temperature and with a light intensity of about 100 μmol photons/m2/s with the toxicant in a total volume of 2 mL test solution. After 30 min of pre-incubation with the toxicant, radiolabelled carbonate (2 μCi per sample) was added and allowed to incorporate for another 15 min. The incorporation was terminated, after a total of 45 min, with formaldehyde (final concentration 2%, v/v). The samples were acidified with concentrated hydrochloric acid (100 μL) and the amount of 14C incorporated into acid-stable components was measured using a liquid scintillation counter.
The studies were conducted at the beginning of September 1999. In order to maintain comparable testing conditions, the likelihood for systematic changes in the algal communities was minimised by carrying out the single substance experiments within two days followed by me mixture experiments within three days.
Inhibition of Algal Reproduction
Effects on the reproduction of a synchronically grown culture of the endospore-forming green alga Scenedesmus vacuolatus strain 211-15 (culture collection of the University of Göttingen, Germany) were studied after 24 h incubation (one generation cycle) according to the method described by Faust et al. (2001). The relative inhibition of reproduction was determined as a reduced cell number of the treated samples in relation to the controls, using a particle counter (Coulter Electronics, Bedford, UK).
Concentration-Response Analyses
Concentration-response analyses were performed in the same way for all individual toxicants and for the mixtures. A geometric dilution series was used with the concentration intervals adjusted to the steepness of the expected concentration-response curve based on information from previous range-finding experiments. The relative inhibition of photosynthetic activity (periphyton test) and the relative inhibition of algal reproduction (algal reproduction test) were calculated using at least 10 or 6 untreated controls and 5 or 3 replicates per treatment, respectively.
Due to the occasional appearance of outliers in the controls, the relative inhibition of photosynthetic activity (periphyton test) was always calculated on the basis of the trimmed mean of the photosynthetic activity in all untreated controls (n = 10), i.e., the lowest and the highest individual control measurement were discarded. The trimmed control mean is obviously less susceptible to the effects of extreme measurements than is the arithmetic mean.
Concentration-response functions were statistically determined by applying the best-fit procedure by Scholze and co-workers (Scholze et al. 2001). With this approach, ten different regression models were applied to each data set in order to determine, on the basis of statistical criteria, the regression model that best described the observed data. Although the improvements in accuracy by this best-fit procedure usually are negligible for median effect levels, they become more pronounced in the upper and lower parts of the curve. As we were interested in the description of the complete concentration-response curves for the components and the mixtures, the best- fit procedure was used in order to increase the accuracy of the concentration-response assessment in the upper and lower parts of the curves. Regression-based effect concentrations (ECx) were derived from the fitted concentration-response regression models, and the corresponding 95% confidence intervals were estimated using a bootstrap approach (Scholze et al. 2001).
Analysis of Mixture Toxicities
For the analysis of mixture toxicities, a fixed-ratio design was used. This means that the ratio of the mixture components was kept constant while the overall concentration of the mixture was systematically varied. The resulting concentration-response relationship can thus be biometrically analysed analogous to the single substances. The mixture experiments were designed to accurately describe the range between 10 and 90% effect. Within this range, reliable estimates of the single substances toxicities were available for predictions of mixture toxicity. In order to avoid that only one or two toxicants would dominate the mixture toxicity, the components were mixed in the ratio of their individual EC50 values (“EC50-mixture”). According to CA, all components contribute equally to the CA-predicted EC50 in such a mixture.
The algal reproduction assay and the periphyton photosynthesis test differed considerably in their sensitivities. Hence, two different molar mixture ratios were used, each corresponding to the EC50 ratio in the corresponding assay.
The ternary mixture in the algal reproduction test was, unintentionally, tested in a mixture ratio slightly different from the planned EC50 mixture ratio (molar mixture ratio Sea-Nine:TBT:Irgarol was 0.624:0.341:0.035 instead of 0.570:0.390:0.040). The former, actually tested, mixture ratio was used for calculations of mixture toxicity according to CA and IA in the algal reproduction test.
Calculations of CA and IA Predictions
Mathematically CA can be formulated as
where n is the number of mixture components, ECx i is the concentration of the ith mixture component that provokes x% effect when applied singly, and c i is the concentration of the respective component in the mixture. Each fraction of c i /ECx i represents the concentration of a mixture component scaled for its relative toxicity and is generally termed the “toxic unit” of that component.
For the calculation of predicted effect concentrations according to CA, equation 1 can be reformulated to:
where ECx i is the concentration of the ith mixture component that provokes x% effect when applied singly, ECx mix is the total concentration of the mixture provoking x% effect, and p i denotes the fraction of component i in the mixture. Concentrations of each mixture predicted to give 10 to 90% effects were calculated in steps of 1% and the resulting concentration/effect pairs were plotted and connected with lines to give a visualization of the predicted concentration-response curve.
Mixture toxicity according to IA is based on the effects of the components and can be calculated by:
where E(c mix ) denotes the effect (scaled from 0–1) of an n-compound mixture, c i is the concentration of the ith compound, and E(c i ) is the effect of that concentration if the compound is applied singly. Accordingly, if the components of a mixture are present in concentrations at which they do not produce an effect when applied singly, i.e., E(c i ) = 0, the mixture toxicity is predicted to be zero.
Mixture effects according to IA can be directly calculated using equation 3, but effect concentrations only through an iterative procedure (Faust et al. 2003). However, for the experimental planning of the concentration range for mixture effects that would be appropriate, a simplified way of calculating the IA prediction may be used (Backhaus et al. 2000a). After the calculation of the mixture effect concentrations ECx mix for CA (Eq. 2), the corresponding concentration c i of each individual component present at ECx mix can be determined, as the mixture ratio is known. The effect E(c i ) that each component would provoke individually at c i can then be determined, using the corresponding concentration-response relationship. These values were then used in equation 3 for the calculation of the IA-predicted mixture effect E(c mix ). Again, for the visualisation of the concentration-response curve, all concentration/effect pairs were plotted and connected with lines. More details on the calculations of CA and IA based predictions are given elsewhere (Faust et al. 2001, 2003).
Mean effects and effect concentrations of individual substances are subject to a stochastic variability. Consequently, the calculation of a prediction according to CA or IA has to produce a mean that is also affected by statistical uncertainty. This uncertainty was quantified by approximating the central 95% confidence intervals for the mean effect and effect concentrations for CA and IA by using the bootstrap concept (Efron and Tibshirani 1993). The bootstrap samples were generated on the basis of the effect distributions that were previously estimated in the fitting process for every individual concentration response function (parametric bootstrap) (Scholze et al. 2001). The non-overlapping of the central 95% confidence intervals obtained for the means was used as a criterion for judging effect differences as statistically significant. All computations were programmed using Proc Nlin of SAS for Windows, version 8.02 (SAS Institute, Cary, USA). The no-observed-effect concentrations (NOECs) were determined using Dunnett’s test (Dunnett 1964).
Results
Single Substances
TBT, Irgarol, and Sea-Nine were highly toxic in both algal tests, with EC50 values ranging from 4 nM (Irgarol) to 550 nM (Sea-Nine). All single substance data, regression models, model parameters, and ECX values are given in Table 2. For a graphical comparison of the observed toxicities, the corresponding fits are presented in Figure 1. At the EC50, the periphyton community photosynthesis was more sensitive to TBT and Irgarol (by a factor of 3.6 and 5.5, respectively) than was the reproduction of Scenedesmus vacuolatus. Periphyton photosynthesis was slightly less sensitive to Sea-Nine than was algal reproduction.
Typical experimental concentration-response data for each biotest are shown for Sea-Nine together with the corresponding regression fits in Figure 2. It can be observed that the effect data variability was typically higher in the community test than in the single species test, with an average coefficient of variation for the controls of 7% (n = 6) in the algal reproduction test and 22% (n = 10) in the periphyton test. This was expected, as a higher variation is often observed in community level tests due to the higher biological complexity relative to single species tests, as well as to the patchiness of the attached periphyton communities.
Concentration-response relationships for Sea-Nine observed in the periphyton photosynthesis (A) and algal reproduction (B) test. Horizontal dotted lines indicate the 95% confidence limits of the control mean (n = 9 in A and n = 12 in B). Solid circles and triangles represent Sea-Nine treatments (n = 5 in A and two pooled experiments n = 3+3 in B). Thick solid lines indicate the regression fits of the observations (Table 2)
Three Component Mixtures
The mixtures were tested in the ratio of the individual EC50-values. As these differed between the two tests, different molar ratios were used. The ratio Sea-Nine:TBT:Irgarol was 0.8969:0.0964:0.0067 in the periphyton test while it was 0.624:0.341:0.035 for the algal reproduction test. The observed EC50 values of the mixtures differed slightly between the two biotests: 503 nM in the periphyton test and 403 nM in the algal reproduction test (Table 2). The variability observed in effect data of the mixture experiments was comparable to that observed in the single substances tests (data not shown).
Both IA and CA predictions overestimated the toxicity of the ternary mixtures for effects higher than 40% and underestimated it for effects below 20% (Fig. 3). The shapes of the prediction curves were clearly different from the observed concentration-response curves; hence, both IA and CA failed to make accurate predictions of the concentration-response curves for the mixtures.
Observed and predicted mixture toxicity according to CA and IA of TBT, Irgarol, and Sea-Nine to periphyton photosynthesis and algal reproduction. The substances are mixed in the ratio of their individual EC50 values. Dots show the mean of the observed toxicity with 95% confidence interval (A: n = 4–5; B: n = 6); thin solid lines indicate the regression fits of the observations (Table 2). The predictions according to Concentration Addition (—) and Independent Action (– – –) are indicated, confidence limits of the predictions are given in Table 3. Horizontal lines indicate the 0 and 50% effect-levels (solid) and the 95% confidence limits of the controls (dotted)
Binary mixtures of TBT, Irgarol, and Sea-Nine and their toxicity to algal reproduction. The substances are mixed in the ratio of their individual EC50 values (Table 2). Dots show the mean of the observed toxicity with 95% confidence interval (A: n = 4–6; B: n = 5–6; C: n = 2–3); thin solid lines indicate the regression fits of the observations (Table 2). The predictions according to Concentration Addition (—) and Independent Action (– – –) are indicated, confidence limits of the predictions are given in table 3, Horizontal lines indicate the 0 and 50% effect-levels (solid) and the 95% confidence limits of the controls (dotted)
All deviations between observed and predicted effect concentrations were statistically significant for mixture effects higher than 50% when compared to CA, and also for most of the IA predictions (Table 3). Thus, effects and effect concentrations for these effect levels were overestimated by both concepts, a situation that can unambiguously be classified as an antagonism. However, for mixture effect levels below 20%, the opposite was observed: mixture toxicity was underestimated by both concepts. Yet, the synergistic deviations were statistically significant in the algal reproduction test only (Table 3). The ternary mixture in the periphyton photosynthesis test did not show a statistically significant synergistic behaviour.
Two Component Mixtures
The algal reproduction assay was used to test all binary combinations of the three antifoulants. The objective was to narrow down the cause of the deviation between observed and predicted mixture toxicity to a possible binary interaction. Similar patterns of higher observed than predicted toxicity, as in the ternary mixture testing, were found at low effect levels whenever TBT was present in the mixtures (Fig. 4A and B). (However, these deviations were not statistically significant (Table 3). In contrast, the observed mixture toxicity at higher effect levels was clearly lower than predicted whenever Sea-Nine was present in the mixtures (Fig. 4B and C). These deviations were statistically significant for both concepts. The toxicity of the binary mixture with TBT and Irgarol was rather well predicted by IA at effect levels above 40% (within a factor 1.3), while CA overestimated the toxicity significantly (a factor 1.8).
Discussion
Single Substance Toxicity
All three antifoulants—TBT, Irgarol, and Sea-Nine—show high toxicity to both periphyton community photosynthesis and to the reproduction of the green algae Scenedesmus vacuolatus. Irgarol caused the highest toxicity in both biotests with EC50 values of 4 nM for periphyton photosynthesis and 22 nM for algal reproduction. These EC50 values are comparable with results from previous short-term studies on microalgal communities: about 5 nM for marine periphyton (Dahl and Blanck 1996b; Berard et al. 2003), 0.4 to 3.3 nM for freshwater periphyton (Berard et al. 2003), and between 2 and 20 nM for different single algal species (Berard et al. 2003).
The toxicity of TBT to periphyton photosynthesis (EC50 = 59 nM) was within the range previously reported with EC50 values ranging from 23 nM (Dahl and Blanck 1996a) to 99 nM (Blanck and Dahl 1996). The algal reproduction test was about 3 to 4 times less sensitive to TBT than the periphyton photosynthesis test with an observed EC50 of 215 nM.
The opposite pattern in sensitivity was observed for Sea-Nine where algal reproduction was more sensitive (EC50 = 314 nM) than periphyton photosynthesis (EC50 = 549 nM). The reason for the lower Sea-Nine toxicity to periphyton photosynthesis as compared to algal reproduction might be the mismatch between the test parameter photosynthesis and the proposed mechanism of action for Sea-Nine. Sea-Nine reacts with intracellular thiols, which participate in the catalytic functions of enzymes followed by generation of free radicals (Diehl et al. 1999), and such thiol-interactive enzyme inhibitors are typically slow acting with increasing effects up to 60 min and beyond (Collier et al. 1991). The shorter time of incubation within the photosynthesis test (45 min) might thus be responsible for the lower toxicity observed in relation to the algal reproduction test that was run for 24 h. Recent results (Göransson, unpublished data) indicate that prolonging exposure beyond 45 min would increase observed Sea-Nine toxicity to periphyton photosynthesis.
Irgarol has been found in natural waters up to 12 to 17 nM (Basheer et al. 2002). Hence, in our periphyton test, these concentrations would affect photosynthesis severely (92% reduction in photosynthetic activity). Reported environmental concentrations of TBT (10 nM; Basheer et al. 2002) and Sea-Nine (10 nM; Martinez et al. 2001) would only give negligible effects on periphyton photosynthesis if applied singly in our tests. However, effects on periphyton community structure and function in long-term experiments (two to three weeks) have been observed at considerably lower concentrations for all three antifoulants compared to short-term effects on periphyton photosynthesis. NOECs were estimated to be 0.5 nM for TBT (Dahl and Blanck 1996a), 0.25 nM for Irgarol (Dahl and Blanck 1996b), and 0.07 nM for Sea-Nine (Arrhenius, unpublished data). Yet, in the present report we have focused only on the acute, short-term effects on periphyton community photosynthesis and algal reproduction (S. vacuolatus).
Mixture Toxicity
The different biochemical mechanism of action of TBT, Irgarol, and Sea-Nine suggested IA to be the concept of choice. CA was comparatively evaluated as a reasonable worst-case approach only. However, both IA and CA failed to accurately predict the mixture toxicity of the three antifoulants over the analysed effect range (Fig. 3). Previous single-species studies have shown excellent IA predictivity with multi-component mixtures of other dissimilar-acting compounds. Both the curve shapes and the EC50s were accurately predicted and the deviation at the 50% effect level was within 1.1 only (Backhaus et al. 2000a; Faust et al. 2003). The predictive power of IA was clearly lower for our three-component antifouling mixture: the curve shapes were distinctly different although the deviations at the 50% effect level were less than a factor 1.6. At low effect levels, there were tendencies to underestimation of toxicity (synergism) and at higher effect levels there were clear overestimations of toxicity (antagonism). These phenomena were observed in the results of both test systems, periphyton community photosynthesis and algal reproduction, respectively. However, the differences between observed and predicted low effect concentrations were statistically significant only in the algal reproduction test for effects around 10%. For periphyton, the deviations were minor and could not be detected as statistically significant. Note that the higher data variability prevents a mean estimation of similar precision and thus only relative big differences between EC mean values can be detected as significant. However, antagonistic deviations observed at higher effect levels (>40%) were significant for both test systems.
Similar synergo-antagonistic patterns were observed in the binary mixture experiments. These phenomenona could be coupled to the presence of TBT and Sea-Nine, respectively. The tendencies to underestimate low mixture effects were only observed when TBT was present in the mixture, although these deviations were not statistically significant in any of the binary experiments. Moreover, Sea-Nine seemed to be a possible cause for the antagonistic effects observed at higher effect levels, as the overestimations of toxicity were clearly observed and statistically significant only in mixtures containing Sea-Nine.
The synergo-antagonistic phenomenona observed in the ternary and binary mixtures might be due to some kind of interactions between the mixture components in the solutions or in the biota. This would imply that the application of IA is not entirely correct for the tested antifoulant mixture because the concept assume a non-interactive type of joint action (Plackett and Hewlett 1952). Interactions whereby one chemical affects the toxicity of others are possible due to not only toxicant/target interactions but also to influences on adsorption, distribution and excretion, biotransformation and bioavailability (Altenburger et al. 2003). The precise molecular mechanisms of these interactions are so far unknown to us. Possible interactions caused by TBT and Sea-Nine might be coupled to the reaction with sulfhydryl-groups that seems to be a mutual property of these substances (Fent 1996; Diehl et al. 1999). The proposed cascade-like effect by Sea-Nine in reacting with thiol groups and the generation of free radicals (Diehl et al. 1999) might also be responsible for interactions. However, this requires further clarification.
Both algal assays give the same picture with clear overestimations in the upper effect region and underestimations in the lower region. This highlights the necessity to consider different effect levels during an assessment of the predictive capabilities of the concepts as a good agreement between prediction and observation for a certain effect does not necessarily allow a generalisation to other effect levels.
In the present periphyton studies, CA predicted slightly higher toxicity than IA, which is in compliance with most published studies (Backhaus et al. 2000a, b; Faust et al. 2001, 2003, Junghans et al. 2003, Altenburger et al. 2004, Arrhenius et al. 2004). CA overestimated the observed mixture toxicity by a factor within 2.5 at the level of 50% effect; at higher effect levels the overestimation was more distinct. In contrast, at low effect levels (effects <10%) there were indications that even CA underestimated the observed toxicity, but these effects were within the control variability and as such only barely visible in Figure 3. For the algal reproduction data, however, it is more evident that CA might not be protective enough as a worst-case estimation, especially for mixture effects lower than analysed in this study. At effect levels below 25%, we observe synergistic effects that are indeed significant at 10% effect and below. The nature of this synergism is not known.
Implications for Hazard and Risk Assessment of Antifouling Agents
Our study confirms the well-established fact that the tested antifoulants are ecotoxicologically important as single compounds, but also that the observed mixture toxicities are higher than the observed single substance toxicities. For example, the EC10 of Sea-Nine if applied singly was 130 nM in the periphyton assay. When 14 nM TBT and 1 nM Irgarol were added (corresponding to the molar EC50 mixture ratio), a total effect of 20% was observed. It has to be emphasized here that neither 14 nM TBT nor 1 nM Irgarol provoke clear individual effects, but still they double the mixture effect. This demonstrates that any ecologically realistic hazard assessment should take mixture effects into account, as assessing effects individually would underestimate the overall hazard of the mixture.
The differences between the IA-predicted and CA-predicted EC50 values are statistically significant in all experiments as judged by the non-overlapping confidence limits (Table 3). These narrow confidence limits are a reflection of the high precision of the experimental data and the associated predictions. In absolute terms, the differences between the predictions are quite small and do not even reach a factor of 2 in any assay or effect level. This is in concordance with the work by Faust (1999) in which it was mathematically proven that the difference between CA and IA for a three-component mixture is always equal or smaller than 3. Hence, the mechanism-based selection between CA (for mixtures of similarly acting substances) and IA (for mixtures of dissimilarly acting substances) might not be overly important for the investigated mixtures from a pragmatic hazard assessment perspective. Nevertheless, it has to be pointed out here that there are fundamental differences of both concepts when it comes to the contribution of low-effect concentrations to the expected mixture toxicity (for further details, see Faust 2001).
Although CA assumes a similar mechanism of action of all mixture components, it has been discussed as a general default assumption for assessing the toxicity of mixtures (Boedeker et al. 1993, Backhaus et al. 2000a, Faust et al. 2000). Whether this is generally acceptable, even for mixtures of non-similarly acting substances, depends on the danger of underestimating the actual hazard and on the quantitative error that might occur. In the present periphyton study, CA is slightly overprotective for most effect levels, e.g., by a factor of 2.5 at the EC50 level. Hence, these results are in concordance with the suggestion to use CA as a pragmatic default assumption of mixture toxicity assessments. This seems to hold true even on a community level of biological complexity and when dealing with a mixture of dissimilarly acting substances.
However, it has to be emphasized here that the results show that CA is only protective in a certain effect range (>20%). In the region of low effect concentrations, we observed tendencies to weak synergistic effects, i.e., higher toxicities than expected by CA or IA. The ratio between predicted and observed EC10 values was highest in the ternary mixture study with the algal reproduction assay (2.4 for CA and 3.7 for IA). In all other cases, the ratio between predicted and observed EC10 did not exceed a factor of 3. On the level of the EC50, differences between observed and predicted toxicities were even smaller. Whether any of these deviations is considered important (besides the pure statistical significance), depends on the actual context of an assessment. From a scientific point of view, the lower accuracy of the concepts for our antifouling mixtures as compared to other mixture studies (Altenburger et al. 2000; Backhaus et al. 2000a; b, Faust et al. 2003; Junghans et al. 2003; Altenburger et al. 2004) clearly needs further investigation.
From an environmental risk-assessment perspective, especially the more than concentration-additive effects in the low-effect region warrant further attention. The deviations between predicted and observed toxicities are likely due to some kind of interactions. However, it should be noted that photosynthesis might not be the most relevant short-term effect parameter for the tested substances due to their different mechanisms of action; only Irgarol affects photosynthesis primarily. Hence, other processes than photosynthesis might be more sensitive. An environmental risk assessment based on these data can, therefore, only be considered as a first step. Next it should be explored whether the observed patterns of mixture effects are found also in an ecologically more realistic context. This could be investigated, e.g., by long-term studies at the community level using more integrating parameters such as changes in species composition.
Future studies should also aim at clarifying the mechanistic basis of the observed phenomenona, especially at estimating the maximum expectable deviation from CA and the conditions (e.g., concentrations and mixture ratios) under which this is expected to occur. Such knowledge would then hopefully allow devising strategies such as the application of an additional safety factor to the CA-predicted mixture toxicity to derive sufficiently safe mixture toxicity estimates.
From their use pattern, it is to be expected that antifoulants occur as mixtures in the marine environment. Unfortunately, the actual exposure situation is unclear in that respect. Specific monitoring data that take into account the variety of different antifouling agents are sorely missing, especially outside harbor regions in which high concentrations of even the single substances are to be expected. It should also be noted that at present there seem to be no legal requirements to assess mixture toxicity of antifouling compounds. However, from a growing body of evidence it is clear that the application of any concept that accounts for possible mixture effects is more realistic than the present chemical-by-chemical assessment. Hence, environmental assessment of hazard and risk of antifoulants should be made with this in mind.
References
Altenburger R, Backhaus T, Boedeker W, Faust M, Scholze M, Grimme LH (2000) Predictability of the toxicity of multiple chemical mixtures to Vibrio fischeri: Mixtures composed of similarly acting chemicals. Environ Toxicol Chem 19(9):2341–2347
Altenburger R, Nendza M, Schuurmann G (2003) Mixture toxicity and its modeling by quantitative structure-activity relationships. Environ Toxicol Chem 22(8):1900–1915
Altenburger R, Walter H, Grote M (2004) What contributes to the combined effect of a complex mixture? Environ Sci Technol 38(23):6353–6362
Arrhenius Å, Grönvall F, Scholze M, Backhaus T, Blanck H (2004) Predictability of the mixture toxicity of 12 similarly acting congeneric inhibitors of photosystem II in marine periphyton and epipsammon communities. Aquat Toxicol 68(4):351–367
Backhaus T, Altenburger R, Boedeker W, Faust M, Scholze M, Grimme LH (2000a) Predictability of the toxicity of a multiple mixture of dissimilarly acting chemicals to Vibrio fischeri. Environ Toxicol Chem 19(9):2348–2356
Backhaus T, Scholze M, Grimme LH (2000b) The single substance and mixture toxicity of quinolones to the bioluminescent bacterium Vibrio fischeri. Aquat Toxicol 49:49–61
Backhaus T, Arrhenius Å, Blanck H (2004) Toxicity of a mixture of dissimilarly acting substances to natural algal communities: Predictive power and limitations of independent action and concentration addition. Environ Sci Technol 38(23):6363–6370
Basheer C, Tan KS, Lee HK (2002) Organotin and Irgarol-1051 contamination in Singapore coastal waters. Marine Pollut Bull 44(7):697–703
Berard A, Dorigo U, Mercier I, Becker-van Slooten K, Grandjean D, Leboulanger C (2003) Comparison of the ecotoxicological impact of the triazines Irgarol 1051 and atrazine on microalgal cultures and natural microalgal communities in Lake Geneva. Chemosphere 53(8):935–944
Biselli S, Bester K, Huhnerfuss H, Fent K (2000) Concentrations of the antifouling compound Irgarol 1051 and organotins in water and sediments of German North and Baltic Sea marinas. Marine Pollut Bull 40(3):233–243
Blanck H, Dahl B (1996) Pollution-induced community tolerance (PICT) in in marine periphyton in a gradient of tri-n-butyltin (TBT) contamination. Aquat Toxicol 35(1):59–77
Blanck H, Wängberg S-Å (1988) Validity of an ecotoxicological test system: short-term and long-term effects of arsenate on marine periphyton communities in laboratory systems. Can J Fish Aquat Sci 10:1807–1815
Bliss CI (1939) The toxicity of poisons applied jointly. Ann J Appl Biol 585–615
Boedeker W, Drescher K, Altenburger R, Faust M, Grimme LH (1993) Combined effects of toxicants: the need and soundness of assessment approaches in ecotoxicology. Sci Total Environ (Suppl) 931–938
Callow ME, Finlay JA (1995) A simple method to evaluate the potential for degradation of antifouling biocides. Biofouling 9(2):153–165
Callow ME, Willingham L, Shade WD, Hurt SS, Jacobson AH, Reinert K (1996) Degradation of antifouling biocides. Biofouling (1-3):239–249
Collier PJ, A R, Waigh RD, Douglas KT, Austin P, Gilbert P (1990a) Chemical reactivity of some isothiazolone biocides. J Appl Bacteriol 69:578–584
Collier PJ, Ramsey AJ, Austin P, Gilbert P (1990b) Growth inhibitory and biocidal activity of some isothiazolone biocides. J Appl Bacteriol 69:569–577
Collier PJ, Austin P, Gilbert P (1991) Isothiazolone biocides: enzyme-inhibition pro-drugs. Int J Pharmaceut 74:195–201
Dahl B, Blanck H (1996a) Pollution-induced community tolerance (PICT) in periphyton communities established under tri-n-butyltin (TBT) stress in marine microcosms. Aquat Toxicol 34:305–325
Dahl B, Blanck H (1996b) Toxic effects of the antifouling agent Irgarol 1051 on periphyton communities in coastal water microcosms. Mar Pollut Bull 342–350
Diehl MA, Fearnside KB, S CJ (1999) Antifungal mechanism of dichloro-n-octyl isothiazolone. Conference of Society for Industrial Microbiology (SIM), Washington, DC
Dunnett C (1964) New tables for multiple comparisons with a control. Biometrics 20:482–491
Efron B, Tibshirani R (1993) An introduction to the bootstrap 1st ed. Chapman & Hall, London
Faust M (1999) Kombinationseffekte von Schadstoffen auf aquatische Organismen; Prüfung der Vorhersagbarkeit am Beispiel einzelliger Grünalgen. Bremen, University of Bremen
Faust M, Altenburger R, Backhaus T, Boedeker W, Scholze M, Grimme LH (2000) Predictive assessment of the aquatic toxicity of multiple chemical mixtures. J Environ Qual 29:1063–1068
Faust M, Altenburger R, Backhaus T, Blanck H, Boedeker W, Gramatica P, Hamer V, Scholze M, Vighi M, Grimme LH (2001) Predicting the joint algal toxicity of multi-component s-triazine mixture at low-effect concentrations of individual toxicants. Aquat Toxicol 56:13–32
Faust M, Altenburger R, Backhaus T, Blanck H, Boedeker W, Gramatica P, Hamer V, Scholze M, Vighi M, Grimme LH (2003) Joint algal toxicity of 16 dissimilarly acting chemicals is predictable by the concept of independent action. Aquat Toxicol 63(1):43–63
Fent K (1996) Ecotoxicology of organotin compounds. Crit Rev Toxicol 26(1):3–117
Greco WR, Unkelbach H-D, Pöch G, Suhnel J, Kundi M, Boedeker W (1992) Consensus on concepts and terminology for combined-action assessment: the Saariselkä agreement, Arch Complex Environ Studies 4(3):65–69
Grimme LH, Boardman NK (1972) Photochemical activities of a particle fraction P1 obtained from the green alga Chlorella fusca. Biochem Biophys Res Commun 49:1617–1623
Grönvall F, Barcelo D, Blanck H, Dahl B, Martinez K, Nihlén P, Peterson M (2001) Development of community tolerance to Irgarol 1051 in marine periphyton after years of coastal water contamination. Madrid, Spain, 11th Annual SETAC Meeting
Hall JLW, Giddings JM, Solomon KR, Balcomb RR (1999) An ecological risk assessment for the use of Irgarol 1051 as an algaecide for antifoulant paints. Crit Rev Toxicol 29(4):367–437
Hermens JLMea (1984) Quantitative structure-activity relationships and mixture toxicity studies of chloro- and alkylanilines at an acute lethal toxicity to the guppy (Poecilia reticulata). Ectoxicol Environ Safety 8:388–394
Hermens JLM, Leeuwangh P (1982) Joint toxicity of mixtures of 8 and 24 chemicals to the guppy (Poecilia reticulata). Ecotoxicol Environ Safety 6:302–310
Hermens JLM, Canton H, Janssen P, Jong R (1984) Quantitative structure-activity relationship and toxicity studies of mixtures with anaesthetic potency: Acute lethal and sublethal toxicity to Daphnia magna. Aquat Toxicol 5:143–154
IMO (2001) TBT ban convention adopted. IMO News 4
Junghans M, Backhaus T, Faust M, Scholze M, Grimme LH (2003) Predictability of combined effects of eight chloroacetanilide herbicides on algal reproduction. Pest Manage Sci 59(10):1101–1110
Konstantinou IK, Albanis TA (2004) Worldwide occurrence and effects of antifouling paint booster biocides in the aquatic environment: a review. Environ Int 30(2):235–248
Könemann H (1980) Structure-activity relationships and additivity in fish toxicities of Environmental pollutants. Ecotoxicol Environ Safety 4:415–421
Könemann H (1981) Fish toxicity tests with mixtures of more than two chemicals: a proposal for a quantitative approach and experimental results. Toxicology 19:229–238
Lambropolou DA, Sakkas VA, Albanis TA (2002) Headspace solid phase microextraction for the analysis of the new antifouling agents Irgarol 1051 and Sea Nine 211 in natural waters. Anal Chim Acta 468:171–180
Lamoree MH, Swart CP, van der Horst A, van Hattum B (2002) Determination of diuron and the antifouling paint biocide Irgarol 1051 in Dutch marinas and coastal waters. J Chromatogr A 970:183–190
Larsen DK, Wagner L, Gustavson K, Forbes VE, Lund T (2003) Long-term effect of Sea-Nine on natural coastal phytoplankton communities assessed by pollution induced community tolerance. Aquat Toxicol 62:35–44
Loewe S (1927) Die Mischarznei. Versuch einer allgemeinen Pharmakologie der Arzneikombinationen. Klin Wochenschr 6:1077–1085
Loewe S, Muischnek H (1926) Über Kombinationswirkungen. 1. Mitteilung: Hilfsmittel der Fragestellung. Nanyn-Schmiedebergs Arch Exp Pathol Pharmakol 114:313–326
Martinez K, Ferrer I, Barcelo D (2000) Part-per-trillion level determination of antifouling pesticides and their byproducts in seawater samples by off-line solid-phase extraxtion followed by high-performance liquid chromatography-atmospheric pressure chemical ionization mass spectrometry. J Chromatogr A 879:27–37
Martinez K, Ferrer I, Hernando MD, Fernandez-Alba AR, Marce RM, Borrul F, Barcelo D (2001) Occurence of antifouling biocides in the Spanish mediterranean marine environment. Environ Technol 22:543–552
Mezuca M, Hernando MD, Piedra L, Aguera A, Fernandez-Alba AR (2002) Chromatography-mass spectrometry and toxicity evaluation of selected contaminants in seawater. Chromatographia 56(3/4):199–206
Molander S, Dahl B, Blanck H, Jonson LA, Sjöström M (1992) Combined effects of Tri-n-butyl Tin (TBT) and diuron on marine periphyton communities detected as pollution-induced community tolerance. Arch Environ Contam Toxicol 22:419–427
Plackett RL, Hewlett PS (1952) Quantal responses to mixtures of poisons. J R Stat Soc B14:141–163
Plackett RL, Hewlett PS (1967) A comparison of two approaches to the construction of models for quantal responses to mixtures of drugs. Biometrics 23(1):27–44
Sakkas VA, Konstantinou IK, Lambropolou DA, Albanis TA (2002) Survey of the occurence of antifouling paint booster biocides in the aquatic environment of Greece. Environ Sci Pollut Res 9(5):327–332
Scholze M, Boedeker W, Faust M, Backhaus T, Altenburger R, Grimme LH (2001) A general best-fit method for concentration-response curves and the estimation of low-effect concentrations. Environ Toxicol Chem 20(2):448–457
Shade WD, Hurt SS, Jacobson AH, Reinert KH (1993) Eological risk assessment of a novel marine antifoulant. In: Gorsuch JW, Dwyer FJ, Ingersoll CG, La Point W (eds.), Environmental toxicology and risk assessment: American Society for Testing and Materials, Philadelphia 2 ASTM STP 1216:381–408
Thomas KV (2001) The environmental fate and behaviour of antifouling paint booster biocides: A review. Biofouling 17(1):73–86
Thomas KV, Blake SJ, Waldock MJ (2000) Antifouling paint booster biocide contamination in UK marine sediments. Marine Pollut Bull 40(9):739–745
Thomas KV, Fileman TW, Readman JW, Waldock MJ (2001) Antifouling pain booster biocides in the UK coastal environment and potential risks of biological effects. Marine Pollut Bull 42(8):677–688
Thomas KV, McHugh M, Hilton M, Waldock M (2003) Increased persistence of antifouling paint biocides when associated with paint particles. Environ Pollut 123(1):153–161
Yebra DM, Kiil S, Dam-Johansen K (2004) Antifouling technology: past, present and future steps towards efficient and environmentally friendly antifouling coatings. Prog Org Coat 50(2):75–104
Acknowledgments
The excellent technical assistance with the algal reproduction tests of Wiebke Meyer, Marianne Matzke, Friederike von Möller, and Erika Lorenz at the University of Bremen (Germany) is gratefully acknowledged. The excellent working facilities at the Kristineberg Marine Research Station and the corresponding funding from the Hierta-Retzius foundation and Göteborg University Marine Research Centre were crucial for these studies. Thanks to three anonymous reviewers for fruitful comments. The research was financially supported by the Commission of the European Union; PREDICT: Prediction and assessment of the aquatic toxicity of mixtures of chemicals (ENV4-CT96-0319); and BEAM: Bridging effect assessment of mixtures to ecosystem situation and regulations (EVK1-1999-00055).
Author information
Authors and Affiliations
Corresponding author
Rights and permissions
About this article
Cite this article
Arrhenius, Å., Backhaus, T., Grönvall, F. et al. Effects of Three Antifouling Agents on Algal Communities and Algal Reproduction: Mixture Toxicity Studies with TBT, Irgarol, and Sea-Nine. Arch Environ Contam Toxicol 50, 335–345 (2006). https://doi.org/10.1007/s00244-005-1057-9
Received:
Accepted:
Published:
Issue Date:
DOI: https://doi.org/10.1007/s00244-005-1057-9