Abstract
Polyphenols are known as inhibitors of glycation since they alter the pathways of the Maillard reaction by several mechanisms involving antioxidant activity, reactive dicarbonyl trapping, inhibition of sugar autoxidation, and amino group binding. Green tea is a rich source of polyphenols. This study aimed to investigate the effects of protein modification with green tea polyphenols on glycation potential of ovalbumin. For this purpose, green tea infusion was prepared and ovalbumin was treated with that infusion at alkaline pH conditions in order to let oxidation of polyphenols to quinone forms and formation of covalent linkages between quinones and amine residues of protein. This modified ovalbumin was heated with glucose at 90 °C. Furosine, the compound formed from N-ε-fructoselysine during acid hydrolysis, and N-ε-carboxymethyl lysine (CML) as indicators of early and advanced glycation, respectively, were monitored. Free lysine concentrations and antioxidant activity were measured in order to enlighten the interaction mechanism. Results showed that treatment of ovalbumin with green tea phenolics before reaction with glucose has a potential to limit glycation. In total 15 % less furosine was formed in treated ovalbumin than control ovalbumin upon heating at 90 °C for 1 h, whereas 14 % less CML was formed.
Similar content being viewed by others
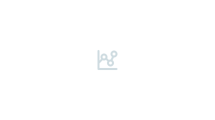
Explore related subjects
Discover the latest articles, news and stories from top researchers in related subjects.Avoid common mistakes on your manuscript.
Introduction
The Maillard reaction or non-enzymatic glycation takes place by the modification of proteins, in particular at lysine side chains, by glucose or other reducing sugars through a series of complex reactions. Firstly, the carbonyl group of reducing sugar and an unprotonated amine group of protein form a freely reversible Schiff base by nucleophilic addition reaction. This is subsequently stabilized after rearrangement into Amadori products or Heyns products according to the type of sugar involved (aldoses or ketoses) [1]. Through a series of oxidative and non oxidative reactions, early glycation products are formed [2]. Furosine, the compound that is formed during acid hydrolysis from N-ε-fructoselysine (FL), is a good marker of early glycation of proteins, especially rich in lysine. Further reactions (degradation, oxidation, cross-linkages and polymerization) lead to the formation of advanced glycation end products (AGEs), such as N-ε-carboxymethyl lysine (CML) [3–5]. Protein glycation in humans is believed to be implicated in the development of chronic degenerative diseases due to the modification of proteins with carbohydrate and lipid derived intermediates resulting in changes of the functional properties of proteins, lipids and DNA [6]. Glycation occurs also during food processing and leads to impairment of nutritional quality of food, generation of undesired compounds (hydroxymethylfurfural, acrylamide, etc.) and formation of AGEs. Dietary intake of AGEs may cause their accumulation in blood stream and in tissue proteins [7], then undergoing further reactions in the body [8]. For this reason, inhibition of glycation has come into prominence for both medical and food science investigations in recent years [9–12]. The inhibition of glycation might follow several mechanisms involving, aldose reductase, antioxidant activity, reactive dicarbonyl trapping, inhibition of sugar autoxidation and amino group binding [13, 14]. Natural antioxidants such as flavonoids and phenolic compounds have good potential as inhibitors of glycation since they alter the pathways of the Maillard reaction [15–18]. Most of studies have revealed that catechins are known to sequester the reactive dicarbonyl compounds, through electrophilic aromatic substitution reactions, thanks to the presence of two highly activating electron-donating groups in A ring of catechins [16, 18]. Recently, catechins have also been reported as trapping agents for the reactive imine intermediates linked to Maillard reaction [19]. Green tea is a rich source of catechins, such as epicatechin, epigallocatechin, epicatechin gallate, epigallocatechin gallate, and it was shown that green tea has anti-glycation effects on diabetic rats [20].
Although there are several studies about the anti-glycation activity of phenolic compounds, the covalent interaction between amino groups of protein and phenolic compounds at oxidation conditions before Maillard reaction has not been studied yet. In this study, how the treatment of proteins with oxidized green tea phenolic compounds before the Maillard reaction affects glycation of protein was investigated. For this purpose, ovalbumin was pretreated with green tea phenolic compounds at oxidation conditions (at pH 9.0, 50 °C for 1 h) to obtain insoluble ovalbumin-phenolic compounds complex. After glycation at 90 °C up to 1 h, the formation of early glycation compound, N-ε-fructoselysine, and advanced glycation end product, CML, were investigated in ovalbumin-glucose model system. Furthermore, antioxidant capacity of ovalbumin and the lysine concentration were determined after treatment in order to prove the linkages between green tea polyphenols and ovalbumin.
Materials and methods
Chemicals
All chemicals and solvents used were of analytical grade. Potassium peroxydisulfate, 2,2′-azinobis (3-ethylbenzothiazoline-6-sulfonic acid) (ABTS), 6-hydroxy-2,5,7,8 tetramethylchroman-2 carboxylic acid (Trolox), albumin from chicken egg white, glucose, lysine, CML standard, sodium borohydride (Fluka), HPLC grade hydrochloric acid and formic acid were purchased from Sigma-Aldrich Chemie (Steinheim, Germany). Disodium hydrogen phosphate, potassium dihydrogen phosphate, and boric acid were from Merck (Germany). Furosine standard was purchased from Neosystem Laboratoire (Strasbourg, France). Syringe filters (nylon, 0.45 µm), Oasis HLB solid phase extraction cartridges (1 mL, 30 mg), Atlantis T3 column (4.6 × 150 mm, 3 µm), Atlantis HILIC column (150 × 2.1 mm, 3 µm), and Atlantis HILIC column (250 × 4.6 mm, 5 µm) were supplied from Waters (Millford, MA). Amicon ultrafiltration cell (model 8200) and polyethersulfone ultrafiltration membrane (NMWL: 30,000 and Dia: 63.5) were purchased from Merck Millipore. Green tea leaves (brand of ÇAYKUR which is produced in Rize/Turkey) were purchased from a local market in Ankara, Turkey.
Preparation of ovalbumin-green tea infusion (OVA-GTI) complex
Green tea infusion (GTI) was used as the source of phenolic compounds comprising mostly catechins. After a total of 3 g tea was brewed in 100 mL of boiling water for 15 min, tea infusion was filtered through a coarse filter paper. Hundred milligrams of ovalbumin from chicken egg white in 10 mL water was mixed with 10 mL GTI and the pH of the mixture was adjusted to 9.0 with 0.1 M NaOH. The reaction tube was mixed using a magnetic stirrer at a speed of 350 rpm at 50 °C for 1 h. These reaction conditions (pH 9.0, 50°C, 1 h) were determined in our recent study as the optimum conditions for the reaction between soluble phenolic compounds and free amino groups [21]. Following the reaction, the OVA-GTI complex was separated from unbound green tea polyphenols by using ultrafiltration. The retentate was further washed with water to remove the remaining traces of unbound soluble fractions. The antioxidant capacity of the water used in the last step was measured in order to be sure that final precipitate was free of soluble antioxidant compounds. Thereafter, final insoluble OVA-GTI complex was lyophilized for further use. As a control, ovalbumin solution was prepared at pH 9 under the same conditions, and lyophilized.
Glycation reaction
A portion of 25 mg lyophilized OVA-GTI complex and control ovalbumin (treated with buffer solution only) were weighed into glass reaction tubes. In total 100 µmol glucose in 1 mL of water was added into tubes and allowed samples to dissolve. After proper mixing, the content of tubes was lyophilized and heated in a water bath maintained at 90 °C for different times up to 1 h. Two sets of reaction were performed at the same conditions for furosine and lysine and for CML analysis. After glycation, a set of the samples were incubated at 37 °C for 7 days in order to see the effect of storage on progression of glycation.
Furosine analysis
Analysis of furosine was performed according to Gökmen et al. [22]. 5 mL of hydrochloric acid (8 N) was added onto the contents of the tubes. The tubes were flushed with nitrogen and tightly closed. They were hydrolyzed for 23 h at 110 °C. The hydrolysates were filtered through filter paper, and then 100 µL were put into a glass vial. It was evaporated to dryness under a gentle stream of nitrogen at 40 °C. Then, the residue was redissolved in 1 mL deionized water, and passed through OASIS HLB cartridges. 10 µL was injected into HPLC system. An Agilent Technologies (Waldbronn, Germany) 1200 HPLC system consisting of a quaternary pump, a degasser unit, photodiode array detector and a temperature- controlled column oven was used. Chromatographic separation was performed on Atlantis HILIC column (250 × 4.6 mm, 5 µm) at 40 °C using a mobile phase of 1 % formic acid at a flow rate of 1 mL/min. Data acquisition was performed acquiring chromatograms at the detection wavelength of 280 nm. Quantification was based on calibration curve prepared using calibration solutions of furosine (1–10 mg/L).
CML analysis
Analysis of CML was performed according to Palermo et al. [23] with some modifications as described elsewhere [24]. The content of reaction tube was mixed with 100 µL of deionized water. A total of 450 µL of sodium borate buffer (0.2 M, pH 9.2) and 500 µL of sodium borohydride (1 M solution prepared in 0.1 M NaOH) were added. They were incubated at room temperature for 4 h in order to reduce fructoselysine into hexitol lysine. Afterward 2 mL hydrochloric acid (8 N) was added and the tubes were closed under nitrogen flush. They were hydrolyzed for 24 h at 110 °C. In total 20 µL of hydrolysate was evaporated to dryness under nitrogen flush. After the content was washed with 1 mL deionized water, it was passed through a preconditioned Oasis HLB cartridge for cleanup. The first 8 drops of the eluent were discarded and rest was collected and diluted properly prior to LC–MS/MS analysis. Sample was injected into Atlantis T3 column (4.6 × 150 mm, 3 µm) at 40 °C coupled to Waters TQD LC–MS/MS system operated in positive ionization mode using the following interface parameters: Source temperature of 120 °C, desolvation temperature of 450 °C, collision gas flow of 0.20 mL/min, desolvation gas flow of 900 L/min, capillary voltage of 3 kV, cone voltage of 25 V, and extractor voltage of 2 V. Chromatographic separation was performed by using a mobile phase consisting of 0.1 % formic acid in water: 0.1 % formic acid in acetonitrile (90:10, v/v) at a flow rate of 0.50 mL/min. Acquisition was performed by monitoring m/z ratio of 205.10 for CML, 84.10 and 130.10 for its product ions. Quantification was performed by means of a matrix-matched calibration curve. Hydrolysate of unheated protein was used as blank matrix. Calibration solutions of CML were prepared in the blank matrix at concentrations of 0, 1, 2.5, 5.0, and 10 µg/mL. Then, they were subjected to the lengthy extraction procedure that was used for actual samples, and analyzed as described above.
Lysine analysis
Lysine analysis was performed according to the method published elsewhere [25] with slight modifications. In total, 100 µL of the hydrolysate was put into a glass vial. It was evaporated to dryness under a gentle stream of nitrogen at 40 °C. The residue was redissolved in 1 mL of deionized water. After passing through a 0.45-µm syringe filter, the filtrate was diluted 10 times with the mixture of acetonitrile:water (1:1, v/v). In total, 10 µL was injected into LC–MS/MS system. Chromatographic separation was performed on Atlantis HILIC column (150 × 2.1 mm, 3 µm) at 40 °C using a gradient mixture of 0.1 % formic acid in water (A) and 0.1 % formic acid in acetonitrile (B) at flow rate of 0.4 mL/min. The eluent composition starting with 15 % of A linearly increased to 40 % in 4 min and held for 3 min. Then, it was linearly decreased to its initial conditions (15 % of A) in 1 min. Doing so, the total chromatographic run was completed in 8 min. Quantification was performed by means of external calibration curves built for lysine in a range between 0.1 and 2.0 mg/L. Waters TQD LC–MS/MS system was operated in positive ionization mode using the following interface parameters: source temperature of 120 °C, desolvation temperature of 350 °C, collision energy 12 V, desolvation gas flow of 900 L/h, capillary voltage of 3.5 kV, cone voltage of 20 V, and extractor voltage of 3 V. Data acquisition was performed by monitoring m/z ratio of 147.0 for lysine and 101.0 and 130.0 for its product ions.
Determination of total antioxidant capacity of protein
The antioxidant capacity of treated or untreated ovalbumin was measured by direct QUENCHER procedure using ABTS·+ radical solution [26]. Antioxidant capacity was measured according to the procedure described elsewhere [27]. Ten milligrams of insoluble fraction was transferred into a test tube and the reaction was started by adding 10 mL of ABTS·+ working solution. Following the vigorous shake in an orbital shaker (at 350 rpm for 27 min) in the dark, the tube was centrifuged at 6080×g for 2 min. After a total of 30-min reaction time, the optically clear supernatant (2 mL) was transferred into a cuvette, and absorbance measurement was performed at 734 nm using a Shimadzu model 2100 variable wavelength UV−visible spectrophotometer (Shimadzu Corp., Kyoto, Japan). If the values of absorbance were below the linear response range of the radical discoloration/color formation due to the high antioxidant activity, preliminary dilution was necessary. Dilution was performed by mixing the ground samples with cellulose, an inert material against the radical solution, at different ratios (1:1, 1:10, 1:100 (w:w) etc.) in a tube depending on the total antioxidant capacity values. Trolox was used as a standard reference to convert the inhibition percentage of each sample to the Trolox equivalent antioxidant capacity (TEAC). The standard solutions of Trolox were prepared in methanol at a concentration range between 0 and 600 µg/mL.
Statistical analysis
The analytical data were reported as the mean ± standard deviation of duplicate independent measurements and were subjected to one-way ANOVA. The significance of mean differences was determined by Tukey HSD post hoc test using SPSS version 17.0. p values of <0.05 were considered statistically significant.
Results and discussion
The total antioxidant capacity of ovalbumin was determined as 85.81 ± 3.34 mmol TE/kg, and it reached only to 101.60 ± 4.28 mmol TE/kg after alkaline treatment for control model system (pH 9.0, 50 °C, 1 h). This slight increase in antioxidant capacity of ovalbumin might be the result of high temperature and alkaline hydrolysis, which causes revealing of the amino acid residues that are previously buried in the structure of protein. Antioxidant property of proteins are first related to their amino acid composition and secondly to their positioning. Localization and physical accessibility of amino acids having antioxidant action is therefore important [28]. Protein structure, its molecular weight, and amino acid sequences may strongly affect its antioxidant capacity. Medina-Navarro et al. [29] emphasized that the structural molecular integrity is the most important issue for the antioxidant action of proteins. Any change that alters the accessibility of amino acid residues may increase antioxidant activity. In a study conducted with different proteins [28], a short period of protein heating was shown to be a very efficient factor that affects the partial denaturation, unfolding of protein chains and liberation of reactive residues, as well as, hydrogen and disulfide bonds from the structure of a protein, promoting reactions of these with free radicals and its transfer. Therefore, the increase after alkaline treatment of ovalbumin might have resulted in increased antioxidant activity due to partial denaturation. However, when ovalbumin was treated with green tea infusion, the antioxidant capacity of ovalbumin reached to 538.93 ± 68.76 mmol TE/kg due to the binding of green tea phenolic compounds to the ovalbumin structure. In previous studies conducted by our group, it was shown that green tea polyphenols caused a significant improvement in the antioxidant activity of wheat bran [21, 30]. During the reaction between wheat bran and green tea polyphenols, increasing the pH of the reaction medium from 2.0 to 9.0 linearly increased the antioxidant capacity [21]. Polyphenols were first oxidized to quinone forms under alkaline conditions following by binding to free amino groups available on the surface of wheat bran matrix, and then, soluble phenolic compounds continue to polymerize at the surface of wheat bran [21]. Similarly, in the present study, oxidized phenolic compounds, mostly catechins, could readily undergo attack by nucleophiles such as lysine, methionine, cysteine and tryptophan moieties in ovalbumin (Fig. 1), which causes increase in the antioxidant capacity. Catechins consist of the 20–30 % of the dry weight of green tea, and comprise most of the phenolic compounds of green tea infusion [31]. The binding of oxidized phenolics also may lead to an impairment of available glycation sites of ovalbumin, which might cause decrease in furosine and CML formation during heating with glucose.
Furosine, the compound derived from acidic hydrolysis of N-ε-fructoselysine (FL), is a good marker of early glycation of proteins, especially rich in lysine. Figure 2 shows furosine formation in ovalbumin-glucose model system during heating at 90 °C for 1 h. In both control ovalbumin-glucose (OVA-G) and treated ovalbumin-glucose (OVA-GTI-G) systems, the amount of furosine increased gradually until a plateau value. In control OVA-G system, the plateau was reached in 10 min, while it took 20 min for OVA-GTI-G system. The initial formation rate of furosine in control OVA-G system was 0.057 mol/mol lysine.min while that of OVA-GTI-G was 0.043 mol/mol lysine.min, which corresponds to a 24 % reduction. The amount of furosine formed in OVA-GTI-G system is much lower than control ovalbumin-glucose system. In OVA-GTI-G system, there was about 15 % reduction in furosine formation compared to control OVA-G system at the end of heating for 1 h. This revealed that treatment ovalbumin with green tea phenolic compounds before heat treatment has a potential to limit the formation of early glycation products during heating.
There are several studies showing anti-glycation effects of polyphenols [18, 32–35]. The effect was mostly attributed to their carbonyl trapping activity of phenols [18, 34]. Antioxidant property is the other possible anti-glycation mechanism, which leads to impairment in the oxidation reaction; however, this effect is particularly important for advanced stages of glycation [36]. Another mechanism, autoxidation of phenols, leads quinone formation, which further reacts with amine residues of the protein molecule, yielding a decreased glycation. Yin et al. [35] reported that high concentration of epicatechin inhibited formation of intermediary radicals during the Maillard reaction that takes place during heating glucose and lysine [35]. It was explained that epicatechin quinone forms reacted with lysine during Maillard reaction. Guerra & Yaylayan [33] also showed that reaction of glycine and oxidized form of catechin leads to the formation of single- and double-addition products and interfere with the Maillard reaction in food through scavenging of amino acids [33]. This reaction of polyphenols is favorable at alkaline conditions and in oxidizing environment. When ovalbumin molecule was reacted with green tea extract at pH 9.0, oxidized form of polyphenols might have reacted with lysine residues of protein, leading to decrease in furosine formation. Rawel et al. [37] also showed that lysozyme was modified by covalent attachment of phenolic compounds at pH 9.0 which is favorable pH conditions in which polyphenols may readily oxidize to respective quinones [37]. Similarly, in our previous study [21], pH 9.0 was found to be the most favorable pH for the binding of green tea polyphenols onto the free amino groups of wheat bran. Silvan et al. [38, 39] presented similar results with ferulic acid and soy isoflavones. Incubation of soy glycine or bovine serum albumin with ferulic acid at 60 °C for 60 min at pH 12 before reaction with fructose was found to reduce fluorescent AGEs and CML formation by nearly 90 and 85 %, respectively [38]. It was stated that ferulic acid might form complexes with proteins providing unusual protective effects toward the oxidation of protein [38]. By using very similar model system preparation technique (at 60 °C for 1 or 16 h at pH 12), they also showed that soy isoflavone-rich extract (composed of a mixture of soy isoflavones) significantly decreased the formation of early Maillard reaction products and also the progress of the reaction to the advanced stage [39]. It was suggested that the formation of early Maillard reaction products may be inhibited by conjugation of isoflavones to the active site of glycation, while AGEs formation may be modulated by trapping of dicarbonyl intermediates and oxygen radical species [39].
The change in free lysine concentration of OVA-G model system upon glycation is shown in Fig. 3. There was a 12 % reduction in the free lysine residues in ovalbumin molecule after treatment with green tea infusion, before reaction with glucose (p < 0.05). This decrease in available lysine residues caused a diminished glycation potential for ovalbumin. Upon heating at 90 °C for 1 h, 63 % of the lysine was modified by glycation in control OVA-G system, whereas it was restricted to 53 % modification in lysine in OVA-GTI-G system (p < 0.05). Therefore, it can be said that treatment of ovalbumin with oxidized phenolics may lead to protection against glycation of lysine residues.
Figure 4 shows CML formation during the reaction between ovalbumin or treated ovalbumin and glucose at 90 °C for 1 h. Treatment with green tea infusion slightly decreased CML formation comparing to control, however this decrease was not statistically significant (p > 0.05) for certain heating times (Fig. 4). Then, 14 % less CML was formed in OVA-GTI-G model system than control (OVA-G) model system after heating for 1 h (p > 0.05).
CML is an advanced glycation end product and it can be formed through two pathways (Fig. 5). The early glycation product N-ε-fructoselysine could undergo oxidation to form CML, or intermediate products of glucose (glyoxal) could bind directly to lysine further forming CML. Therefore, it is clear that the restricted amount of N-ε-fructoselysine resulted in decreased CML formation. The 15 % reduction in furosine formation correlates well with the 14 % reduction in CML formation during 1 h of heating of the OVA-GTI-G model system. This result also brings us to the point that the predominant CML formation route is through the oxidation of furosine, not through the direct binding of glyoxal to lysine in our experimental conditions. Similarly, Akıllıoğlu & Gökmen [24] showed that complexation of casein with epicatechin before reaction with glucose resulted in decrease in CML formation. However, in that study, non-covalent interactions were focused on, epicatechin was complexed with casein by hydrophobic interactions. It was explained that, the decreased CML concentrations besides the undiminished furosine level indicated that epicatechin may have trapped glyoxal, and therefore had inhibited advanced glycation of casein [24].
Prolongation of Maillard reaction results in the accumulation of advanced glycation end products. Therefore, CML concentrations of model systems were also determined after storage of samples at 37 °C for 7 days. Storage of glycated samples resulted in increase in CML levels as expected; in both model systems approximately 0.2 mmol/mol lysine of CML was formed upon incubation during storage. CML levels reached from 0.50 to 0.74 mmol/mol lysine, and from 0.43 to 0.64 mmol/mol lysine in model systems of OVA-G and OVA-GTI-G, respectively, heated at 90 °C for 1 h. There could not be found statistically significant effect of complexation with green tea polyphenols on CML formation upon incubation, except the samples heated for 1 h (p < 0.05; Fig. 6). There are conflicting results about the effects of polyphenols on glycation; some researchers reported increased glycation [40, 41]. Nagai et al. [33] stated that epicatechin could both inhibit and enhance glycation [41]. It was explained by Fujiwara et al. [40] that catechol group containing polyphenols generate hydrogen peroxide during autoxidation of catechol structure, and then hydrogen peroxide may generate hydroxyl radicals, and finally CML is generated by the reaction of hydroxyl radicals and Amadori products [40]. In our study, although treatment of ovalbumin with green tea polyphenols before heat treatment with glucose caused a reduction in CML during heating, the effect was not profound for the prolongation of glycation during storage (p > 0.05).
The decrease in both CML and furosine concentrations during heating of ovalbumin complexed with green tea polyphenols proves that, green tea polyphenols have effect on the early stage of glycation. The effect of polyphenols on prolonged storage is not distinct. Therefore, it can be concluded that, CML formation takes place through N-ε-fructoselysine during heating. This route might be predominant in our experimental conditions, since green tea polyphenols prevent oxidation reactions that favor the other route (through direct binding of oxidation products of glucose to lysine). Fernandez-Gomez et al. [42] reported that isomerized and oxidized forms of chlorogenic acid might be able to bind to bovine serum albumin protein under mimicked physiological conditions and this binding leads to increase in antioxidant capacity of BSA and inhibit the formation of AGEs.
About 10 % of consumed dietary AGEs were shown to be absorbed by humans and be correlated with circulating and tissue levels of AGEs [43]. Studies in healthy people showed that dietary AGEs correlate with circulating AGE levels, such as CML and methylglyoxal, and with oxidative stress markers [44]. Furthermore, reduction in AGEs in the diet in diabetes patients [45] and kidney disease patients [46, 47] or healthy individuals [48] also reduced markers of oxidative stress and inflammation. The modification of protein molecule by covalent attachment of green tea polyphenols seems to have an advantage in terms of anti-glycation concept. The results revealed that quinone forms of green tea polyphenols might react with free amino groups of ovalbumin under alkaline conditions. Thereby, the concentration of glycation products, which occur during heating of ovalbumin and glucose, decreased due to the modified lysine moieties in ovalbumin. However, researches with advanced analytical tools should be performed for the identification of adducts formed during complexation of protein and oxidized phenolic compounds. Due to the fact that lysine is an essential amino acid, there are health concerns about the bioavailability of modified lysine residues in protein. Lysine modification might result in lower availability. Therefore there is a need to enlighten the consequences of modification of lysine residues with oxidized polyphenols, which is another important research topic.
References
Wu Q, Li S, Li X, Fu X, Sui Y, Guo T, Xie B, Sun Z (2014) A significant inhibitory effect on advanced glycation end product formation by catechin as the major metabolite of lotus seedpod oligomeric procyanidins. Nutrients 6:3230–3244
Kanska U, Boratynski J (2002) Thermal glycation of proteins by d-glucose and D-fructose. Arch Immunol Ther Exp (Warsz) 50:61–66
Hayase F, Shibuya T, Sato J, Yamamoto M (1996) Effects of oxygen and transition metals on the advanced Maillard reaction of proteins with glucose. Biosci Biotechnol Biochem 60:1820–1825
Miller AG, Hegge S, Uhlmann A, Gerrard JA (2005) A continuous enzyme assay and characterisation of fructosyl amine oxidase enzymes (EC 1.5.3). Arch Biochem Biophys 434:60–66
Yim HS, Kang SO, Hah YC, Chock PB, Yim MB (1995) Free radicals generated during the glycation reaction of amino acids by methylglyoxal. A model study of protein-cross-linked free radicals. J Biol Chem 270:28228–28233
Ames JM (2007) Dietary Maillard reaction products: implications for human health and disease. Czech J Food Sci 27:66–69
Baynes JW (2001) The role of AGEs in aging: causation or correlation. Exp Gerontol 36:1527–1537
Vlassara H (2005) Advanced glycation in health and disease role of the modern environment. Ann N Y Acad Sci 1043:452–460
Chompoo J, Upadhyay A, Kishimoto W, Makise T, Tawata S (2011) Advanced glycation end products inhibitors from Alpinia zerumbet rhizomes. Food Chem 129:709–715
Peng X, Cheng KW, Ma J, Chen B, Ho CT, Lo C, Chen F, Wang M (2008) Cinnamon bark proanthocyanidins as reactive carbonyl scavengers to prevent the formation of advanced glycation endproducts. J Agric Food Chem 56:1907–1911
Sun Z, Peng X, Liu J, Fan KW, Wang M, Chen F (2010) Inhibitory effects of microalgal extracts on the formation of advanced glycation endproducts (AGEs). Food Chem 120:261–267
Wang X, Zhang LS, Dong LL (2012) Inhibitory effect of polysaccharides from pumpkin on advanced glycation end-products formation and aldose reductase activity. Food Chem 130:821–825
Boušová I, Martin J, Jahodář L, Dušek J, Palička V, Dršata J (2005) Evaluation of in vitro effects of natural substances of plant origin using a model of protein glycoxidation. J Pharm Biomed Anal 37:957–962
Mesías M, Navarro M, Martínez-Saez N, Ullate M, del Castillo MD, Morales FJ (2014) Antiglycative and carbonyl trapping properties of the water soluble fraction of coffee silverskin. Food Res Int 62:1120–1126
Delgado-Andrade C (2016) Carboxymethyl-lysine: thirty years of investigation in the field of AGE formation. Food Funct 7:46–57
Noda Y, Peterson DG (2007) Structure-reactivity relationships of flavan-3-ols on product generation in aqueous glucose/glycine model systems. J Agric Food Chem 55:3686–3691
Totlani VM, Peterson DG (2005) Reactivity of epicatechin in aqueous glycine and glucose Maillard reaction models: quenching of C2, C3, and C4 sugar fragments. J Agric Food Chem 53:4130–4135
Totlani VM, Peterson DG (2007) Influence of epicatechin reactions on the mechanisms of Maillard product formation in low moisture model systems. J Agric Food Chem 55:414–420
Bin Q, Peterson DG, Elias RJ (2012) Influence of phenolic compounds on the mechanisms of pyrazinium radical generation in the Maillard reaction. J Agric Food Chem 60:5482–5490
Babu PV, Sabitha KE, Shyamaladevi CS (2008) Effect of green tea extract on advanced glycation and cross-linking of tail tendon collagen in streptozotocin induced diabetic rats. Food Chem Toxicol 46:280–285
Doğan E, Gökmen V (2015) Mechanism of the interaction between insoluble wheat bran and polyphenols leading to increased antioxidant capacity. Food Res Int 69:189–193
Gökmen V, Serpen A, Morales FJ (2009) Determination of furosine in thermally processed foods by hydrophilic interaction liquid chromatography. J AOAC Int 92:1460–1463
Palermo M, Fiore A, Fogliano V (2012) Okara promoted acrylamide and carboxymethyl-lysine formation in bakery products. J Agric Food Chem 60:10141–10146
Akıllıoğlu HG, Gökmen V (2014) Effects of hydrophobic and ionic interactions on glycation of casein during Maillard reaction. J Agric Food Chem 62:11289–11295
Kocadaglı T, Zilic S, Tas NG, Vancetovic J, Dodig D, Gökmen V (2016) Formation of α-dicarbonyl compounds in cookies made from wheat, hull-less barley and colored corn and its relation with phenolic compounds, free amino acids and sugars. Eur Food Res Technol 242:51–60
Serpen A, Gökmen V, Fogliano V (2012) Total antioxidant capacities of raw and cooked meats. Meat Sci 90:60–65
Celik EE, Gökmen V, Fogliano V (2013) Soluble antioxidant compounds regenerate the antioxidants bound to insoluble parts of foods. J Agric Food Chem 61:10329–10334
Zilic S, Akıllıoğlu G, Serpen A, Barac M, Gökmen V (2012) Effects of isolation, enzymatic hydrolysis, heating, hydratation and Maillard reaction on the antioxidant capacity of cereal and legume proteins. Food Res Int 49:1–6
Medina-Navarro R, Durán-Reyes G, Díaz-Flores M, Vilar-Rojas C (2010) Protein antioxidant response to the stress and the relationship between molecular structure and antioxidant function. PLoS ONE 5:1–11
Çelik EE, Gökmen V (2014) Investigation of the interaction between soluble antioxidants in green tea and insoluble dietary fiber bound antioxidants. Food Res Int 63C:266–270
Wang H, Provan GJ, Helliwell K (2000) Tea flavonoids: their functions, utilisation and analysis. Trend Food Sci Technol 11:152–160
Colahan-Sederstrom PM, Peterson DG (2005) Inhibition of key aroma compound generated during ultrahigh-temperature processing of bovine milk via epicatechin addition. J Agric Food Chem 53:398–402
Guerra PV, Yaylayan VA (2014) Interaction of flavanols with amino acids: postoxidative reactivity of the B-ring of catechin with glycine. J Agric Food Chem 62:3831–3836
Sang S, Shao X, Bai N, Lo CY, Yang CS, Ho CT (2007) Tea polyphenol (−)-epigallocatechin-3-gallate: a new trapping agent of reactive dicarbonyl species. Chem Res Toxicol 20:1862–1870
Yin J, Hedegaard RV, Skibsted LH, Andersen ML (2014) Epicatechin and epigallocatechin gallate inhibit formation of intermediary radicals during heating of lysine and glucose. Food Chem 146:48–55
Zhang X, Chen F, Wang M (2014) Antioxidant and antiglycation activity of selected dietary polyphenols in a cookie model. J Agric Food Chem 62:1643–1648
Rawel HM, Kroll J, Rohn S (2001) Reactions of phenolic substances with lysozyme-physicochemical characterisation and proteolytic digestion of the derivatives. Food Chem 72:59–71
Silván JM, Assar SH, Chou S, del Castillo MD, Ames JM (2011) Control of the Maillard reaction by ferulic acid. Food Chem 128:208–213
Silvan JM, Chou S, Ames JM, del Castillo MD (2014) Glycation is regulated by isoflavones. Food & Funct 5:2036–2042
Fujiwara Y, Kiyota N, Tsurushima K, Yoshitomi M, Mera K, Sakashita N, Takeya M, Ikeda T, Araki T, Nohara T, Nagai R (2011) Natural compounds containing a catechol group enhance the formation of N ε-(carboxymethyl) lysine of the Maillard reaction. Free Radic Biol Med 50:883–891
Nagai R, Shirakawa J, Ohno R, Moroishi N, Nagai M (2014) Inhibition of AGEs formation by natural products. Amino Acids 46:261–266
Fernandez-Gomez B, Ullate M, Picariello G, Ferranti P, Mesa MD, del Castillo MD (2015) New knowledge on the antiglycoxidative mechanism of chlorogenic acid. Food Funct 6:2081–2090
Koschinsky T, He CJ, Mitsuhashi T, Bucala R, Liu C, Buenting C, Heitmann K, Vlassara H (1997) Orally absorbed reactive glycation products (glycotoxins): an environmental risk factor in diabetic nephropathy. Proc Natl Acad Sci 94:6474–6479
Uribarri J, Cai W, Peppa M, Goodman S, Ferrucci L, Striker G, Vlassara H (2007) Circulating glycotoxins and dietary advanced glycation endproducts: two links to inflammatory response, oxidative stress, and aging. J Gerontol A Biol Sci Med Sci 62:427–433
Vlassara H, Cai W, Crandall J, Goldberg T, Oberstein R, Dardaine V, Peppa M, Rayfield EJ (2002) Inflammatory mediators are induced by dietary glycotoxins, a major risk factor for diabetic angiopathy. Proc Natl Acad Sci 99:15596–15601
Uribarri J, Peppa M, Cai W, Goldberg T, Lu M, He C, Vlassara H (2003) Restriction of dietary glycotoxins reduces excessive advanced glycation end products in renal failure patients. J Am Soc Nephrol 14:728–731
Uribarri J, Peppa M, Cai W, Goldberg T, Lu M, Baliga S, Vassalotti JA, Vlassara H (2003) Dietary glycotoxins correlate with circulating advanced glycation end product levels in renal failure patients. Am J Kidney Dis 42:532–538
Vlassara H, Cai W, Goodman S, Pyzik R, Yong A, Chen X, Zhu L, Neade T, Beeri M, Silverman JM, Ferrucci L, Tansman L, Striker GE, Uribarri J (2009) Protection against loss of innate defenses in adulthood by low advanced glycation end products (AGE) intake: role of the antiinflammatory AGE receptor-1. J Clin Endocrinol Metab 94:4483–4491
Author information
Authors and Affiliations
Corresponding author
Ethics declarations
Conflict of interest
None.
Compliance with ethics requirements
This article does not contain any studies with human or animal subjects.
Rights and permissions
About this article
Cite this article
Cömert, E.D., Akıllıoğlu, H.G. & Gökmen, V. Mitigation of ovalbumin glycation in vitro by its treatment with green tea polyphenols. Eur Food Res Technol 243, 11–19 (2017). https://doi.org/10.1007/s00217-016-2717-x
Received:
Revised:
Accepted:
Published:
Issue Date:
DOI: https://doi.org/10.1007/s00217-016-2717-x