Abstract
Nickel is an ubiquitous transition metal that is industrially applied in many forms, which inevitably leads to a high degree of occupational and environmental exposure. Over-exposure to nickel can produce a variety of adverse effects on human health, including allergy and lung and nasal cancers. In the present study, it is demonstrated, for the first time, that nickel [(Ni(II)] (as a nickel nitrate salt) at concentrations that may be attained in vivo, induces neutrophils’ apoptosis by the intrinsic pathway. The use of diphenyleneiodonium, a NADPH oxidase inhibitor, delayed Ni(II)-induced apoptosis, suggesting that NADPH oxidase-derived reactive oxygen species and subsequent signaling could contribute to this event. This is an important finding since increased apoptosis mediated by nickel may disrupt the physiological activities of neutrophils, with potential impact in its immunological and antimicrobial role.
Similar content being viewed by others
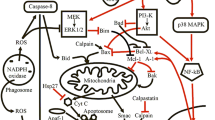
Avoid common mistakes on your manuscript.
Introduction
Nickel is an ubiquitous transition metal that is industrially applied in many forms, which inevitably leads to a high degree of occupational and environmental exposure (Barceloux 1999). Nickel can be absorbed via inhalation, ingestion and to a limited extent following dermal exposure (Chen et al. 2001; Krockova et al. 2011; Liden et al. 2008; Lu et al. 2005). This metal may be included in a group of micronutrients termed “possibly essential elements”, which include the elements that have been demonstrated as having beneficial effects in higher animals. These include conditionally essential, pharmacologically beneficial, and nutritionally beneficial. As reviewed by Forrest Nielsen (Nielsen 2007), recent animal experiments have shown that nickel is beneficial, if not essential, for optimal reproductive function, bone composition and strength, energy metabolism, and sensory function. This review also provides further evidence supporting the possible essentiality of nickel due to its requirement for some lower forms of life, where it participates in hydrolysis and redox enzyme reactions, regulates gene expression, and stabilizes certain structures. Estimates of the presumed human nickel requirement range within 5–50 μg/day (EMEA 2008). However, over-exposure to nickel can produce a variety of adverse effects on human health. Nickel allergy in the form of contact dermatitis is the most common reaction (Torres et al. 2009). Nevertheless, epidemiological studies of nickel compounds from occupationally exposed populations in the last few decades have also accumulated considerable evidence that exposure to both water-insoluble and water-soluble nickel is associated with lung and nasal cancers. In 1990, nickel was classified by International Agency for Research on Cancer (IARC), as carcinogenic for humans (nickel compounds Group 1, metal nickel group 2B) (Chen et al. 2003; Lu et al. 2005; Rana 2008).
A frequently discussed mechanism involving nickel adverse effects is the induction of oxidative damage of cellular constituents due to the generation of reactive oxygen species (ROS). Our group has shown that nickel, at concentrations that may be attained in vivo, induces the production of ROS by THP-1 monocytic cells (Freitas and Fernandes 2011) and human neutrophils (Freitas et al. 2010). Circulating neutrophils are recruited to sites of inflammation as a first line of defense against infectious agents or ‘‘nonself’’ substances that penetrate the body’s physical barriers (Babior 2000). The resolution of inflammation is associated with neutrophil clearance, which occurs upon apoptosis of neutrophils in situ and their subsequent engulfment by tissue macrophages. Human peripheral blood neutrophils have a short life span in vivo (t1/2 of about 7 h), while in vitro these cells undergo spontaneous apoptosis within 24 h of culture (Zhang et al. 2003). Importantly, an early apoptotic event may counteract the important physiological activities of neutrophils.
The ability of nickel [referred to hereafter as Ni(II)] to activate human neutrophils and then to induce its apoptosis could be one of the key properties of its carcinogenic mechanism, through regulating tumor cell proliferation, angiogenesis, and metastasis (Amulic et al. 2012). Importantly, evidence is emerging that these ROS are crucial in the execution of most neutrophil cell death mechanisms (Geering and Simon 2011). Since Ni(II) induces oxidative damage resulting from an increase of ROS production, it is possible that Ni(II)-induced ROS are involved in apoptosis. Nickel-induced apoptosis was first reported in Chinese hamster ovary (CHO) cells (Shiao et al. 1998). The phenomenon of apoptosis was also observed in other cell lines, for example, in CHO cells (Shiao et al. 1998), Leydig cells (Krockova et al. 2011), Jurkat T cells (Au et al. 2006), BEAS-2B cells, oral epithelium cells (Trombetta et al. 2005). However, there are no reports about the effect of Ni(II) on human neutrophils’ apoptosis. This prompted us to investigate the possible influence of Ni(II) in human neutrophils’ apoptosis, as well as the putative mechanisms involved.
Materials and methods
Materials
Diphenyleneiodonium (DPI), pepsatin, aprotinin, leupeptin, bovine serum albumin (BSA), phenylmethylsulphonyl fluoride (PMSF), soybean trypsin inhibitor (SBTI) were purchased from Sigma Chemical Co. (St. Louis, USA). Nickel nitrate was obtained from Fluka Chemie GmbH (Steinheim, Germany). Dulbecco’s modified eagle’s medium (DMEM) was acquired from GIBCO-BRL (Carlsbad, CA, USA). PercollTM Polyvinylidene difluoride membrane (PVDF) was obtained from GE Healthcare (Piscataway, NJ, USA). All antibodies utilized were acquired from Santa Cruz Biotechnology (Santa Cruz, CA, USA). Enhanced chemiluminescence system (ECL) was obtained from Pierce Biotechnology (Rockford, IL, USA). JC-1 and annexin V were purchased from Molecular Probes (Eugene, OR).
Methods
Isolation of human neutrophils by the gradient density centrifugation method
Venous blood was collected from healthy human volunteers by antecubital venipuncture, into vacuum tubes with EDTA (0.5 %). The isolation of human neutrophils was made by a four-step discontinuous PercollTM gradient, as previously described in Dooley et al. (1982). Residual erythrocytes were removed by hypotonic lysis. Isolated neutrophils (98 % purity) were estimated to be >96 % viable by trypan blue exclusion and were resuspended in DMEM supplemented with 10 % heat-inactivated fetal bovine serum (FBS).
Cellular necrosis
Cellular necrosis was determined by the trypan blue exclusion analysis. Neutrophils were incubated with Ni(II) (0–500 μM) for 18 h at 37 °C. Twenty microliters of neutrophils suspension were added to an equal volume of 0.4 % trypan blue in a microtube and mixed gently. After 2 min on ice, neutrophils number and viability (viable cells excluding trypan blue) were counted. Assays were performed in triplicate.
Assessment of neutrophils’ apoptosis
Cytomorphological alterations
After 6 h of incubation with Ni(II) (0, 250 or 500 μM), in the presence or absence of DPI (10 μM) neutrophils were cytocentrifuged, stained with Diff-Quik, and counted under light microscopy (×1,000) to determine the proportion of cells showing characteristic apoptotic morphology. At least 400 cells were counted per slide. All experiments were performed at least four times.
Annexin V binding assay
To measure phosphatidylserine exposure on apoptotic cell surface, a flow cytometric assay using annexin V binding was performed. After 6 h of incubation with Ni(II), neutrophils were washed with binding buffer (10 mM HEPES (pH 7.4), 150 mM NaCl, 5 mM KCl, 1 mM MgCl2, and 1.8 mM CaCl2, pH 7.4) and resuspended in 100 μL binding buffer containing annexin V conjugated to FITC (1:500 dilution). After 20 min incubation at room temperature in the dark, 400 μL of binding buffer was added and the samples were immediately analyzed in a C6 flow cytometerTM (Accuri).
Preparation of cell extracts for measuring pro-apoptotic signalling proteins
Whole cell lysates were obtained as previously described. Briefly, after 3 h of incubation with Ni (II), neutrophils (5 × 106 cells/mL) were washed and resuspended in lysis buffer [10 mM Tris (pH 7.4), 100 mM NaCl, 1 mM EDTA, 1 mM EGTA, 1 mM NaF, 20 mM Na4P2O7, 2 mM Na3VO4, 0.1 % SDS, 0.5 % sodium deoxycholate, 1 % triton X-100, 10 % glycerol) containing the following protease inhibitors cocktail: 1 mM PMSF, 60 μg/mL aprotinin, 10 μg/mL leupeptin, 1 μg/mL pepsatin. After 20 min incubation on ice, the suspension was centrifuged at 14,000×g for 10 min and supernatant was collected. Total protein content was determined by Bradford method (Bradford 1976).
Analysis of caspase 3 activation by western blot
Caspase activation was analyzed by Western blot in nickel-exposed neutrophils, collected as reported above. For Western blots, Laemmli’s sample buffer containing 50 mM Tris buffer, pH 6.8, 1 % SDS, 5 % β-mercaptoethanol, 10 % glycerol, and 0.001 % bromophenol blue were added to cell pellets and boiled for 5 min. Cellular proteins (30 μg) were subjected to SDS-PAGE on 12 or 15 % acrylamide gel, transferred to PVDF membranes and blocked with TBS-Tween containing 5 % BSA. After this step, blots were exposed to the appropriate primary antibody anti-caspase-3 (1:500) and anti-tubulin (1:1,000), for 18 h at 4 °C. Membranes were washed three times with TBS-Tween, followed by 1 h incubation with appropriate peroxidase-conjugated secondary antibody (1:10,000) followed by enzyme substrate. Immunoreactive proteins were visualized by enhanced chemiluminescence. The bands were quantified by densitometry, using Scion Image Software (Scion Co, Frederick, MD, USA). Scion Image software is built-in calibration system that integrates labeling intensity and dimension of bands against a background measurement converting the data in densitometric units.
Flow cytometry assessment of mitochondrial transmembrane potential
The mitochondrial stability was measured by the use of the cationic dye JC-1, which incorporates to the mitochondrial intermembrane space. The monomer (green) can polymerize forming clusters known as J-aggregates (red) in a transmembrane potential-dependent manner (Fossati et al. 2003). Therefore, viable, non-apoptotic cells exhibit a pronounced reddish fluorescence of mitochondria that can be detectable by flow analysis (in the FL-2 channel). Conversely, apoptotic process results in loss of mitochondrial transmembrane potential (ΔΨm) and a subsequent decrease of the reddish fluorescence shifting to an increase in green fluorescence (as seen in FL-1 channel). Isolated neutrophils (5x106 cells/mL) were stimulated with Ni(II) (125–500 μM), cycloheximide (5 μg/mL, as positive control) or left unstimulated (negative control) and incubated for 3 h at 37 °C in a 5 % CO2 atmosphere. JC-1 dye (10 μg/mL) was then added to each group and after 30-min incubation at 37 °C in 5 % CO2 atmosphere labeled cells suspensions were submitted to Accuri C6 flow cytometerTM analysis. FL-1 (515–545 nm) and FL-2 (564–606 nm) channels were assessed.
Statistical analysis
Statistics were calculated using GraphPad Prism™ (version 5.0; GraphPad Software). Results are expressed as mean ± standard error of the mean (SEM) (from at least four individual experiments, performed in triplicate in each experiment). Statistical comparison between groups was estimated using the one-way analysis of variance (ANOVA), followed by the Bonferroni’s post hoc test. In all cases, p values lower than 0.05 were considered as statistically significant.
Results
Cellular necrosis
According to trypan blue assay, the Ni(II) concentrations used in the current study did not induce cellular necrosis up to 18 h of exposure (graphical data not shown).
Apoptotic cytomorphological alterations
An incubation of human neutrophils with Ni(II) during 6 h was sufficient to alter the morphologic aspect of the cells indicative of apoptosis, as shown in Fig. 1. Indeed, 500 μM of Ni(II) significantly increased the percentage of cells with apoptotic features up to 66.3 ± 5.4 %, compared to 43.3 ± 4.4 % in the control (without metal). The use of DPI, an inhibitor of NADPH oxidase prevented Ni(II)-induced apoptosis down to control levels.
Morphological analysis of neutrophils: A (1) medium alone 0 h; (2) medium alone 6 h; (3) Ni(II) 500 μM 6 h; (4) Ni (II) 500 μM + DPI (10 μM). Arrowheads indicate apoptotic neutrophils. B Graphic representation of morphological analysis of human neutrophils incubated with different concentrations of Ni(II) in the presence or absence of DPI (10 μM). Values are given as mean ± SEM (n ≥ 4). *p < 0.05 significantly different from the control (without metal)
Apoptosis by Annexin V
The pro-apoptotic effect of Ni(II) on human neutrophils was also assessed by annexin V binding assay (Fig. 2). We observed that the incubation of neutrophils with Ni(II) significantly increased the apoptotic rate in a concentration-dependent manner. Once again, DPI prevented the pro-apoptotic effect of Ni(II).
Flow cytometric analysis of annexin V binding assay. A Neutrophils alone at 6 h of incubation; Neutrophils incubated with Ni(II) 250 μM; Neutrophils incubated with Ni(II) 500 μM; Neutrophils incubated with Ni(II) 500 μM + DPI. B Percentage of Annexin V +cells incubated with different concentrations of Ni(II) in the presence or absence of DPI (10 μM). Values are given as mean ± SEM. (n ≥ 4). **p < 0.01 significantly different from the control (without metal)
Caspase-3 activation
In order to understand the mechanism by which Ni(II) induces apoptosis, the activation of caspase-3 was studied. It was clearly demonstrated (Fig. 3) that Ni(II) induces the degradation of procaspase-3, which leads to activation of caspase-3. It was also shown that the incubation of cells with DPI reversed the effect of Ni(II) on caspase activation, confirming the involvement of NADPH oxidase in Ni(II)-induced apoptosis.
Assessment of Δψm
Neutrophils were incubated for 3 h in the absence or in the presence of increasing concentrations of Ni(II) (125–500 μM). Cells were then stained with JC-1 dye for 30 min and analyzed by flow cytometry. The results obtained (Fig. 4) showed that Ni(II) induced an apoptotic process resulting in loss of ΔΨm, well denoted by the decrease of the reddish fluorescence shifting to an increase in green fluorescence (as seen in FL-1/FL-2 channels ratio). In this case DPI did not affect the signal provided by Ni(II).
Discussion
Nickel is ubiquitously present in the environment and the exposure to low levels may cause a variety of adverse effects in human health (Chen et al. 2001; Kasprzak et al. 2003). Nickel is known to accumulate in biological tissues, reaching concentrations of 20–1,000 μM (Lewis et al. 2009). The Ni(II) concentrations used in the current study are within this concentration window, but were selected in such a way that loss of cellular viability by necrosis, under the current experimental conditions, was avoided. Indeed, Ni(II) did not induce necrosis in human neutrophils up to a concentration of 500 μM after 18 h of incubation, corroborating our previous findings (Freitas et al. 2010). We have previously reported that Ni(II), at concentrations that may be attained in vivo, evokes the production of superoxide radical (O •−2 ), hydrogen peroxide (H2O2), and hypochlorous acid (HOCl) in human neutrophils in vitro, via activation of PKC (Freitas et al. 2010). Although Ni(II) is known to induce cellular damage, no research has been done before to evaluate the putative Ni(II)-induced apoptosis. As pointed before, evidence is emerging that these ROS are crucial in the execution of most neutrophil cell death mechanisms. In fact, the majority of molecular pathways causing neutrophil apoptosis were shown to be dependent on ROS generation (Geering and Simon 2011; Simon et al. 2000). As Ni(II) induces oxidative burst, it is possible that Ni(II)-induced ROS are involved in apoptosis. In the present study, apoptosis was initially recognized by its characteristic cellular morphology. During the early process of apoptosis, several events occur in sequence, namely cell shrinkage, loss of cell volume, and higher density of cytoplasm, in which the organelles become tightly packed. When neutrophils were incubated with Ni(II), the number of apoptotic cells increased, appearing as a round or oval mass with dark and dense purple nuclear chromatin fragments. Although the mechanisms and morphologies of apoptosis and necrosis differ, there is overlap between these two processes (Elmore 2007). In fact, with conventional histology it is not always easy to distinguish apoptosis from necrosis, which can occur simultaneously, depending on factors such as the intensity and duration of the stimulus, and the extent of ATP depletion (Zeiss 2003). Apoptosis is a tightly controlled process in which cell death is executed through the activation of specific signaling pathways. Within cells, there are positive and negative regulatory pathways of apoptosis, and it is the balance between these pathways that determines cell fate (Pulido and Parrish 2003). Thus, to confirm the pro-apoptotic pathway, we performed a study of annexin V binding activity. Apoptotic cell death is accompanied by a change in plasma membrane structure by surface exposure of phosphatidylserine (PS), while the membrane integrity remains unchallenged. Surface exposed PS can be detected by its affinity for annexin V, a phospholipid binding protein (van Engeland et al. 1998). The results obtained corroborate the morphological analysis since 250 μM of Ni(II) was sufficient to induce a significant annexin V binding effect. To discriminate between necrotic and apoptotic cells, a membrane impermeable DNA stain, propidium iodide (PI) was added simultaneously to the cell suspension. In this way vital, apoptotic, and necrotic cells can be discriminated on basis of a double-labeling for annexin V and PI (van Engeland et al. 1998). According to our results, the majority of the cells exposed to Ni(II) are annexin V positive suggesting an apoptotic effect of the metal after 6 h of exposure. Nevertheless, a small percentage of neutrophils are PI positive, indicating that Ni(II), although in a lesser extent, also induced necrosis, probably as a late apoptotic event. In principle, there are two alternative pathways that initiate apoptosis: one is mediated by death receptors on the cell surface, sometimes referred to as the extrinsic pathway, while the other is mediated by mitochondria, referred to as the intrinsic pathway or mitochondrial pathway (Igney and Krammer 2002; Rana 2008). The intrinsic signaling pathways that initiate apoptosis are mitochondrial-initiated events, involving a subsequent cascade of intracellular pro-apoptotic signals. During this process, several key events occur in mitochondria, including the release of caspase activators such as cytochrome c, changes in electron transport, and loss of mitochondrial transmembrane potential (ΔΨm). For this reason, the effect of Ni(II) on ΔΨm was also studied. As such, we use the JC-1 dye, which in healthy cells with high mitochondrial ΔΨm, forms complexes known as J-aggregates with intense red fluorescence. On the other hand, in apoptotic cells with low ΔΨm, JC-1 remains the monomeric form, which shows only green fluorescence. Our results clearly demonstrated that Ni(II) alters the electrochemical gradient across the mitochondrial membrane, contributing actively in this important step of cellular apoptosis. These results suggests that Ni(II) induced apoptosis by the intrinsic pathway. This finding is corroborated by M’Bemba-Meka et al. (2005) who reported that NiSO4 induced lymphocyte apoptosis through an alteration on mitochondrial function. Caspases belong to a family of highly conserved cysteine-dependent aspartate-specific acid proteases that use a cysteine residue as the catalytic nucleophile and share a stringent specificity for cleaving their substrates after aspartic acid residues in target proteins (Chowdhury et al. 2008). There are two tiers of caspase activation during apoptosis. Initiator caspases (caspases 2, 8, 9, and 10) are activated through the apoptosis-signaling pathways and activate the effector caspases (caspases 3, 6, and 7) which, in an expanding cascade, carry out apoptosis (Chowdhury et al. 2008). We studied the effect of Ni(II) after 3 h of incubation on activation of caspase-3. Our results indicate that Ni(II) induced a significant activation of this effector caspase, which is corroborated by Au et al. (Au et al. 2006) for Jurkat T cells, a cellular model of T-lymphocyte cells. Reactive species have recently been regarded as important intracellular signaling messengers inducing apoptosis (Elmore 2007; Fialkow et al. 2007; Sim et al. 2005), inclusively in neutrophils (Geering and Simon 2011; Simon et al. 2000). Evidences that apoptosis can be induced by ROS are provided by studies in which mediators of apoptosis, are activated by intracellular production of ROS or are inhibited by the addition of antioxidants (Au et al. 2006; Papa and Skulachev 1997; Sim et al. 2005). We have shown that Ni(II) is able to trigger oxidative burst in human neutrophils (Freitas et al. 2010). As such, our main goal was to understand the involvement of NADPH oxidase on Ni(II)-induced neutrophils apoptosis. For this purpose we use DPI, an NADPH oxidase inhibitor, in all of the standard apoptotic analysis. We demonstrated that Ni(II)-induced apoptosis has the contribution of NADPH oxidase activation since, in some experiments, DPI delayed the apoptosis induced by this metal. These results were corroborated by other authors, such as Au et al. (2006) who demonstrated that Ni(II) exposure results in the generation of ROS, which eventually leads to apoptosis of Jurkat T cells by caspase-3. Also Chen et al. (2010) reported an induction of apoptosis in normal rat kidney cells by NiCl2, accompanied by rising levels of ROS in cells, indicating that some relationship between these two processes produced by Ni(II) are occurring. Another study made by Pan et al. (2010) showed that nickel-induced apoptosis was attenuated by ROS scavengers, indicating that ROS are probably involved in Ni(II)-induced apoptosis in BEAS-2B cells. Nevertheless, we cannot discard that other ROS-generating systems than NADPHox have a role in Ni(II)-induced apoptosis. As the JC-1 assay evidenced, we did not find a relation between NADPH oxidase activation and Ni(II)-induced ΔΨm dissipation, suggesting that Ni(II) induces apoptosis by multiple pathways. In conclusion, in the present study we demonstrated, for the first time, that Ni(II) at concentrations that may be attained in vivo, induces human neutrophils’ apoptosis, through the intrinsic pathway. The use of DPI, an NADPH oxidase inhibitor, delayed Ni(II)-induced apoptosis, suggesting that NADPH oxidase-derived ROS signaling could contribute to this event. This is an important finding since this effect mediated by nickel may counteract the important physiological activities of neutrophils. Thus, as mentioned before, the ability of this metal to activate human neutrophils and then to induce its apoptosis could be one of the key properties of its carcinogenic mechanism, through regulation of tumor cell proliferation, angiogenesis, and metastasis.
References
Amulic B, Cazalet C, Hayes GL, Metzler KD, Zychlinsky A (2012) Neutrophil function: from mechanisms to disease. Annu Rev Immunol 30:459–489
Au A, Ha J, Hernandez M, Polotsky A, Hungerford DS, Frondoza CG (2006) Nickel and vanadium metal ions induce apoptosis of T lymphocyte Jurkat cells. J Biomed Mater Res A 79:512–521
Babior BM (2000) Phagocytes and oxidative stress. Am J Med 109:33–44
Barceloux DG (1999) Nickel. J Toxicol Clin Toxicol 37:239–258
Bradford MM (1976) A rapid and sensitive method for the quantitation of microgram quantities of protein utilizing the principle of protein-dye binding. Anal Biochem 72:248–254
Chen F, Ding M, Castranova V, Shi X (2001) Carcinogenic metals and NF-kappaB activation. Mol Cell Biochem 222:159–171
Chen CY, Wang YF, Huang WR, Huang YT (2003) Nickel induces oxidative stress and genotoxicity in human lymphocytes. Toxicol Appl Pharmacol 189:153–159
Chen CY, Lin TK, Chang YC, Wang YF, Shyu HW, Lin KH, Chou MC (2010) Nickel(II)-induced oxidative stress, apoptosis, G2/M arrest, and genotoxicity in normal rat kidney cells. J Toxicol Environ Health A 73:529–539
Chowdhury I, Tharakan B, Bhat GK (2008) Caspases—an update. Comp Biochem Physiol B 151:10–27
Dooley DC, Simpson JF, Meryman HT (1982) Isolation of large numbers of fully viable human neutrophils: a preparative technique using percoll density gradient centrifugation. Exp Hematol 10:591–599
Elmore S (2007) Apoptosis: a review of programmed cell death. Toxicol Pathol 35:495–516
EMEA (2008) Guideline on the specification limits for residues of metal catalysts or metal reagents. European Medicines Agency
Fialkow L, Wang Y, Downey GP (2007) Reactive oxygen and nitrogen species as signaling molecules regulating neutrophil function. Free Radic Biol Med 42:153–164
Fossati G, Moulding DA, Spiller DG, Moots RJ, White MR, Edwards SW (2003) The mitochondrial network of human neutrophils: role in chemotaxis, phagocytosis, respiratory burst activation, and commitment to apoptosis. J Immunol 170:1964–1972
Freitas M, Fernandes E (2011) Zinc, cadmium and nickel increase the activation of NF-kappaB and the release of cytokines from THP-1 monocytic cells. Metallomics 3:1238–1243
Freitas M, Gomes A, Porto G, Fernandes E (2010) Nickel induces oxidative burst, NF-kappaB activation and interleukin-8 production in human neutrophils. J Biol Inorg Chem 15:1275–1283
Geering B, Simon HU (2011) Peculiarities of cell death mechanisms in neutrophils. Cell Death Differ 18:1457–1469
Igney FH, Krammer PH (2002) Death and anti-death: tumor resistance to apoptosis. Nat Rev Cancer 2:277–288
Kasprzak KS, Sunderman FW Jr, Salnikow K (2003) Nickel carcinogenesis. Mutat Res 533:67–97
Krockova JZ, Massanyi P, Sirotkin AV, Pivko J, Makarevich AV, Lukac N, Capcarova M, Toman R, Polakova Z (2011) Nickel induced structural and functional alterations in mouse Leydig cells in vitro. J Trace Elem Med Biol 25:14–18
Lewis JB, Messer RL, Pitts L, Hsu SD, Hansen JM, Wataha JC (2009) Ni(II) ions dysregulate cytokine secretion from human monocytes. J Biomed Mater Res B Appl Biomater 88:358–365
Liden C, Skare L, Vahter M (2008) Release of nickel from coins and deposition onto skin from coin handling–comparing euro coins and SEK. Contact Dermatitis 59:31–37
Lu H, Shi X, Costa M, Huang C (2005) Carcinogenic effect of nickel compounds. Mol Cell Biochem 279:45–67
M’Bemba-Meka P, Lemieux N, Chakrabarti SK (2005) Role of oxidative stress, mitochondrial membrane potential, and calcium homeostasis in nickel sulfate-induced human lymphocyte death in vitro. Chem Biol Interact 156:69–80
Nielsen F (2007) Trace mineral deficiencies. In: Handbook of Nutrition and Food. Carolyn D. Berdanier, Johanna T. Dwyer, Elaine B. Feldman CRC Press: 159-176
Pan J, Chang Q, Wang X, Son Y, Zhang Z, Chen G, Luo J, Bi Y, Chen F, Shi X (2010) Reactive oxygen species-activated Akt/ASK1/p38 signaling pathway in nickel compound-induced apoptosis in BEAS 2B cells. Chem Res Toxicol 23:568–577
Papa S, Skulachev VP (1997) Reactive oxygen species, mitochondria, apoptosis and aging. Mol Cell Biochem 174:305–319
Pulido MD, Parrish AR (2003) Metal-induced apoptosis: mechanisms. Mutat Res 533:227–241
Rana SVS (2008) Metals and apoptosis: recent developments. J Trace Elem Med Biol 22:262–284
Shiao YH, Lee SH, Kasprzak KS (1998) Cell cycle arrest, apoptosis and p53 expression in nickel(II) acetate-treated Chinese hamster ovary cells. Carcinogenesis 19:1203–1207
Sim S, Yong TS, Park SJ, Im KI, Kong Y, Ryu JS, Min DY, Shin MH (2005) NADPH oxidase-derived reactive oxygen species-mediated activation of ERK1/2 is required for apoptosis of human neutrophils induced by Entamoeba histolytica. J Immunol 174:4279–4288
Simon HU, Haj-Yehia A, Levi-Schaffer F (2000) Role of reactive oxygen species (ROS) in apoptosis induction. Apoptosis 5:415–418
Torres F, das Gracas M, Melo M, Tosti A (2009) Management of contact dermatitis due to nickel allergy: an update. Clin Cosmet Investig Dermatol 2:39–48
Trombetta D, Mondello MR, Cimino F, Cristani M, Pergolizzi S, Saija A (2005) Toxic effect of nickel in an in vitro model of human oral epithelium. Toxicol Lett 159:219–225
van Engeland M, Nieland LJ, Ramaekers FC, Schutte B, Reutelingsperger CP (1998) Annexin V-affinity assay: a review on an apoptosis detection system based on phosphatidylserine exposure. Cytometry 31:1–9
Zeiss CJ (2003) The apoptosis-necrosis continuum: insights from genetically altered mice. Vet Pathol 40:481–495
Zhang B, Hirahashi J, Cullere X, Mayadas TN (2003) Elucidation of molecular events leading to neutrophil apoptosis following phagocytosis: cross-talk between caspase 8, reactive oxygen species, and MAPK/ERK activation. J Biol Chem 278:28443–28454
Acknowledgments
We would like to thank Genilson Rodrigues and Renata Tureta for their technical support. This work was supported by Conselho Nacional de Desenvolvimento Científico e Tecnológico (CNPq), Coordenação de Aperfeiçoamento de Pessoal de Nível Superior (CAPES), Fundação de Amparo à Pesquisa do Estado do Rio de Janeiro (FAPERJ). This work has been supported by Fundação para a Ciência e a Tecnologia through grant no. PEst-C/EQB/LA0006/2011. Marisa Freitas acknowledges Fundação para a Ciência e Tecnologia the financial support for the Pos-doc (SFRH/BPD/76909/2011) grant in the ambit of “POPH-QREN-Tipologia 4.1-Formação Avançada” co-sponsored by FSE and national funds of MCTES.
Author information
Authors and Affiliations
Corresponding author
Rights and permissions
About this article
Cite this article
Freitas, M., Barcellos-de-Souza, P., Barja-Fidalgo, C. et al. Nickel induces apoptosis in human neutrophils. Biometals 26, 13–21 (2013). https://doi.org/10.1007/s10534-012-9590-2
Received:
Accepted:
Published:
Issue Date:
DOI: https://doi.org/10.1007/s10534-012-9590-2