Abstract
Slow or nonexistent natural recovery of the Caribbean long-spined sea urchin (Diadema antillarum) following a mass mortality event in 1983–1984 has prompted interest in hatchery-origin production and restocking to aid coral-reef restoration. A critical first step is the ability to propagate D. antillarum from gametes, at scale. However, a unique larval biology and difficult and lengthy culture period of ~ 40 days has resulted in inconsistent success over the past 20-plus years. The purpose of this study was to develop protocols for rearing D. antillarum within a novel 1800-L recirculating aquaculture system capable of scaled production. Five separate experiments investigated larval development in response to diet quantity, diet composition, and initial stocking density within 40-L replicate culture tanks. The initial experiment was used to develop a microalgae reference diet consisting of Tisochrysis lutea and Chaetoceros sp. and revealed similar growth and survival between high quantity (40.0 × 103 cells mL−1) and low quantity (10.0 × 103 cells mL−1) treatments at 21 days post-fertilization (DPF). Experiments 2–4 examined diet quality by comparing carbon-equivalent microalgae compositions. Mixed diets containing Rhodomonas lens outperformed the reference diet in multiple experiments and a tripartite diet containing all three species resulted in significantly higher survival at 42 DPF. The highest growth overall occurred from a monoalgal R. lens diet, which indicated that this species is critically important. Further observations of density-dependent growth dynamics revealed that initial stocking densities > 1 larvae mL−1 significantly reduced growth over 28 DPF. Data generated were used to establish fundamental larviculture protocols that have since led to the production of over 1000 juveniles.
Similar content being viewed by others
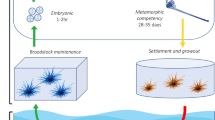
Explore related subjects
Discover the latest articles, news and stories from top researchers in related subjects.Avoid common mistakes on your manuscript.
Introduction
Aquaculture has been increasingly utilized in recent years to attempt enhancement of populations of organisms for ecological restoration with the goal of recovering and increasing ecosystem services (Alleway et al. 2019; Lorenzen 2014; Patterson 2019). Relevant examples of aquaculture-based ecological enhancements include Crassostrea virginica (Jaris et al. 2019), Zostera marina (Tanner and Parham 2010), Tripneustes gratilla (Neilson et al. 2018; Westbrook et al. 2015), and coral (Boström-Einarsson et al. 2020; Leal et al. 2016; Lirman and Schopmeyer 2016) restoration. Unprecedented global declines of coral reefs have resulted in the rapid growth of propagation programs attempting direct enhancement of ecologically important coral species (Barton et al. 2017; Osinga et al. 2011; Rinkevich 1995). Restoring propagated corals alone, however, does not address the stressors that led to reef decline and long-term outplant survival can be low (Ware et al. 2020). Practically, coral enhancement should exist within a larger reef restoration framework that aims to restore structural habitat and biodiversity via a multi-niche ecological approach. Key to this approach in the Caribbean is the re-establishment of lost herbivory via enhancement of long-spined sea urchin, Diadema antillarum. Historically, this species was the primary generalist herbivore on Caribbean reefs (Ogden 1977; Sammarco 1982), until a mass mortality event in 1983–1984 reduced populations by 93–100% and pervasively altered reef ecosystem dynamics via reduced herbivory and subsequent declines of hard coral cover, habitat complexity, and biodiversity (Hughes et al. 2010; Lessios 2016).
Despite several established methods to commercially produce echinoderms from gametes (Harris and Eddy 2015; McBride 2005), and implementation of aquaculture-based urchin enhancements on coral reefs in Hawaii (Neilson et al. 2018; Westbrook et al. 2015), a scalable hatchery process for D. antillarum has not yet been established. Past attempts to culture this species over the last 20-plus years have been met with varying degrees of success. Eckert (1998) recorded the first instance of complete development, having produced 5 juveniles after a 36-day larviculture period within 1-L plastic beakers with mechanical paddle stirrers. Subsequent failed culture attempts and anecdotal reports of difficulties from other culturists led the same author to conclude that, “[D. antillarum] seems very sensitive to poor culturing conditions”, and that the larvae could be particularly susceptible to pathogens and/or have specific nutritional requirements leading up to metamorphosis. Water quality is a primary, related concern as abnormal embryo-larval development occurs in response to dissolved metal concentrations as low as 15 and 11 µg/L of nickel and copper, respectively (Bielmyer et al. 2005). A unique larval morphology poses additional challenges. Compared to more commonly cultured species, which exhibit a typical echinopluteus larval form, D. antillarum larvae are characterized as echinopluteus transversus, with two distinctly long postoral arms protruding at low angles of elevation from the larval body (Fig. 1b).
This trait is disadvantageous to captive larviculture as relatively turbulent flow dynamics result in mechanical damage to the postoral arms, increased infection potential, and diminished swimming and feeding capacity. Additionally, there are physiological constraints on growth, including reduced feeding, digestive and metabolic efficiency, that are possibly inherent to transversus form larvae (Rendleman and Pace 2018). Cumulatively, these constraints could contribute to the long, 2–8 month (Hernandez et al. 2006) and 28-day plus D. antillarum planktotrophic larval duration observed in the wild and in captivity, respectively.
Continued efforts to culture D. antillarum led to the creation of a prototype system with the potential for mass culture (Moe 2014). Still, speculative factors including environmental toxins, water quality, and general culture methods precluded reliable development through metamorphosis (Leber et al. 2009). Outstanding restoration goals led to the development of a novel recirculating aquaculture system (RAS) to investigate larviculture bottlenecks and improve the feasibility of scaled production (Pilnick et al. 2021). These culture efforts represented the first attempt to culture D. antillarum within a RAS and have to-date produced over 1000 juveniles. As with any novel intensive aquaculture system, an investigation of appropriate culture protocols was required to achieve successful development.
Practically, diet quantity, diet composition, and initial larval stocking density are important considerations for D. antillarum larviculture, given that the development of planktotrophic sea urchin larvae is strongly linked to exogenous feeding (Bertram and Strathmann 1998) and that sea urchin larvae are subjected to competition for resources and space within culture tanks (Azad et al. 2012). Different food quantities, feeding frequencies, and species of live microalgae, used for captive larval diets, can directly influence larval growth, survival, and metamorphic success (Carboni et al. 2012; Cárcamo et al. 2005; Kelly et al. 2000; Liu et al. 2007) and appear to be species-specific. The nutritional quality of different microalgae species can vary depending on factors such as cell size, digestibility, and biochemical composition (Guedes and Malcata 2012). While mixed microalgae diets generally perform better (Azad et al. 2011; Carcámo et al. 2005; Gomes et al. 2021), it has been suggested that the cryptophyte Rhodomonas lens is an essential dietary component for larval D. antillarum (Eckert 1998; Leber et al. 2009). Other microalgae highly regarded for marine larviculture used in prior culture attempts include Tisochrysis lutea (formerly Isochrysis galbana), Chaetoceros gracilis, and Dunaliella tertiolecta (Eckert 1998; Leber et al. 2009; Moe 2014). However, a diet protocol for rearing D. antillarum within a production-oriented RAS has not yet been developed. Likewise, few studies have investigated the impact of stocking density on sea urchin larviculture within commercial production settings (Azad et al. 2011; Buitrago et al. 2005; Suckling et al. 2018). Apart from a single experiment conducted in small-scale 1.3-L culture vessels that revealed an inverse relationship between larval density and growth (Leber et al. 2009), a determination of appropriate D. antillarum stocking density within a production-oriented RAS has also not yet occurred. Larval nutrition and stocking density remain extremely relevant for D. antillarum larviculture, especially when considering the feasibility of production for restoration. The current study outlines an experimental process used to determine an appropriate (1) diet quantity, (2) diet composition, and (3) initial larval stocking density for D. antillarum larviculture within a novel RAS capable of scaled production for restoration.
Methods
Broodstock maintenance and spawning
Adult D. antillarum were collected in March 2018 from reefs at < 8-m depth off Marathon, Florida, by the Florida Fish and Wildlife Research Institute under Florida Keys National Marine Sanctuary permit # FKNMS-2018–023. These broodstock were transported to a land-based restoration aquaculture facility operated by The Florida Aquarium in Apollo Beach, FL. Urchins were quarantined in a RAS within a greenhouse for 45 days. Following quarantine, 14 broodstock were transferred to 450-L fiberglass tanks within a 2400-L seawater RAS. Broodstock husbandry adhered to methods previously described in Pilnick et al. (2021).
Spawning was induced via thermal shock following methods described in Leber et al. (2009) and Moe (2014). Broodstock were transferred to a polyethylene tank filled with 150-L of 1-µm filtered 35 ppt artificial seawater (ASW) prepared by mixing a commercial grade marine salt (Tropic Marin, Wartenburg, Germany) and municipal freshwater purified by reverse osmosis and de-ionization. Water was heated to 5 °C above holding temperature and aeration was provided. Eggs were collected in a 60-mL syringe following release from females and gently rinsed in 2-L egg collectors with 35-µm mesh. Typically, males released gametes first and eggs were fertilized upon collection by residual sperm in the spawning bin. Fecundity and fertilization rates were assessed via 1-mL volumetric subsample counts using a Sedgewick rafter counting slide.
General larval rearing methods
Larval rearing followed the methods established in Pilnick et al. (2021). Fewer than 1 million embryos were incubated at 25–26 °C in semi-circular 40-L culture tanks with pulsed aeration supplied through perforated rigid tubing at intervals of 3–5 s on and 20–30 s off. At 3 days post-fertilization (DPF), pluteus larvae were volumetrically transferred to separate, randomly assigned 40-L replicate culture tanks within a single 1800-L RAS (system picture and schematic supplied in Pilnick et al. 2021). Pulsed aeration within replicate tanks suspended negatively buoyant larvae throughout the culture period. ASW was used for incubation and larval culture throughout. Water quality parameters remained within the range of those described in Pilnick et al. (2021). Feeding with live microalgae was initiated at 3 DPF. Larvae were exposed to food for a 16-h static feeding period during which flow-through from the RAS was turned off. Filtered water from header tanks in-line with the RAS was subsequently sent to the culture tanks at 1–2 LPM for an 8-h flushing period. Effluent wastewater passed through a 5-µm filter sock and protein skimmer (Reef Octopus Regal 200-INT, Honya Co. Ltd, Shenzhen, China), 20-µm cartridge filters, and 50-W ultraviolet filtration to remove uneaten microalgae before water returned to the header tank. Microalgae stock cultures were obtained from the University of Florida Tropical Aquaculture Lab or purchased from a commercial culture facility (AlgaGen LLC, Vero Beach, FL, USA) at regular intervals. Growth of these cultures was extended by the addition of modified F/2 nutrient medium with reduced copper (AlgaGen LLC, Vero Beach, FL, USA) and sterilized ASW.
Experiments
Five separate experiments were conducted to iteratively evaluate the impacts of diet quantity, diet composition, and initial stocking density on larval growth and survival. All experiments began at 3 DPF, except for the fourth experiment which was re-initiated with surplus larvae at 6 DPF following an unexpected culture crash. The duration of each experiment ranged from 21 to 42 DPF. Various microalgae diets included T. lutea, C. gracilis, C. muelleri, and R. lens and are described for each experiment below. Diet treatments in experiments 1–4 were standardized based on microalgal carbon content, as described in Strathmann (1967). In mixed diets containing more than one species, each species contributed an equal proportion of carbon (pg) to the total pool. In experiments comparing diet compositions, total carbon content was also equivalent between treatments. The carbon content values for T. lutea, C. gracilis, and R. lens were obtained from Ohs et al. (2010) and equaled 7.0, 15.0, and 40.7 pg cell−1, respectively. Both C. muelleri and C. gracilis were assigned a carbon content value of 15.0 pg cell−1 due to similar values for C. muelleri reported by Leonardos and Geider (2004) and were used interchangeably in this study. Microalgae culture densities were enumerated daily with a hemocytometer and used to calculate volumes to feed to each replicate tank. Excluding the first experiment, the total amount of microalgae fed increased equivalently at 14 DPF across all treatments. Table 1 contains information on microalgae diet composition, quantity, and larval stocking density for each treatment level for the following experiments.
Experiment 1, diet quantity
A reference diet consisting of T. lutea and C. muelleri was used in an initial experiment to determine an appropriate microalgal cell concentration for early larval development. Larval performance on high (40 × 103 cells mL−1) and low (10 × 103 cells mL−1) quantity diets was compared over 21 DPF (n = 5 replicate tanks/treatment). This duration was chosen due to interest in early larval development. The target initial stocking density for each replicate tank was 4 larvae mL−1.
Experiment 2, diet composition
A diet composition experiment was conducted to compare larval performance among three diet treatments (n = 4 replicate tanks/treatment) over 21 DPF: (1) T. lutea + C. muelleri (reference diet), (2) R. lens + T. lutea, and (3) R. lens + C. muelleri. This duration was also chosen due to interest in early larval development. The target initial stocking density for each replicate tank was 4 larvae mL−1.
Experiment 3, diet composition
A subsequent diet composition experiment was conducted to compare larval performance among (1) T. lutea + C. gracilis (reference diet, with substitution of C. muelleri with C. gracilis), (2) R. lens + C. gracilis (the numerically best performing diet from experiment 2), and (3) R. lens + T. lutea + C. gracilis (a tripartite diet with higher microalgal diversity) over 42 DPF (n = 4 replicate tanks/treatment) to capture full larval development. The target initial stocking density for each replicate tank was lowered to 2 larvae mL−1.
Experiment 4, diet composition
A desire to economize larviculture methods justified an investigation of mixed and monoalgal diets. Larval performance was compared between two diet treatments, (1) R. lens + C. gracilis, and (2) R. lens over 28 DPF (n = 3 replicate tanks/treatment), at which point the experiment was concluded to maintain adequate replication. The target initial stocking density for each replicate tank was 2 larvae mL−1.
Experiment 5, initial larval stocking density
Density-dependent growth dynamics observed in experiments 3–4 indicated that reduced larval densities resulted in higher growth. Thus, an experiment was conducted to evaluate performance among three initial target stocking densities, (1) ~ 0.75 larvae mL−1, (2) ~ 1.5 larvae mL−1, and (3) ~ 2.25 larvae mL−1 over 35 DPF (n = 3 replicate tanks/treatment), at which point the experiment was concluded to maintain adequate replication. All replicate tanks were fed a monoalgal diet of R. lens throughout.
Data collection
Larval performance was evaluated by estimating survival and analyzing growth at weekly or biweekly intervals beginning at 3 DPF. Larval density (larvae mL−1) within each replicate tank was estimated from triplicate 10-mL subsample counts performed using a plankton wheel counter. Subsample means were used as the value for each replicate tank. Percent survival was calculated based on the estimated number of larvae remaining at the final day relative to initial stocking. To estimate growth, morphometric data was analyzed from larvae within each replicate tank. A homogenized volume of water containing approximately 30 larvae was concentrated using a 100- or 200-µm mesh sieve and transferred to a Sedgewick rafter counting slide. All larvae were photographed using a compound microscope at 4 × or 10 × magnification depending on body size. A minimum of 12 dorsoventrally oriented individuals were haphazardly selected for measurements. An image analysis software (Motic Images Plus 3.0) was used to measure larval body length (BL) and body width (BW) (Fig. 1). Larval body area was calculated using the formula for the area of an ellipse (Formula 1). Mean larval body area from subsampled larvae was used as the value for each replicate tank. Postoral arm length was not included as a factor of interest due to the tendency for mechanical damage during growth in this species.
Statistical analysis
Larval growth, as measured by mean body area, was compared between treatments with either an unpaired t-test or ANOVA at the final time point. Assumptions of normally distributed data and homogeneity of variances were assessed graphically and confirmed with Shapiro–Wilk and Bartlett’s tests, respectively. Following an ANOVA, pairwise post hoc analyses were performed with Tukey’s HSD test. Survival data were analyzed statistically by comparing the proportion of estimated number of larvae alive to the estimated number of larvae not alive (larvae stocked at the initial time point minus larvae alive at the final time point) at the final time point using a logistic regression (glm function in R, family = quasibinomial) followed by a Wald-chi-square test. For trials with greater than 2 treatment groups, pairwise comparisons of survival data were further analyzed with a Tukey HSD post hoc test on the log odds of survival. A p-value of 0.05 was used to assess significance. All analyses were performed with R (R Core Team 2021). All means are reported as mean ± standard error.
Results
Detailed information for each spawn used to initiate an experiment is provided in Table 2.
Experiment 1, diet quantity
An initial experiment was conducted to approximate an appropriate microalgae diet quantity and to establish a reference diet within the novel larval rearing system. No significant differences in body size (t = 2.28, df = 8, p = 0.054) or survival (glm, p > 0.05) were detected between the 40.0 × 103 cells mL−1 and 10 × 103 cells mL−1 diets at 21 DPF (Fig. 2a, b). Survival was 26.02% ± 2.28 and 17.03% ± 3.77 for the high and low quantity diets, respectively. Larval performance overall was similar between diets.
Larval body size (area; µm2 × 103) and density (larvae mL−1) presented for experiment 1 (a and b), experiment 2 (c and d), experiment 3 (e and f), experiment 4 (g and h), and experiment 5 (i and j) over time. Lower case alphabetical letters adjacent to size and density curves denote significant differences between treatments. Data points are presented as means ± standard error. Microalgae species included Tisochrysis lutea, Chaetoceros muelleri, Chaetoceros gracilis, and Rhodomonas lens
Experiment 2, diet composition
After approximating an appropriate diet quantity, subsequent experiments were conducted to identify high-performing microalgae species and carbon equivalent species combinations. Significantly lower survival (glm, p < 0.05) was observed from the reference diet (15.30% ± 5.84) than from R. lens + T. lutea (41.14% ± 4.43) and R. lens + C. muelleri (52.33% ± 9.55) at 21 DPF (Fig. 2d). Similarly, both diets containing R. lens resulted in significantly greater body size compared to the reference diet (F = 16.33, df = 2, p < 0.05) (Fig. 2c). The incorporation of carbon equivalent amounts of R. lens improved larval performance.
Experiment 3, diet composition
A second diet composition experiment was conducted to test larval performance with mixed diets containing R. lens, including a novel diet with three algae species, over a longer duration. A significant difference in survival was detected among all three diet treatments at 42 DPF (glm, p < 0.005) (Fig. 2f). Survival, in order of decreasing performance, resulted from the tripartite R. lens + T. lutea + C. gracilis sp. diet (26.00% ± 5.23), R. lens + C. gracilis (12.28% ± 3.88), and the reference diet (1.05% ± 0.49). While further diet diversification improved survival, no significant difference was observed in body size among any of the treatments (F = 3.38, df = 3, p = 0.09) (Fig. 2e).
Experiment 4, diet composition
Following the identification of an individually important microalgae species (R. lens), experiment 4 was conducted to test the feasibility of culturing D. antillarum on a monoalgal diet. Significantly higher survival (glm, p < 0.005) resulted from the R. lens + C. gracilis diet (76.93% ± 4.46) compared to the monoalgal R. lens diet (47.15 ± 4.69) at 28 DPF (Fig. 2h). Despite improved survival from the mixed diet, the monoalgal R. lens diet resulted in significantly larger body size at 28 DPF (t = − 5.25, df = 4, p = 0.01) (Fig. 2g).
Experiment 5, initial larval stocking density
Observations indicating density-dependent growth dynamics led to an investigation of the influence of initial stocking density on larval performance. No significant difference in survival was observed among the 0.75 larvae mL−1 (27.63% ± 14.07), 1.50 larvae mL−1 (25.31% ± 4.14), and 2.25 larvae mL−1 (20.31% ± 3.47) treatments at 35 DPF (glm, p = 0.82) (Fig. 2j). While survival was not impacted, body size from the 0.75 larvae mL−1 treatment was significantly greater than from the other higher density treatments (F = 40.36, df = 2, p < 0.005) (Fig. 2i).
Discussion
Determining a microalgal cell concentration appropriate for the specific feeding regime within the novel RAS was an essential first step towards developing production-oriented D. antillarum culture protocols. Broadly, Hodin et al. (2019) state that most echinoderm larvae grow rapidly when fed between 5.0 and 10.0 × 103 cells mL−1 of ~ 10-µm diameter live microalgae every 2–3 days. Optimal cell concentrations, however, can be species-specific and depend on the microalgae type and feeding regime used. Two separate small-scale D. antillarum culture attempts reported the most success when larvae were exposed to 5.0–10.0 × 103 cells mL−1 mixtures of R. lens + T. lutea (Eckert 1998), and different 10.0 × 103 cells mL−1 combinations of T. luteau, C. gracilis, and R. lens (Leber et al. 2009). These feedings occurred every 3–7 days after 100% water exchange in standalone 1–2.4-L vessels. Larger-scale culture attempts in standalone 50-L vessels were supplied between 30.0 and 50.0 × 103 cells mL−1 combinations of R. lens + T. lutea + C. gracilis every 3–4 days after 100% water exchange (Moe 2014). Production-oriented recirculating or flow-through culture systems require more frequent feeding of fewer cells than standalone tanks due to constant or periodic water exchange and active removal of unconsumed food. For example, Paracentrotus lividus larvae exhibit greater survival and similar growth when cultured in flow-through systems and fed daily with 1.0–6.0 × 103 cells mL−1 compared to standalone tanks fed every 3 days with 3.0–18.0 × 103 cells mL−1 (Carboni et al. 2012). In the present study, D. antillarum were fed every 24 h after 8 h of flow-through representing at least 100% water exchange. In experiment 1, growth and survival between the 10.0 × 103 and 40.0 × 103 cells mL−1 treatment combinations of T. lutea + C. muelleri were similar. This suggested that larvae were not limited by the lower cell concentration over 21 DPF with this feeding regime and diet composition. An even lower initial concentration of 4.4 × 103 cells mL−1 of R. lens + C. muelleri improved growth and survival compared to 10.0 × 103 cells mL−1 of T. lutea + C. muelleri in experiment 2. Disregarding differences in diet quality between these treatments, this result suggests that particle encounter rates were likely sufficient down to 4.4–10.0 × 103 cells mL−1 over a 16-h feeding period. Low initial cell concentrations were preferred to limit the amount of unconsumed food and reduce the risk of fouling and disease. Despite the lack of statistical differences in growth between treatments in experiment 1, numerical divergence between treatments after 14 DPF suggested that moderately increasing the cell concentration at this timepoint could be beneficial. Regardless, similarly poor survival from both treatments averaging 17–26% indicated that some factor(s) other than food quantity, such as food quality and/or larval stocking density, impacted performance.
Comparisons between carbon-equivalent microalgae compositions in experiments 2–4 revealed the importance of diet quality on D. antillarum larval development. Larvae were stocked at equivalent densities across treatments within each experiment, unlikely to be limited by algal cell concentrations and had access to the same quantity of dietary carbon. Therefore, differences in larval performance likely resulted from different algal compositions. The reference diet developed in experiment 1 (T. lutea + C. muelleri) also helped to improve the quality of diets over successive experiments through comparisons to a benchmark (Glencross et al. 2007). The incorporation of R. lens improved D. antillarum larval performance, corroborating prior recommendations (Eckert 1998; Leber et al. 2009; Moe 2014). Both diets containing R. lens in experiment 2 significantly improved growth and survival at 21 DPF compared to the reference diet without R. lens. A similar trend extended into late larval development, as both diets containing R. lens in experiment 3 significantly improved survival at 42 DPF compared to the reference diet. The apparent dietary benefit of this microalgae is not unique to D. antillarum. Rhodomonas spp. have been deemed a high-quality diet for numerous filter-feeding invertebrates including copepods (Dayras et al. 2021; Knuckey et al. 2005; Ohs et al. 2010), artemia (Seixas et al. 2009), rotifers (Coutinho et al. 2020), scallops (Tremblay et al. 2007), oysters (Brown et al. 1998), mussels (Jose Fernández-Reiriz et al. 2015), and sea urchins (Castilla-Gavilán et al. 2018; Gomes et al. 2021; Hinegardner 1969). Nutritional factors, including cell size and morphology, biochemical composition, and/or digestibility, vary by microalgae species (Brown et al. 1997; Guedes and Malcata 2012) and can help to explain improved D. antillarum larval performances from diets containing R. lens.
Filter feeding invertebrates can ingest a variety of food particles; however, optimum size ranges exist (Fernandez 2001; Lavens and Sorgeloos 1996) and larger, yet still ingestible, microalgae are thought to improve growth (Cárcamo et al. 2005; Fernández-Reiriz et al. 2015; Seixas et al. 2009). Echinoderm larvae cannot actively select food prior to ingestion and instead consume particles that can be captured efficiently and passed through the esophagus into the gut (Strathmann 1971). The ideal particle size range for larval D. antillarum is unknown; however, smaller particles are thought to be less readily captured by urchin larvae than larger ingestible particles (Strathmann et al. 1972). Thus, it is possible that fewer T. lutea and Chaetoceros sp. (3–5-µm and 5–8-µm, respectively [Brown et al. 1997]) cells were captured and ingested when compared to larger R. lens cells (8–12-µm [Brown et al. 1997]). Increased cell size and capture efficiency is unlikely to fully explain improved D. antillarum larval performance as a similarly sized and commonly used microalgae species, Dunaliella tertiolecta (10–12-µm [Brown et al. 1997]), yielded poor results in other studies (Leber et al. 2009; Wijers et al. unpublished data). Rhodomonas spp. have favorable biochemical properties for marine aquaculture in general due to desirable fatty acid profiles and relatively high protein and carbohydrate contents (Brown et al. 1997; Castilla-Gavilán et al. 2018; Coutinho et al. 2020; Dunstan et al. 2005; Fernández-Reiriz et al. 2015; Seixas et al. 2009). These microalgae and other cryptophytes can notably synthesize a diversity of polyunsaturated fatty acids (PUFAs) including eicosapentaenoic acid (EPA, 20:5 n-3) and docosahexaenoic acid (DHA; 22:6 n-3) (Peltomaa et al. 2018), which are both essential fatty acids for healthy marine larval development (Sorgeloos et al. 1998). The importance of individual or relative proportions of these long-chain PUFAs for D. antillarum development is unknown, as is their ability to elongate and desaturate their precursors, a feature which has already been documented in other urchin species (Liu et al. 2007; Schiopu et al. 2006). Interestingly, Chaetoceros spp. and T. lutea also contain relatively high amounts of long-chain PUFAs, but neither are rich in both EPA and DHA (Brown et al. 1997). In the present study, these species underperformed when combined in the absence of R. lens. Castilla-Gavilán et al. (2018) demonstrated that Paracentrotus lividus larvae fed Rhodomonas sp. contained higher total lipid content than those fed other microalgae. A similar dynamic could have positive implications for larval D. antillarum development.
Dietary protein is an important nutritional source of nitrogen and amino acids and can directly influence growth rates of marine invertebrates (Enright et al. 1986; Kreeger and Langdon 1993). Direct comparisons of protein content between all three microalgae species used in this study are unavailable and can vary depending on culture conditions. Despite this, R. lens has been shown to produce and contain higher levels of total protein than T. lutea (Fernández-Reiriz et al. 2015; Seixas et al. 2009) and T. lutea has also been shown to have higher proportional protein content than C. gracilis (Lora-Vilchis et al. 2004). Rhodomonas spp. produce a phycobiliprotein pigment called phycoerythrin that can account for up to 12% of total protein content (Seixas et al. 2009) and may have additional undescribed nutritional benefits. Nutrients within poorly digestible cells are unlikely to be assimilated effectively, regardless of cell size or biochemical composition. The digestive capabilities of larval D. antillarum are unknown; however, enzymes capable of hydrolyzing carbohydrates, lipids, and proteins have been described from other urchin species (Annunziata et al. 2014; Fenaux 1982; Stumpp et al. 2013) and larvae are thought to prioritize defecating less digestible particles (Strathmann 1971). Diet quality in this study could have been affected by differences in digestibility of microalgae cells and respective degrees of nutrient and energy assimilation. In separate studies, mussel and oyster species exhibited higher absorption rates and efficiencies when fed Rhodomonas sp. compared to T. lutea (Fernández-Reiriz et al. 2015; González-Araya et al. 2012). Larval sea urchins reportedly do not break down T. lutea within the gut as easily as other microalgae and, while diatoms are thought to be digestible (Strathmann 1971), the silica-based frustules that comprise Chaetoceros spp. cell walls could be more recalcitrant than R. lens membranes.
While R. lens appeared to be a crucial dietary component, D. antillarum nonetheless benefited from mixed microalgae diets. A comparison of mono-algal R. lens and mixed R. lens + C. gracilis diets in experiment 4 resulted in significantly higher survival from the mixed diet. Similarly, the tripartite diet in experiment 3 resulted in significantly higher survival compared to the R. lens + C. gracilis diet, which indicated a possible positive correlation between overall diet diversity and performance. This is unsurprising, given that mixed diets are more likely to provide nutritional balance (Brown et al. 1997; Ohs et al. 2010) and have been shown to improve survival of other sea urchin larvae (Gomes et al. 2021). The comparative importance of T. lutea or Chaetoceros sp. as supplements to R. lens is unclear given statistically similar growth and survival between mixed diets in experiment 2. However, R. lens + Chaetoceros sp. diets performed well and produced the numerically largest larvae in experiments 2 and 3 and the statistically highest survival in experiment 4. Despite improved survival from higher diversity diets and potential benefits from the inclusion of a diatom, the mono-algal R. lens diet in experiment 4 produced significantly larger larvae than the mixed diet and the highest growth overall in this study. This suggests that, as for other sea urchin species (Castilla-Gavilán et al. 2018; Hinegardner 1969), Rhodomonas sp. can viably be used as a mono-algal diet for D. antillarum.
A density-dependent relationship between larval survival and growth was observed and should be considered concurrently to diet quantity and quality. Higher survival from the mixed-algal diet treatment in experiment 4 corresponded with lower growth, indicating an inverse relationship between larval density and growth. Similarly, the tripartite diet in experiment 3 resulted in the highest survival and lowest growth. Within the same experiment, the production of unexpectedly large larvae from the reference diet treatment may have resulted from a drastic increase in algal cells per larvae due to extremely low survival of ~ 1%. In these instances, reduced survival and lower larval densities potentially lessened competition for physical space and/or resources, including food, leading to higher growth. This dynamic has been observed in the larval culture of other sea urchin species (Azad et al. 2012; Buitrago et al. 2005; Suckling et al. 2018) and warranted investigation in D. antillarum. Experiment 5 was conducted to test the hypothesis that, other factors being equal, D. antillarum growth and survival improves at lower larval densities. Indeed, a similar trend revealed that the lowest initial stocking density resulted in significantly larger larvae than higher density treatments. While survival was statistically similar between all three larval density treatments, proportionally fewer larvae remained in the higher density cultures. Statistical differences could have resulted from extending the experiment past 35 DPF or from stocking the 1.5 and 2.25 larvae mL−1 treatments at higher initial densities. Regardless of initial stocking densities and diet compositions, experiments extending past 30 DPF (experiments 3 and 5) into late larval development concluded at average densities less than 1 larvae mL−1. This observation, in conjunction with the highest growth observed from the 0.75 larvae mL−1 treatment in experiment 5, supports culturing D. antillarum at initial densities ≤ 1 larvae mL−1.
This study represents a series of investigations intended to develop D. antillarum larval culture protocols within a novel RAS capable of scaled production for restoration. Variables of interest included (1) microalgae diet quantity, (2) microalgae diet composition, and (3) initial larval stocking density, which improved outcomes over multiple culture attempts. In summary, daily fed microalgae concentrations down to 4.4–10.0 × 103 cells mL−1 were adequate for rearing D. antillarum over 21 DPF with at least 100% daily water exchange. Gradually increasing cell concentrations as larvae grow and presumably increase consumption rates past this point was likely beneficial, but this was not empirically confirmed. The microalgae R. lens was a critical dietary component and other Rhodomonas species with similar nutritional profiles are likely to perform equally well. Increasingly diverse mixed microalgal diets containing R. lens improved larval survival and supplementing with a diatom may have been beneficial. Lastly, density-dependent growth dynamics were observed, whereby reduced larval densities resulted in higher growth. Production-oriented D. antillarum larval cultures should be conducted at densities ≤ 1 larvae mL−1. The experimentally derived protocols outlined here eventually resulted in complete development of this species within the novel RAS (Pilnick et al. 2021). Over 1000 juveniles have been produced to-date and have been utilized for pilot-scale restocking studies as well as juvenile behavior and growout research (Hassan et al. unpublished data). Further larval culture optimizations aimed at improving yields are yet necessary. Suggestions for additional research include determining prey capture and consumption rates, digestion efficiencies of different microalgae, and types and ratios of supplementary microalgae to best support R. lens diets. Subsequent understanding of how larval nutrition affects settlement and post-settlement success will further improve production viability. Continued investigations into juvenile growout and restocking methods can further advance D. antillarum restoration objectives.
Data availability
The datasets generated during and/or analyzed during the current study are available from the corresponding author on reasonable request.
References
Alleway HK et al (2019) The ecosystem services of marine aquaculture: valuing benefits to people and nature. Bioscience 69(1):59–68. https://doi.org/10.1093/biosci/biy137
Annunziata R et al (2014) Pattern and process during sea urchin gut morphogenesis: the regulatory landscape. Genesis 52(3):251–268. https://doi.org/10.1002/dvg.22738
Azad AK, Pearce CM, McKinley RS (2011) Influence of microalgal species and dietary rations on larval development and survival of the purple sea urchin, Strongylocentrotus purpuratus (Stimpson, 1857). Aquaculture 322:210–217. https://doi.org/10.1016/j.aquaculture.2011.09.029
Azad AK, Pearce CM, McKinley RS (2012) Influence of stocking density and temperature on early development and survival of the purple sea urchin, Strongylocentrotus purpuratus (Stimpson, 1857). Aquac Res 43(11):1577–1591. https://doi.org/10.1111/j.1365-2109.2011.02960.x
Barton JA, Willis BL, Hutson KS (2017) Coral propagation: a review of techniques for ornamental trade and reef restoration. Rev Aquac 9:238–256. https://doi.org/10.1111/raq.12135
Bertram DF, Strathmann RR (1998) Effects of maternal and larval nutrition on growth and form of planktotrophic larvae. Ecology 79(1):315–327. https://doi.org/10.1890/0012-9658(1998)079[0315:EOMALN]2.0.CO;2
Bielmyer GK et al (2005) The effects of metals on embryo-larval and adult life stages of the sea urchin, Diadema antillarum. Aquat Toxicol 74:254–263. https://doi.org/10.1016/j.aquatox.2005.05.016
Boström-Einarsson L et al (2020) Coral restoration—a systematic review of current methods, successes, failures and future directions. PLoS ONE 15:e0226631. https://doi.org/10.1371/journal.pone.0226631
Brown MR et al (1997) Nutritional properties of microalgae for mariculture. Aquaculture 151(1–4):315–331. https://doi.org/10.1016/S0044-8486(96)01501-3
Brown MR, McCausland MA, Kowalski K (1998) The nutritional value of four Australian microalgal strains fed to Pacific oyster Crassostrea gigas spat. Aquaculture 165(3–4):281–293. https://doi.org/10.1016/S0044-8486(98)00256-7
Buitrago E et al (2005) Mass production of competent larvae of the sea urchin Lytechinus variegatus (Echinodermata: Echinoidea). Aquac Int 13(4):359–367. https://doi.org/10.1007/s10499-004-6551-y
Carboni S et al (2012) Effects of dietary microalgae on growth, survival and fatty acid composition of sea urchin Paracentrotus lividus throughout larval development Aquaculture 324:250–258. https://doi.org/10.1016/j.aquaculture.2011.10.037
Cárcamo PF, Candia AI, Chaparro OR (2005) Larval development and metamorphosis in the sea urchin Loxechinus albus (Echinodermata: Echinoidea): effects of diet type and feeding frequency. Aquaculture 249:375–386. https://doi.org/10.1016/j.aquaculture.2005.03.026
Castilla-Gavilán M et al (2018) Optimising microalgae diets in sea urchin Paracentrotus lividus larviculture to promote aquaculture diversification. Aquaculture 490:251–259. https://doi.org/10.1016/j.aquaculture.2018.02.003
Coutinho P et al (2020) Enriching rotifers with “Premium” microalgae: Rhodomonas lens. Mar Biotechnol 22(1):118–129. https://doi.org/10.1007/s10126-019-09936-4
Dayras P et al (2021) Microalgal diet influences the nutritive quality and reproductive investment of the cyclopoid copepod Paracyclopina nana. Front Mar Sci 8:69751. https://doi.org/10.3389/fmars.2021.697561
Dunstan GA, Brown MR, Volkman JK (2005) Cryptophyceae and Rhodophyceae; chemotaxonomy, phylogeny, and application. Phytochemistry 66(21):2557–2570. https://doi.org/10.1016/j.phytochem.2005.08.015
Eckert G (1998) Larval development, growth and morphology of the sea urchin Diadema antillarum. Bull Mar Sci 63:443–451
Enright CT et al (1986) Growth of juvenile Ostrea edulis L. fed Chaetoceros gracilis Schütt of varied chemical composition. J Exp Mar Biol Ecol 96(1):15–26. https://doi.org/10.1016/0022-0981(86)90010-9
Fenaux L (1982) Nutrition of larvae. In: Jangoux M, Lawrence JM (ed) Echinoderm nutrition, 1st edn. CRC Press, London, pp 479–489. https://doi.org/10.1201/9781003078920
Fernández RG (2001) Artemia bioencapsulation I. Effect of particle sizes on the filtering behavior of Artemia franciscana. J Crust Biol 21(2):435–442. https://doi.org/10.1163/20021975-99990144
Fernández-Reiriz MJ, Irisarri J, Labarta U (2015) Feeding behaviour and differential absorption of nutrients in mussel Mytilus galloprovincialis: responses to three microalgae diets. Aquaculture 446:42–47. https://doi.org/10.1016/j.aquaculture.2015.04.025
Glencross BD, Booth M, Allan GL (2007) A feed is only as good as its ingredients–a review of ingredient evaluation strategies for aquaculture feeds. Aquac Nutr 13(1):17–34. https://doi.org/10.1111/j.1365-2095.2007.00450.x
Gomes A et al (2021) Effects of single and mixed-diatom diets on growth, condition, and survival of larvae of the sea urchin Paracentrotus lividus (Lamarck, 1816). Aquac Int 29(3):1069–1090. https://doi.org/10.1007/s10499-021-00676-8
González-Araya R et al (2012) The selection of an ideal diet for Ostrea edulis (L.) broodstock conditioning (part B). Aquaculture 362:55–66. https://doi.org/10.1016/j.aquaculture.2012.06.029
Guedes AC, Malcata FX (2012) Nutritional value and uses of microalgae in aquaculture. In: Muchlisin ZA (ed) Aquaculture, 1st edn. InTech, Croatia, pp 59–78. https://doi.org/10.5772/30576
Harris LG, Eddy SD (2015) Sea urchin ecology and biology. In: Brown NP, Eddy SD (ed) Echinoderm aquaculture, 1st edn. Wiley & Sons, New Jersey, pp 1–24. https://doi.org/10.1002/9781119005810.ch1
Hernández JC et al (2006) Temporal patterns of larval settlement of Diadema antillarum in Canary Islands using an experimental larval collector. Bull Mar Sci 78:271–279
Hinegardner RT (1969) Growth and development of the laboratory cultured sea urchin. Biol Bull 137(3):465–475. https://doi.org/10.2307/1540168
Hodin J et al (2019) Culturing echinoderm larvae through metamorphosis. Methods Cell Biol 150:125–169. https://doi.org/10.1016/bs.mcb.2018.11.004
Hughes TP et al (2010) Rising to the challenge of sustaining coral reef resilience. Trends Ecol Evol 25:633–642. https://doi.org/10.1016/j.tree.2010.07.011
Jaris H, Brown DS, Proestou DA (2019) Assessing the contribution of aquaculture and restoration to wild oyster populations in a Rhode Island coastal lagoon. Conserv Genet 20(3):503–516. https://doi.org/10.1007/s10592-019-01153-9
Kelly MS et al (2000) Morphology and survivorship of larval Psammechinus miliaris (Gmelin) (Echinodermata: Echinoidea) in response to varying food quantity and quality. Aquaculture 183(3–4):223–240. https://doi.org/10.1016/S0044-8486(99)00296-3
Knuckey RM et al (2005) Development of an optimal microalgal diet for the culture of the calanoid copepod Acartia sinjiensis: effect of algal species and feed concentration on copepod development. Aquaculture 249(1–4):339–351. https://doi.org/10.1016/j.aquaculture.2005.02.053
Kreeger DA, Langdon CJ (1993) Effect of dietary protein content on growth of juvenile mussels, Mytilus trossulus (Gould 1850). Biol Bull 185(1):123–139. https://doi.org/10.2307/1542136
Lavens P, Sorgeloos P (1996) Manual on the production and use of live food for aquaculture (No. 361). Food and Agriculture Organization (FAO)
Leal MC et al (2016) Coral aquaculture: applying scientific knowledge to ex situ production. Rev Aquac 8:136–153. https://doi.org/10.1111/raq.12087
Leber K, et al (2009) Developing restoration methods to aid in recovery of a key herbivore, Diadema antillarum, on Florida coral reefs. Mote Marine Laboratory Technical Report No. 1347. https://dspace.mote.org/handle/2075/316
Leonardos N, Geider RJ (2004) Responses of elemental and biochemical composition of Chaetoceros muelleri to growth under varying light and nitrate: phosphate supply ratios and their influence on critical N: P. Limnol Oceanog 49(6):2105–2114. https://doi.org/10.4319/lo.2004.49.6.2105
Lessios HA (2016) The Great Diadema antillarum die-off: 30 years later. Ann Rev Mar Sci 8:267–283. https://doi.org/10.1146/annurev-marine-122414-033857
Lirman D, Schopmeyer S (2016) Ecological solutions to reef degradation: optimizing coral reef restoration in the Caribbean and Western Atlantic. PeerJ 4:e2597. https://doi.org/10.7717/peerj.2597
Liu H et al (2007) The effect of diet type on growth and fatty acid composition of the sea urchin larvae, II. Psammechinus miliaris (Gmelin). Aquaculture 264:263–278. https://doi.org/10.1016/j.aquaculture.2006.12.022
Lora-Vilchis MC, Cordero-Esquivel B, Voltolina D (2004) Growth of Artemia franciscana fed Isochrysis sp. and Chaetoceros muelleri during its early life stages. Aquac Res 35(11):1086–1091. https://doi.org/10.1111/j.1365-2109.2004.01123.x
Lorenzen K (2014) Understanding and managing enhancements: why fisheries scientists should care. J Fish Biol 85:1807–1829. https://doi.org/10.1111/jfb.12573
McBride SC (2005) Sea urchin aquaculture. Am Fish Soc Symp 46:179–208
Moe M (2014) Breeding the West Indian Sea egg: Tripneustes ventricosus. CORAL Magazine 11:80–94
Neilson BJ et al (2018) Herbivore biocontrol and manual removal successfully reduce invasive macroalgae on coral reefs. PeerJ 6:e5332. https://doi.org/10.7717/peerj.5332
Ogden J (1977) Carbonate-sediment production by parrot fish and sea urchins on Caribbean reefs: reef biota. Studies in Geology: Reefs and Related Carbonates-Ecology and Sedimentology 4:281–288
Ohs CL et al (2010) Evaluation of dietary microalgae for culture of the calanoid copepod Pseudodiaptomus pelagicus. Aquaculture 307(3–4):225–232. https://doi.org/10.1016/j.aquaculture.2010.07.016
Osinga R et al (2011) The biology and economics of coral growth. Mar Biotechnol 13:658–671. https://doi.org/10.1007/s10126-011-9382-7
Patterson JT (2019) The growing role of aquaculture in ecosystem restoration. Restor Ecol 27:938–941. https://doi.org/10.1111/rec.13002
Peltomaa E, Johnson MD, Taipale SJ (2018) Marine cryptophytes are great sources of EPA and DHA. Mar Drugs 16(1):3. https://doi.org/10.3390/md16010003
Pilnick AR et al (2021) A novel system for intensive Diadema antillarum propagation as a step towards population enhancement. Sci Rep 11(1):1–13. https://doi.org/10.1038/s41598-021-90564-1
R Core Team (2021) R: a language and environment for statistical computing. R Foundation for Statistical Computing, Vienna, Austria. URL https://www.R-project.org/
Rendleman AJ, Pace DA (2018) Physiology of growth in typical and transversus echinopluteus larvae. Invertebr Biol 137(4):289–307. https://doi.org/10.1111/ivb.12227
Rinkevich B (1995) Restoration strategies for coral reefs damaged by recreational activities: the use of sexual and asexual recruits. Restor Ecol 3:241–251. https://doi.org/10.1111/j.1526-100X.1995.tb00091.x
Sammarco PW (1982) Echinoid grazing as a structuring force in coral communities: whole reef manipulations. J Exp Mar Biol Ecol 61:31–55. https://doi.org/10.1016/0022-0981(82)90020-X
Schiopu D, George SB, Castell J (2006) Ingestion rates and dietary lipids affect growth and fatty acid composition of Dendraster excentricus larvae. J Exp Mar Biol Ecol 328(1):47–75. https://doi.org/10.1016/j.jembe.2005.06.019
Seixas P et al (2009) Nutritional value of the cryptophyte Rhodomonas lens for Artemia sp. J Exp Mar Biol Ecol 381:1–9. https://doi.org/10.1016/j.jembe.2009.09.007
Sorgeloos P et al (1998) Use of brine shrimp, Artemia spp., in larval crustacean nutrition: a review. Reviews Fish. Sci. 6(1–2):55–68. https://doi.org/10.1080/10641269891314195
Strathmann RR (1967) Estimating the organic carbon content of phytoplankton from cell volume or plasma volume. Limnol Oceanog 12(3):411–418. https://doi.org/10.4319/lo.1967.12.3.0411
Strathmann RR (1971) The feeding behavior of planktotrophic echinoderm larvae: mechanisms, regulation, and rates of suspension feeding. J Exp Mar Biol Ecol 6(2):109–160. https://doi.org/10.1016/0022-0981(71)90054-2
Strathmann RR, Jahn TL, Fonseca JR (1972) Suspension feeding by marine invertebrate larvae: clearance of particles by ciliated bands of a rotifer, pluteus, and trochophore. Biol Bull 142(3):505–519. https://doi.org/10.2307/1540326
Stumpp M et al (2013) Digestion in sea urchin larvae impaired under ocean acidification. Nature Clim Change 3(12):1044–1049. https://doi.org/10.1038/nclimate2028
Suckling CC, Terrey D, Davies AJ (2018) Optimising stocking density for the commercial cultivation of sea urchin larvae. Aquaculture 488:96–104. https://doi.org/10.1016/j.aquaculture.2018.01.022
Tanner CE, Parham T (2010) Growing Zostera marina (eelgrass) from seeds in land-based culture systems for use in restoration projects. Restor Ecol 18(4):527–537. https://doi.org/10.1111/j.1526-100X.2010.00693.x
Tremblay R et al (2007) Effect of Rhodomonas salina addition to a standard hatchery diet during the early ontogeny of the scallop Pecten maximus. Aquaculture 262(2–4):410–418. https://doi.org/10.1016/j.aquaculture.2006.10.009
Ware M et al (2020) Survivorship and growth in staghorn coral (Acropora cervicornis) outplanting projects in the Florida Keys National Marine Sanctuary. PLoS ONE 15:e0231817. https://doi.org/10.1371/journal.pone.0231817
Westbrook CE et al (2015) Survivorship and feeding preferences among size classes of outplanted sea urchins, Tripneustes gratilla, and possible use as biocontrol for invasive alien algae. PeerJ 3:e1235. https://doi.org/10.7717/peerj.1235
Funding
This work was supported by the National Oceanic and Atmospheric Administration [award number NA20NMF4630304]; the United States Department of Agriculture National Institute of Food and Agriculture [Hatch project number FLA-FOR-005902]; The Florida Aquarium; and the University of Florida. Supplemental support was provided by Florida Sea Grant. These sponsors had no role in study design; in the collection, analysis, and interpretation of data; in the writing of this report; or in the decision to submit this article for publication.
Author information
Authors and Affiliations
Contributions
Aaron R. Pilnick: conceptualization, formal analysis, investigation, data curation, writing — original draft, visualization. Keri L. O’Neil: conceptualization, resources, writing — review and editing, funding acquisition. Matthew A. DiMaggio: conceptualization, methodology, resources, writing — review and editing, supervision. Joshua T. Patterson: conceptualization, methodology, writing — review and editing, supervision, funding acquisition.
Corresponding author
Ethics declarations
Competing interests
The authors declare no competing interests.
Ethics approval
No specific approval of research ethics committees was required to accomplish the goals of this study because experimental work was conducted with an unregulated invertebrate species.
Additional information
Handling Editor: Gavin Burnell
Publisher's note
Springer Nature remains neutral with regard to jurisdictional claims in published maps and institutional affiliations.
Rights and permissions
Springer Nature or its licensor holds exclusive rights to this article under a publishing agreement with the author(s) or other rightsholder(s); author self-archiving of the accepted manuscript version of this article is solely governed by the terms of such publishing agreement and applicable law.
About this article
Cite this article
Pilnick, A.R., O’Neil, K.L., DiMaggio, M.A. et al. Development of larviculture protocols for the long-spined sea urchin (Diadema antillarum) and enhanced performance with diets containing the cryptophyte Rhodomonas lens. Aquacult Int 30, 3017–3034 (2022). https://doi.org/10.1007/s10499-022-00945-0
Received:
Accepted:
Published:
Issue Date:
DOI: https://doi.org/10.1007/s10499-022-00945-0