Abstract
Plant secondary metabolism evolved in the context of highly organized and differentiated cells and tissues, featuring massive chemical complexity operating under tight environmental, developmental and genetic control. Biotechnological demand for natural products has been continuously increasing because of their significant value and new applications, mainly as pharmaceuticals. Aseptic production systems of plant secondary metabolites have improved considerably, constituting an attractive tool for increased, stable and large-scale supply of valuable molecules. Surprisingly, to date, only a few examples including taxol, shikonin, berberine and artemisinin have emerged as success cases of commercial production using this strategy. The present review focuses on the main characteristics of plant specialized metabolism and their implications for current strategies used to produce secondary compounds in axenic cultivation systems. The search for consonance between plant secondary metabolism unique features and various in vitro culture systems, including cell, tissue, organ, and engineered cultures, as well as heterologous expression in microbial platforms, is discussed. Data to date strongly suggest that attaining full potential of these biotechnology production strategies requires being able to take advantage of plant specialized metabolism singularities for improved target molecule yields and for bypassing inherent difficulties in its rational manipulation.
Similar content being viewed by others
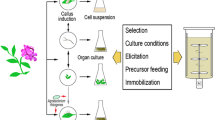
Avoid common mistakes on your manuscript.
Main Features of Plant Special Metabolites
Whereas plant primary metabolites are essential for immediate survival of all plants, being directly involved in growth, development and reproduction, secondary metabolites (natural products or specialized metabolites) play a major role in ecology and fitness of a species, often being restricted to certain taxa, hence the term specialized metabolites.
Despite progresses in synthetic chemistry, natural products and molecules derived or modeled from them provided 40% of all drugs approved between 1981 and 2014 [1]. Plants play a prominent role in the development of this pharmaceutical arsenal. The list of interesting secondary metabolites is even greater if we include those employed for non-medicinal purposes, such as chemical industry components, food additives, dyes, perfumes, cosmetics and nutraceuticals. Plant-derived pharmaceuticals are often expensive, due to difficult or non-economically viable chemical synthesis [2], hard purification from complex extracts, or presence in low concentrations, sometimes only in rare or endangered species.
The estimated number of secondary metabolites exceeds 200,000, with terpenes being the largest class (over 30,000), followed by alkaloids (over 20,000), all of which are produced by committed biosynthetic pathways [3]. In spite of the great number and diversity of structures, several features are common to most secondary metabolites (Fig. 1), including the generation of chemical diversity from core compounds through the action of modifying enzymes, such as hydroxylases, oxygenases, methyltransferases, and glycosyltransferases. The origins of secondary metabolites, both biosynthetic and evolutionary, stem from primary metabolism. All secondary metabolites can be traced back to a primary metabolite precursor, hence the term secondary, and the genes encoding proteins dedicated to secondary metabolism appear to have arisen from primary metabolism-related genes by duplication, mutations and subsequent diversification [4, 5]. The cooperation of two or more metabolic pathways is often found in the biosynthesis of secondary metabolites, such as flavonoids, which depend on both shikimic and malonic acid pathways to be assembled. Biosynthetic pathways leading to secondary metabolites can have steps expressed in different tissues or organs.
Biosynthesis of the alkaloid vinblastine requires tissues such as epidermis, parenchyma idioblasts, laticifers and vascular parenchyma [6]. A significant number of secondary metabolites is produced and/or stored in specialized cell types or structures, including glandular trichomes and resin ducts. This characteristic is particularly true for lipophilic metabolites, such as terpenes [7]. Different subcellular compartments are often involved in secondary metabolite biosynthesis. This is the case of bisindole alkaloids production, which requires the involvement of subcellular compartments as diverse as cytosol, vacuole, chloroplast, nucleus and endoplasmic reticulum [8]. Such complexity of anatomical and subcellular distribution of biochemical pathways adds another level of regulation to the flux of carbon toward secondary products, i.e. metabolite transporters [9]. In most cases, these transporters are of the ABC-type or H+-driven. In the subcellular context, the vacuole often functions as a storage compartment of hydrophilic secondary metabolites.
Plant secondary metabolites are frequently modulated by biotic and abiotic signals [10]. These include both herbivore and pathogen attacks, as well exposure to drought, flooding, extreme temperatures, excess irradiance, UV stress, salinity, and mineral imbalance. Phytohormones and signaling molecules that mediate adaptive responses to these challenges are also capable of triggering the accumulation of secondary metabolites. Notable among these are jasmonic acid and its methyl ester methyl jasmonate, salicylic acid, ethylene, abscisic acid and nitric oxide. Accumulation of plant secondary metabolites can be regulated by development and light [11], as well as by the circadian clock [12].
Biotic and abiotic stresses generate reactive oxygen and nitrogen species (ROS and NOS), which trigger secondary metabolite production, accumulation and release. ROS signaling of secondary metabolism is often a hub in stress responses [13]. Since several secondary metabolites have significant antioxidant properties, their induction by ROS may also contribute to mitigate the damaging effect of these chemical species on cellular structures and to control their distribution [14, 15].
Patterns of secondary metabolite accumulation may be classified in three major types: constitutive, preformed, and inducible [4]. Constitutively accumulated metabolites are present as basal chemical constituents that often have defense activity against pathogens [16] and herbivores. This may be the case of phenolic acids and flavonols. The preformed accumulation often involves vacuolar storage in non-toxic forms that upon physical damage imposed by herbivores or necrotrophic pathogens, for example, are released and modified to become bioactive. This is the case of glucosinolates and cyanogenic glucosides, which are stored in vacuoles and, when released, may form isothiocyanates and cyanide, respectively. Inducible metabolites are absent or present in low concentrations prior to stress events when their biosynthesis is activated ensuing accumulation, as is the case of several terpenes and alkaloids. Many secondary metabolites display combined strategies of accumulation to various extents [15].
Enzymes of secondary metabolism are frequently arranged in metabolic channels or metabolons, physical associations of enzymes organized in membranes of various cellular compartments. The formation of metabolons is a dynamic process, generally started by transmembrane enzymes that act as organizers. As shown for the cyanogenic glucoside dhurrin of sorghum, the endoplasmic reticulum membrane itself may be part of the metabolon and its local composition and charge may modulate the channel complex activity [17]. These protein-membrane arrangements can also be dismantled and later re-organized, depending on cell demands. Several advantages are attributed to metabolons, including acceleration of biochemical reactions, reduced dilution of metabolites, improved flux control coefficient of pathways, easier metabolic flow of relatively insoluble or unstable intermediates, less undesired effects of toxic intermediates, facilitated allocation of metabolites to appropriate storage compartments, and improved metabolic cross-talk and redirection [18].
Physical organization in close proximity is not only observed for enzymes involved in plant secondary metabolism, but it is also found at the genome level. Clusters of related genes involved in biosynthesis of several classes of secondary metabolites (terpenes, alkaloids, benzoxazinoids and cyanogenic glucosides) resembling prokaryotic operons have been described [19]. Secondary metabolism gene clusters most likely originated from primary metabolism genes [20]. Clusters include regulatory sequences, promoters, coding sequences, and intergenic regions, making possible the design of minimum regulatory clusters [21]. Transcription factors controlling gene clusters can lie outside their structure. Cluster arrangement may facilitate co-expression, coinheritance, assembly of metabolic channels, and regulatory chromatin changes [20].
The complexity and peculiar features of secondary metabolite biosynthesis and homeostasis as outlined above make producing them in reproducible, controlled and scalable fashion a hard task. Nonetheless, there are a number of cultivation strategies to obtain metabolites of interest, using wild types or genetically modified versions of whole plants, axenic cultures of cells, organs and microorganisms expressing plant genes, as well as chemical syntheses or semi-synthesis. In fact, although cell cultures can present low yields, genetic and biochemical instability [22], there are notable cases of success, such as the production of taxol, ginsenosides, and the red dye shikonin. Next, basic aspects affecting aseptic biological production systems of natural products, including recent key examples of synthetic biology strategies, will be discussed. Techniques that properly consider specific features of secondary metabolism, or are even designed to take advantage from them, may provide important solutions for producing compounds of economic interest.
Aseptic Cultivation Based Solutions for Producing Bioactive Metabolites
Under the right set of conditions, plant cultivation in field or greenhouse environment can be advantageous over axenic production systems, particularly when compound market value is not sufficiently high to make the latter approach commercially attractive. However, extraction from plants has some drawbacks, such as climate dependence, susceptibility to pathogens, poor adaptation to grow in dense monocultures, specific requirements of fertilizers and agrochemicals, logistics, and regulatory issues for field deployment of genetically modified plants. Wild plant genetic heterogeneity can impact on product quality and the need for extensive purification steps to obtain the active molecules from largely impure extracts increases costs and chemical residues.
A range of complementary solutions for the production of bioactive metabolites is available [23, 24]. The techniques range from in vitro callus and cell suspension cultures, immobilized cells, root and shoot cultures, hairy roots, whole plant liquid cultures, micropropagation techniques, and plant cell catalyzed biotransformation. These strategies may involve genetically transformed cell lines with engineered metabolic pathways in homologous or heterologous plant species, as well as microorganisms, which facilitate production scale-up (Fig. 2). Cell suspension cultures and immobilized cells can also be used to produce target compounds. Cell cultures have an unlimited potential for growth and plant regeneration.
Plant Cell Cultures
Suspension cultures are fast growing and amenable to continuous culturing in a chemostat vessel. Plant cells are biosynthetically totipotent for each cell is potentially able to produce the range of chemicals found in the parent plant. Plant cell culture has three main uses: production of secondary metabolites, biomass generation, and basic studies [25,26,27]. Cell cultures are a continuous source of natural products [25, 28, 29]. Growth conditions, e.g., temperature, pH, light quality and quantity, phytohormones, aeration, agitation, immobilization, as well as the medium composition, can be manipulated in order to optimize the production of the desired metabolites [25, 30,31,32,33].
Several secondary metabolites accumulate during stationary phase of growth. At this stage, carbon allocation for primary metabolism is reduced, so secondary compounds are more actively synthesized. It has been frequently observed that many new enzymatic activities appear during stationary phase, suggesting biochemical differentiation of cells [34]. In cell cultures, secondary metabolites can be produced under controlled conditions. High yielding cell selection, product harvest, validation, and extraction are facilitated and less costly. Cell banks may be preserved at low temperatures for long periods and genetically modified cells can be grown in a contained environment. Some metabolites in plant cell cultures may accumulate at higher titer in cell cultures compared to the parent plants [35], and cell cultures may also be a source of new chemical diversity.
Undifferentiated cultures can undergo significant somaclonal variation, usually after several subculture cycles, leading to variable production of secondary metabolites. After a certain period of time (ranging from weeks to years), genetic stability is often achieved and the culture can be regarded as homogeneous [34]. On the other hand, somaclonal variation may be a useful source of variability to explore in terms of biosynthetic capacity. However, plant cell cultures frequently yield low quantities of target metabolites and entail high costs. These constraints are of less importance if the target metabolite has a high market value, and if the costs of culture media, precursors and elicitors are kept low. Often some basic conditions need to be achieved: high yields of compound(s), high growth rates, and amenability to scale up.
An emblematic case is taxol (paclitaxel) production by Taxus cell cultures (Fig. 3). Taxol is considered one of the most important anticancer drugs of the last few decades. Its antitumor activity is due to microtubule stabilization [36]. Production of taxol by calli, immobilized and suspension cells of T. cuspidata and T. canadensis in completely defined media showed the feasibility of cell culture as source of this drug [37]. Cultures of other Taxus species were also shown to produce taxol, while further improvement of taxol yield from cell cultures was achieved by precursor feeding (e.g. phenylalanine) and jasmonate elicitation [38, 39]. Additional key improvements were reached by employing a two-stage culture system, elicitation with MeJA, immobilization in alginate beads and scaling up in stirred bioreactor [40, 41]. Taxus media cells have been engineered to express taxadiene synthase gene, encoding the enzyme catalyzing the first committed step in taxol biosynthesis, yielding higher taxane production [42]. Currently, cell culture brought to industrial scale is one of the major commercial sources of high quality taxol (http://www.phytonbiotech.com/ access October 16th, 2017).
On the other hand, as a result of the presence of high concentration of carbohydrates and auxins in culture medium, cells show relatively fast growth rates, but low expression of secondary metabolic pathways. This common condition may be a function of various factors, such as competition for common precursors, inadequate metabolite storage in vacuoles, poor compartmentation of enzyme and intermediates, nonfunctional plastids, low expression of enzymes and regulatory proteins [15, 43]. Problems in the normal function of small RNAs [44] and selective proteolysis systems may also contribute to the unfavorable biochemical phenotype that leads to low yields of metabolites of interest in some cell cultures. As discussed in previous sections, considering the strong dependence of secondary pathways on subcellular compartments, different cell types, tissues and even organs to fully operate in plants, it is not surprising that relatively disorganized cultures often fall short of providing attractive yields of target molecules. To overcome or alleviate this constraint, a number of strategies can be implemented. Essentially, these approaches have explored the responsiveness of secondary metabolism to specific environmental stimuli and associated signaling molecules, dependence on primary metabolite precursors, frequent inverse relationship with growth, and partial recovery of tissue-like condition.
Common Strategies to Increase Secondary Metabolite Production in Plant Cell Cultures
Selection and optimization of cell lines may increase the productivity of a given process by several orders of magnitude. Cell line selection for callus induction to obtain high-producing lines can begin with the choice of an elite parent plant. Cell cultures are heterogeneous populations with different physiology, requiring screening for variant cell clones containing the highest levels of desired product. This has been exploited with cinnamic acid derivatives, anthraquinones, berberines, shikonins and anthocyanins [25, 28]. An inverse relationship between mean cell aggregate size and taxol yield has been described, pointing out to the potential importance of this parameter in developing elite cell cultures [45]. On the other hand, immobilized cultures may create a pseudo-tissue condition, in which a certain degree of differentiation is recovered and new molecule gradients are established. Mutation strategies have been employed in order to obtain cells overproducing secondary metabolites. Selective agents (such as p-fluorophenylalanine, 5-methyltryptophan, glyphosate and biotin) have been tested to select high yielding cell lines. In this method, a large population of cells is exposed to sublethal doses of a toxic (or cytotoxic) metabolic inhibitor or an environmental stress, selecting resistant cells, which may overproduce target molecules or their precursors [25, 46].
Media optimization determines the appropriate amounts and ratios of key nutrients, which affect the supply of precursors and energy, playing a crucial role in production of secondary metabolites [47]. The common dichotomy between growth and secondary metabolism led to two-stage culture systems, involving an initial growth stage, to generate a large amount of biomass and a second stage, when the media is depleted of growth stimulants such as nitrate, phosphate and growth regulators, while sometimes also increasing carbon supply. Metabolite accumulation can be modulated by the concentration and nature of the inorganic and organic components and phytohormones [27, 28].
Supplying a precursor compound (preferably cheap and available) of a secondary metabolite biosynthetic pathway can increase the yield of the final product. Higher production of secondary metabolites upon supplying precursor or intermediate compounds has been frequently noted. Feeding l-phenylalanine or benzoic acid to Taxus suspension cultures, for example, increased taxol yield; supplying the same amino acid improved anthocyanin yield in suspensions of strawberry [48, 49].
Contents of secondary metabolites fluctuate during development (e.g., [50, 51]). Regulation of secondary metabolic pathways is considered an integral part of plant development [52]. An ‘elicitor’ may be defined as a substance initiating or improving biosynthesis of specific compound(s) when introduced in small concentrations. Elicitors can be classified on the basis of their ‘nature’ as abiotic or biotic, and on the basis of their ‘origin’ as exogenous or endogenous.
Production of secondary metabolites can be enhanced by the treatment of undifferentiated cells with abiotic or biotic elicitors. Stress factors, such as osmotic shock, heavy metal ions, inorganic salts, methyl jasmonate, salicylic acid, nitric oxide, chitosan, chitin and bacterial, fungal and yeast homogenates, cyclodextrins, temperature changes or UV and visible radiation, may enhance secondary metabolite accumulation [53,54,55,56]. Elicitation was effective, for example, in stimulating production of taxol by Taxus cell suspension cultures, ajmalicine by Catharanthus roseus, and tropane alkaloids by suspension cultures of Datura stramonium. This strategy, in conjunction with end-product removal and accumulation in an extractive phase, has proven to be very successful for increasing yields. Two-phase operation with elicitation-enhanced alkaloid production in cell suspension cultures of Eschscholzia californica was also developed [35]. Jasmonic acid, its active conjugate jasmonoyl-isoleucine, and related molecules have proven to be of particular importance in the elicitation of most secondary metabolites studied so far [57], suggesting it may be a signaling hub in transduction pathways leading to activation of specialized metabolism.
Many interesting compounds are produced in roots or shoots, but not in suspension cultures, e.g., hyoscyamine and digoxin, respectively, wherein a certain degree of differentiation is necessary for the expression of the appropriate biosynthetic pathway. As discussed above, the need for specialized tissues and cells with particular cellular environments and subcellular differentiation, coordinated transport of intermediates and split-pathway expression patterns, are common features of secondary metabolism. When biosynthesis of a certain compound does not occur in cell culture, use of shoot, root or whole plant cultures may be an alternative.
Production of Secondary Metabolites in Differentiated Cultures
Differentiated cultures are genetically more stable than cell cultures. Hyoscyamine and scopolamine are obtained from root cultures, whereas essential oils, morphinan alkaloids of Papaver somniferum and sesquiterpene lactones (artemisinin) of Artemisia annua, in shoot cultures [27, 53, 55, 58]. Valepotriates from Valeriana glechomifolia have been successfully produced by whole plant liquid cultures in floating rafts [59].
Adventitious root cultures, normally induced by phytohormones in medium (in many cases by high auxin concentrations), are amenable to biotechnological applications due to availability of root-specific bioreactors, possibility of becoming autotrophic (through manipulation of light, CO2, and carbohydrate supply), and a natural capacity of secreting metabolites. Production of secondary metabolites from adventitious root cultures involves various stages, resembling those of cell cultures. Adventitious root cultures have been successfully used for producing essentially all classes of secondary metabolites. Medium optimization, elicitation and precursor feeding have been used for enhancing metabolite yields in adventitious root cultures of Bupleurum falcatum, Echinacea spp., Hyoscyamus niger, Panax ginseng, Scopolia parviflora, Withania somnifera, and Polygonum multiflorum [47, 60].
Shoots exhibit some properties comparable to roots, namely genetic stability, good competence for secondary metabolism, and possibility of positive growth-production correlation. Shoots and somatic embryos may be grown in bioreactors for metabolite production and/or clonal mass propagation. However, there are some differences in the metabolic pattern, as some pathways are specifically expressed in either roots or shoots. Other differences concern a somewhat slower growth rate (fastest doubling time circa 3 days) and light requirements [61,62,63].
Further improvement of target metabolite yields either in cell cultures or in differentiated cultures can be attained by genetic modification. This strategy may also be used to overcome limitations of production of specific culture systems or species. Metabolic engineering may focus on biosynthetic, transporter or regulatory genes using generic or tissue/cell-specific promoter sequences. Such spatial specificity may improve target metabolite yields by mimicking the natural distribution conditions of secondary metabolic pathways in whole plants and differentiated cells, potentially optimizing enzyme activity by providing appropriate microenvironmental conditions and cofactor/activator supply.
Genetically Modified Cultures for the Production of Bioactive Compounds
The complex and puzzling molecular/biochemical/cellular regulatory scenario of secondary metabolism poses a formidable challenge for metabolic engineers, particularly in the case of cell cultures. Nevertheless, there are examples of successful modification of secondary metabolic pathways, leading to desired metabolic profiles [64]. Overall, there seems to be no single recipe for success, the degree of which may vary on an individual basis. Some strategies, however, appear to be more likely to yield the expected results.
The interruption of the expression of end portions of pathways through the use of antisense or RNAi technology has been applied in an effort to increase the accumulation of upstream metabolites. Insertion of single reaction steps or short side pathways that draw on abundant intermediates of the original pathway or a combination of the first two strategies just outlined may also be useful. Particular attention was given to the adequate supply of enzyme cofactors and systems for their regeneration, so increased enzyme activity is not compromised at the post-translational level [65]. The introduction of feedback insensitive forms of enzymes with significant input on metabolic flux control has been effective for increasing the yields of some metabolites of early parts of pathway(s). Similarly, the introduction of transporters to shuttle final metabolites into the vacuole, causing a relief of feedback inhibition, can be a means to improve metabolite yield.
One of the most promising approaches to engineer secondary metabolic pathways has been based on the modification of expression of transcription factors that control biosynthetic enzyme genes. In theory, this ensures a relatively coordinated and almost ‘stoichiometric’ production of enzymes, allowing for optimized biosynthesis. The efficiency of this approach is justified by the systemic nature of biochemical pathways (metabolic flux control) [66]. The introduction of novel secondary pathways in host cells or plants is often effective, suggesting that primary metabolite precursors may not be rate limiting. The expression of Arabidopsis genes leading to benzylglucosinolates in tobacco plants was successfully accomplished by employing USER™ Cloning and USER™ Fusion, a versatile ligation-free technique based on uracil excision that allows cloning of large fragments of DNA (up to a few kb) [67, 68].
Various transcription factors regulating the expression of genes encoding secondary metabolism enzymes are modulated by jasmonate. Examples include ORCA (terpenoid indole alkaloids), ERF (nicotine and artemisinin), MYC2 (indole glucosinolates, alkaloids and anthocyanins), MYB (phenylpropanoids, aliphatic glucosinolates), WRKY (terpenes and alkaloids) [69]. Not surprisingly, jasmonate and its derivatives are some of the most powerful and widely used stimulators of secondary metabolite production in biotechnological applications. The genetic manipulation of the jasmonate signaling pathway in plants may lead to new insights on the production of valuable metabolites.
Because of the complex morphology of plant cells and tissues, compartmentalization must be taken into account in attempts to modify metabolic pathways. Plant cell function is the result of elaborate interactions involving the genomes of chloroplasts, mitochondria and nucleus [70]. As it has been established for flavonoid and monoterpene indole alkaloid metabolism, portions of secondary pathways can be distributed between cytosol, endoplasmic reticulum, chloroplast, vacuole, and nucleus [71, 72]. Effective modification of secondary metabolic pathways demands targeting of introduced genes to the correct compartment. This ensures the adequate cell microenvironment for enzyme activity and promotes correct balance of precursors and products in the pathway [73]. Hence, constructs for gene introduction should consider sequences encoding target peptides.
The use of chloroplast genomes to express introduced genes can be advantageous to achieve higher expression levels. Since chloroplasts are abundant in several cell types, particularly in leaf mesophyll tissues, and each chloroplast has multiple copies of its genome, the effect of the overall copy number of introduced genes per cell after transplastome selection becomes relevant. In addition, as a rule, chloroplast genomes are of typical prokaryotic structure, made of circular double stranded sequences with very constant gene composition and relative positioning, allowing easy integration in specific regions by homologous recombination, as well as the introduction of gene clusters or synthetic multigene operons [74]. Modifications introduced in the nuclear genome, on the other hand, are not so readily directed to specific positions. However, significant progress in site specific gene insertion/modification in the nuclear genome can be obtained by CRISPR/Cas technology, which takes advantage of components of the DNA repairing machinery [75].
Cell, tissue and organ specific promoters can be used to control metabolism through gene expression in specific locations, thereby avoiding pitfalls of ectopic expression and metabolic energy waste. Terminally differentiated cell-specific promoters associated with glandular trichomes, secretory resin ducts, root hairs and stomata guard cells are potential tools to direct expression to certain cells only. Root, shoot, fruit, seed, and flower-specific promoters are also available from various well characterized genes, as well as tissue specific promoters, such as vascular tissue-related, for example. Various databases and software packages are available for analyzing plant promoter sequences to find conserved cis elements and transcription factor binding sites, including combinatorial cis-regulatory regions [76]. Temporally regulated expression may be attained by using promoters associated with developmental phases, such as senescence, flowering, fruit maturation, and promoter elements of clock regulated genes, e.g., morning or evening specific cis elements for gene expression [77,78,79,80].
Alternatives exist for inducible promoters, such as responsiveness to chemicals (e.g., ethanol or pathogen elicitors), light or phytohormone responsive elements. The introduction of appropriate responsive elements in the transgene promoter construct may render the expression sensitive to drought, salinity, osmotic, UV exposure and other abiotic stresses. These are conditions that often lead to secondary metabolite accumulation [81, 82].
Combined overexpression of biosynthetic genes regulated by glucocorticoid-inducible promoter increased production of various monomeric indole alkaloids; however, single gene overexpression induced some monomeric indole alkaloids, while inhibiting the accumulation of others. These data indicate the need for overexpressing multiple genes to enhance metabolic flux toward late products of the pathway [83].
Overexpression of the transcription factor ORCA3, which regulates the expression of various monoterpene indole alkaloid biosynthetic genes, and of the terpene moiety-related enzyme geraniol 10-hydroxylase (G10H), whose transcription is not regulated by ORCA3, was attempted to improve accumulation of dimeric indole (bisindole) alkaloids in hairy roots and plants of Catharanthus roseus [84]. In cell cultures, overexpression of ORCA3 leads to an increase in total alkaloids, but, due to lack of production of vindoline, no dimeric alkaloids, such as vinblastine, accumulated. This result probably reflected the need for tissue differentiation to achieve full biosynthesis.
Monomeric catharanthine and vindoline are coupled to form antitumor dimeric alkaloids. Catharanthine is secreted to the leaf surface, apparently for defense against pathogens, whereas vindoline would be released from cells upon herbivore damage, then allowing dimeric alkaloid biosynthesis. Viral-induced gene silencing of an epidermal ATP-binding cassete catharanthine transporter (CrTPT2) of Catharanthus plants increased intracellular contents of catharanthine, thereby leading to higher concentration of dimeric alkaloids of pharmaceutical value in leaves [85]. Engineering plant secondary metabolite transporters may become a valuable technology for improving yields, including in heterologous microbial platforms [86].
Clearly, the tool kit to genetically engineer secondary metabolism pathways leading to valuable metabolites is continuously expanding and improving. Among the simplest and most widely used genetically altered cultures to produce bioactive secondary metabolites are hairy roots.
Features of Hairy Roots
Hairy roots, also called transformed roots, are formed as a result of infection with Agrobacterium rhizogenes, a soil borne bacterium responsible for the hairy root disease in a number of dicots [87]. Hairy roots show extensive branching and rapid plagiotropic growth on hormone-free medium [88]. Hairy root cultures show similar or even higher biosynthetic capacity of secondary metabolite production compared to mother plants, as well as higher genetic and biochemical stability compared to conventional root cultures [89]. Hairy roots have been used for obtaining stable transgenic plants, large-scale production of bioactive compounds, plant regeneration and functional analysis of genes, to study plant-pathogen and plant-symbiont interactions, expression of recombinant proteins, and phytoremediation [61, 90,91,92,93,94,95,96]. Hairy roots are widely used to investigate regulation of plant secondary metabolism in organized cultures. Metabolic engineering tools have made hairy root cultures amenable to introduction of transgenes and thus to genetic modification of their metabolic pathways [97, 98].
Hairy root cultures have been used for the production of ajmalicine in Catharanthus roseus [99], ginsenosides in Panax ginseng [100], tropane alkaloids in Datura metel, Duboisia sp. and Hyoscyamus sp. [101], withanolides in Withania coagulans [102], and artemisinin of Artemisia annua [103]. In this last study, a combined cultivation in submerged liquid phase growth stage followed by gas-phase step in nutrient mist reactor was the most effective of three bioreactor types tested. Sesquiterpene production in this set up could have been influenced by the inherent stress conditions of liquid to gas-phase shift. Ginsenoside production in hairy root cultures of Panax ginseng was successfully scaled up to bioreactor level, achieving productivity almost 2-fold higher than in small flasks [104].
The in vitro transformation of plant material with A. rhizogenes involves chemotactism of Agrobacterium toward the plant cells, binding of bacteria to the surface components of the cell wall, activation of virulence (vir) genes, transfer and integration of the transfer-DNA (T-DNA) into the plant genome. T-DNA of Ri plasmid contains multiple open reading frames (ORFs) [105]. As a result of transformation, the metabolism of plant cells is changed and hairy roots are formed.
Several factors can affect efficiency of transformation to develop hairy roots. Both plant and bacterial aspects play roles in the process. Bacterial factors include A. rhizogenes strain, culture medium and selection of antibiotic for elimination after co-cultivation. Plant factors involve species, ecotypes, explant type, developmental stage, and growth rate. To attain optimum results of transformation, both microorganism and plant factors must be evaluated on a case-by-case basis [106, 107].
In several plant species, higher amounts of bioactive compounds in hairy roots have been reported when compared to the mother plants. Moreover, progress in scale-up of hairy root cultures for biomass and secondary metabolites production has made this system a useful tool for industrial applications [108, 109].
T-DNA of A. rhizogenes is known to activate the synthesis of secondary metabolites in transformed plant cells [110]. The potential of hairy roots for production of various bioactive compounds has been well documented. A. rhizogenes mediated transformation of plants may be used similarly to A. tumefaciens methods. However, hairy roots originate from single cells and are comprised only of transformed cells, whereas tumors induced by A. tumefaciens encompass a chimera of transformed and non-transformed cells.
Roots of Atropa belladonna engineered with A. rhizogenes carrying 6-hydroxylase gene of Hyoscyamus muticus were observed to produce five-fold higher concentration of scopolamine [98]. Hairy roots induced by genetic transformation of A. rhizogenes or its regenerated plants have been successfully used for the production of several pharmaceutical or aromatic compounds. Rol genes from A. rhizogenes proved to be potential activators of secondary metabolism in transformed cells, possibly by turning on defense genes [93, 111].
In spite of its regulatory complexity, plant specialized metabolism can be engineered in host organisms such as other plant species, bacteria and yeast. Expression in heterologous plant species is somewhat facilitated by the presence of several primary metabolism pathways to support specialized target molecules, as well as by well-established transformation protocols for model systems. On the other hand, limitations may arise from the lack of terminally differentiated cell types required for proper biosynthesis and end-product storage, such as secretory ducts and glandular trichomes.
From a biotechnological perspective, the expression of plant specialized metabolites in microbes is a great asset since it provides a simplified platform for scale up and mass production. Although at first it may seem unlikely to succeed, the transfer of plant secondary metabolic pathways to bacteria and yeast is not that far from the cellular scenario in which this particular type of metabolism evolved. The presence of gene clusters resembles the physical organization of bacterial operons, yeast metabolons are essentially similar to those of plants, and so is the presence of vacuoles, key cellular compartments shared by fungi and plants. Combining the expression of transporters, the use of appropriate inducible promoters and metabolic inhibitors, synthetic biology for producing important classes of plant secondary metabolites has been successfully accomplished in bacteria and yeast. Next, a brief description of some representative examples of ingenious ways to produce special plant metabolites in microbes is presented.
Microbial Platforms for Expressing Plant Pathways
Metabolic engineering can reconstitute plant metabolic pathways in heterologous systems, mostly bacteria, yeasts or other plant species. In the first two cases, a simpler, robust and amenable to scale up organism is sought, whereas in the case of other plant species, easier cultivation and genetic transformation, as well as faster growth, are the driving reasons for applying this strategy. In heterologous plant species, common early precursors may be readily available, often simplifying matters. Microbial production of secondary metabolites of interest may facilitate purification processes, since there are relatively less contaminating compounds compared to plant cells. Although cost-effectiveness to produce fine biochemicals using plants is attractive, demanding basically sunlight, carbon dioxide, water and soil, microbial cultures may be grown using as nutrient sources low-cost abundant feedstock biomass.
Structure and physico-chemical properties of the product of interest, host codon usage preference, requirements for post-translational modifications in biosynthetic or regulatory proteins (glycosylation, farnesylation), precursor availability, requirements for subcellular compartments, and effective strategies for host genetic transformation are some relevant aspects to consider in selecting a microbial platform to express plant pathways [65]. The selection of an appropriate microbial host may also depend on the desired strategy of engineering [64]. Pre-existing pathways present in the microbe may be modified to accommodate parts of plant metabolic catalytic steps or an entire new pathway may be introduced. Exogenous precursor supply may further stimulate activity of introduced pathways. Host strain optimization is often a crucial aspect to achieve higher yields. Combined inhibition of competitive endogenous pathways and expression of plant genes encoding target metabolite biosynthesis in regulated fashion by means of inducible promoters is an interesting strategy. Production of artemisinic acid at 25 g/L in yeast and semi-synthesis of the antimalaric chemotherapeutic artemisinin used this approach [112]. Expression of enzymes of different sources (plants, fungi, animals and bacteria) and improvement of catalytic activity by enzyme engineering may also be useful in yeast hosts [113].
In any of the scenarios just outlined, a demand for general precursors is often present. To minimize this bottleneck, the catalytic steps leading to general precursors in higher demand may be duplicated or specific enzymes with higher input in the metabolic flux control can be overexpressed. Extra copies of enzymes may be introduced with modifications so as to operate in a different subcellular context (e.g. conventional membrane bound and simplified soluble form) [114].
Examples of molecules of interest produced in engineered microbes include taxadiene (taxol precursor) [115], artemisinic acid (artemisinin precursor) [112], S-scoulerine (berberine precursor), 8-hydroxy-cadinene (gossypol precursor), resveratrol [116], and opioids, such as thebaine and hydrocodone [113]. Ingenious strategies have been used to introduce biosynthetic gene clusters using episomal type expression vectors in microorganisms. A combination of SOE-PCR (Splicing by Overlap Extension Polymerase Chain Reaction) and in vitro homologous recombination was used for the expression of early steps of taxol biosynthesis in yeast [115].
Cell-free systems have also been considered as alternatives for expressing biochemical pathways leading to metabolites of interest. Although in relatively early stage of development for plant secondary metabolites, these systems rely essentially on matrix immobilized enzymes, scaffold protein co-expressed pathways optimized for enzyme titer, or artificial cell-type structures, such as lipid and foam vesicles [114]. In line with these strategies, the use of ionic liquids for maintaining immobilized or free enzymes or, depending on toxicity levels, for cultivating plant or microbial cells, may also prove useful [117].
Conclusion
Plant aseptic cultures are complementary tools for the production of bioactive and other industrially relevant metabolites. Several approaches are being combined to yield interesting target compounds, in some cases successfully at industrial level. Rather than focusing on a specific biosynthetic pathway, the systemic nature and specific features of secondary metabolism must be considered (Fig. 4). Adequately addressing and taking advantage of the singularities of plant secondary metabolism, effectively integrating physiological, biochemical, molecular, and synthetic biology approaches, will be instrumental in making aseptic cultures highly attractive technologies for producing plant secondary metabolites.
References
Newman, D. J., & Cragg, G. M. (2016). Natural products as sources of new drugs from 1981 to 2014. Journal of Natural Products, 79, 629–661.
Urabe, D., Asaba, T., & Inoue, M. (2015). Convergent strategies in total syntheses of complex terpenoids. Chemical Reviews, 115, 9207–9231.
Kutchan, T., Gershenzon, J., Moller, B. L., & Gang, D. (2015). Natural products. In B. B. Buchanan, W. Gruissem, & R. L. Jones (Eds.), Biochemistry and molecular biology of plants (pp. 1132–1206). Oxford: Wiley.
Hartmann, T. (2007). From waste products to ecochemicals: Fifty years research of plant secondary metabolism. Phytochemistry, 68, 2831–2846.
Ober, D. (2010). Gene duplications and the time thereafter: Examples from plant secondary metabolism. Plant Biology, 12, 570–577.
De Luca, V., Salim, V., Thamm, A., Masada, S. A., & Yu, F. (2014). Making iridoids/secoiridoids and monoterpenoid indole alkaloids: Progress on pathway elucidation. Current Opinion in Plant Biology, 19, 35–42.
Nagegowda, D. A. (2010). Plant volatile terpenoid metabolism: biosynthetic genes, transcriptional regulation and subcellular compartmentation. FEBS Letters, 584, 2965–2973.
Courdavault, V., Papon, N., Clastre, M., Giglioli-Guivarc’h, N., St-Pierre, B., & Burlat, V. (2014). A look inside an alkaloid multisite plant: The Catharanthus logistics. Current Opinion in Plant Biology, 19, 43–50.
Nour-Eldin, H. H., & Halkier, B. A. (2013). The emerging field of transport engineering of plant specialized metabolites. Current Opinion in Biotechnology, 24, 263–270.
Ramakrishna, A., & Ravishankar, G. A. (2011). Influence of abiotic stress signals on secondary metabolites in plants. Plant Signaling and Behavior, 6, 1720–1731.
Matsuura, H. N., Fragoso, V., Paranhos, J. T., Rau, M. R., & Fett-Neto, A. G. (2016). The bioactive monoterpene indole alkaloid N, β-D-glucopyranosyl vincosamide is regulated by irradiance quality and development in Psychotria leiocarpa. Industrial Crops and Products, 86, 210–218.
Kooke, R., & Keurentjes, J. J. (2012). Multi-dimensional regulation of metabolic networks shaping plant development and performance. Journal of Experimental Botany, 63, 3353–3365.
Sewelam, N., Kazan, K., & Schenk, P. M. (2016). Global plant stress signaling: Reactive oxygen species at the cross-road. Frontiers in Plant Science, 7, 187.
Matsuura, H. N., & Fett-Neto, A. G. (2013). The major indole alkaloid N,β-D-glucopyranosyl vincosamide from leaves of Psychotria leiocarpa Cham. & Schltdl. is not an antifeedant but shows broad antioxidant activity. Natural Product Research, 27, 402–411.
Matsuura, H. N., Rau, M. R., & Fett-Neto, A. G. (2014). Oxidative stress and production of bioactive monoterpene indole alkaloids: Biotechnological implications. Biotechnology Letters, 36, 191–200.
Pedras, M. S., & Yaya, E. E. (2015). Plant chemical defenses: Are all constitutive antimicrobial metabolites phytoanticipins? Natural Products Communications, 10, 209–218.
Laursen, T., Borch, J., Knudsen, C., Bavishi, K., Torta, F., Martens, H. J., et al. (2016). Characterization of a dynamic metabolon producing the defense compound dhurrin in sorghum. Science, 354, 890–893.
Jørgensen, K., Rasmussen, A. V., Morant, M., Nielsen, A. H., Bjarnholt, N., Zagrobelny, M., et al. (2005). Metabolon formation and metabolic channeling in the biosynthesis of plant natural products. Current Opinion in Plant Biology, 8, 280–291.
Boycheva, S., Daviet, L., Wolfender, J. L., & Fitzpatrick, T. B. (2014). The rise of operon-like gene clusters in plants. Trends in Plant Science, 19, 447–459.
Osbourn, A. (2010). Secondary metabolic gene clusters: evolutionary toolkits for chemical innovation. Trends in Genetics, 26, 449–457.
Nützmann, H.-W., & Osbourn, A. (2014). Gene clustering in plant specialized metabolism. Current Opinion in Biotechnology, 26, 91–99.
Ochoa-Villarreal, M., Howat, S., Hong, S., Jang, M. O., Jin, Y. W., Lee, E. K., et al. (2016). Plant cell culture strategies for the production of natural products. BMB Rep., 49, 149–158.
Bonfill, M., Malik, S., Mirjalili, M. H., Goleniowski, M., Cusido, R., & Palazón, J. (2013). Production and genetic engineering of terpenoids production in plant cell and organ cultures. In K. G. Ramawat & J.-M. Mérillon (Eds.), Natural products: Phytochemistry, botany and metabolism of alkaloids, phenolics and terpenes (pp. 2761–2796). Berlin: Springer.
Malik, S., Bhushan, S., Sharma, M., & Ahuja, P. S. (2016). Biotechnological approaches to the production of shikonins: A critical review with recent updates. Critical Reviews in Biotechnology, 36, 327–340.
Maschke, R. W., Geipel, K., & Bley, T. (2015). Modeling of plant in vitro cultures: Overview and estimation of biotechnological processes. Biotechnology and Bioengineering, 112, 1–12.
Kumar, A. (2015). Improving secondary metabolite production in tissue cultures. In B. Bahadur, M. Venkat Rajam, L. Sahijram, & K. V. Krishnamurthy (Eds.), Plant biology and biotechnology: Volume II: Plant genomics and biotechnology (pp. 397–406). New Delhi: Springer.
Gandhi, S. G., Mahajan, V., & Bedi, Y. S. (2015). Changing trends in biotechnology of secondary metabolism in medicinal and aromatic plants. Planta, 241, 303–317.
Fischer, R., Vasilev, N., Twyman, R. M., & Schillberg, S. (2015). High-value products from plants: The challenges of process optimization. Current Opinion in Biotechnology, 32, 156–162.
Yousefzadi, M., Sharifi, M., Chashmi, N. A., Behmanesh, M., & Ghasempour, A. (2010). Optimization of podophyllotoxin extraction method from Linum album cell cultures. Pharmaceutical Biology, 48, 1421–1425.
Malik, S., Bhushan, S., Verma, S. C., Sinha, A. K., Sharma, M., & Ahuja, P. S. (2008). Effect of pH on cell growth and shikonin derivative formation in Arnebia euchroma suspension cultures. Medicinal and Aromatic Plant Science and Biotechnology, 2, 43–49.
Malik, S., Bhushan, S., Sharma, M., & Singh Ahuja, P. (2011). Physico-chemical factors influencing the shikonin derivatives production in cell suspension cultures of Arnebia euchroma (Royle) Johnston, a medicinally important plant species. Cell Biology International, 35, 153–158.
Malik, S., Andrade, S. A. L., Sawaya, A. C. H. F., Bottcher, A., & Mazzafera, P. (2013). Root-zone temperature alters alkaloid synthesis and accumulation in Catharanthus roseus and Nicotiana tabacum. Industrial Crops and Products, 49, 318–325.
Yousefzadi, M., Sharifi, M., Behmanesh, M., Moyano, E., Bonfill, M., Cusido, R. M., et al. (2010). Podophyllotoxin: Current approaches to its biotechnological production and future challenges. Engineering in Life Sciences, 10, 281–292.
Bourgaud, F., Gravot, A., Milesi, S., & Gontier, E. (2001). Production of plant secondary metabolites: A historical perspective. Plant Science, 161, 839–851.
Zhong, J.-J. (2001). Biochemical engineering of the production of plant-specific secondary metabolites by cell suspension cultures. In J. J. Zhong, S. Y. Byun, G. H. Cho, J. W. Choi, J. R. Haigh, H. Honda, E. James, J. W. Kijne, D. I. Kim, T. Kobayashi, J. M. Lee, M. Kino-oka, J. C. Linden, C. Liu, J. Memelink, N. Mirjalili, H. Nagatome, M. Taya, M. Phisaphalong, R. van der Heijden, & R. Verpoorte (Eds.), Plant cells (pp. 1–26). Berlin: Springer.
Barbuti, A. M., & Chen, Z.-S. (2015). Paclitaxel through the ages of anticancer therapy: Exploring its role in chemoresistance and radiation therapy. Cancers, 7, 2360–2371.
Fett-Neto, A. G., DiCosmo, F., Reynolds, W. F., & Sakata, K. (1992). Cell culture of Taxus as a source of the antineoplastic drug taxol and related taxanes. Nature Biotechnology, 10, 1572–1575.
Kintzios, S. (2008). Secondary metabolite production from plant cell cultures: The success stories of rosmarinic acid and taxol. In K. G. Ramawat & J. M. Merillon (Eds.), Bioactive molecules and medicinal plants (pp. 85–100). Berlin: Springer.
Mulabagal, V., & Tsay, H. S. (2004). Plant cell cultures: An alternative and efficient source for the production of biologically important secondary metabolites. International Journal of Applied Science and Engineering, 2, 29–48.
Bentebibel, S., Moyano, E., Palazon, J., Cusido, R. M., Bonfill, M., Eibl, R., et al. (2005). Effects of immobilization by entrapment in alginate and scale-up on paclitaxel and baccatin III production in cell suspension cultures of Taxus baccata. Biotechnology and Bioengineering, 89, 647–655.
Bonfill, M., Bentebibel, S., Moyano, E., Palazón, J., Cusidó, R. M., Eibl, R., et al. (2007). Paclitaxel and baccatin III production induced by methyl jasmonate in free and immobilized cells of Taxus baccata. Biologia Plantarum, 51, 647–652.
Exposito, O., Syklowska-Baranek, K., Moyano, E., Onrubia, M., Bonfill, M., Palazon, J., et al. (2010). Metabolic responses of Taxus media transformed cell cultures to the addition of methyl jasmonate. Biotechnology Progress, 26, 1145–1153.
Pasquali, G., Porto, D. D., & Fett-Neto, A. G. (2006). Metabolic engineering of cell cultures versus whole plant complexity in production of bioactive monoterpene indole alkaloids: Recent progress related to old dilemma. Journal of Bioscience and Bioengineering, 101, 287–296.
Gupta, O. P., Karkute, S. G., Banerjee, S., Meena, N. L., & Dahuja, A. (2017). Contemporary understanding of miRNA-based regulation of secondary metabolites biosynthesis in plants. Frontiers in Plant Science, 8, 374.
Patil, R. A., Kolewe, M. E., & Roberts, S. C. (2013). Cellular aggregation is a key parameter associated with long term variability in paclitaxel accumulation in Taxus suspension cultures. Plant Cell, Tissue and Organ Culture, 112, 303–310.
Yue, W., Ming, Q. L., Lin, B., Rahman, K., Zheng, C. J., Han, T., et al. (2016). Medicinal plant cell suspension cultures: Pharmaceutical applications and high-yielding strategies for the desired secondary metabolites. Critical Reviews in Biotechnology, 36, 215–232.
Murthy, H. N., Lee, E.-J., & Paek, K.-Y. (2014). Production of secondary metabolites from cell and organ cultures: Strategies and approaches for biomass improvement and metabolite accumulation. Plant Cell, Tissue and Organ Culture, 118, 1–16.
Fett-Neto, A. G., Melanson, S. J., Nicholson, S. A., Pennington, J. J., & Dicosmo, F. (1994). Improved taxol yield by aromatic carboxylic acid and amino acid feeding to cell cultures of Taxus cuspidata. Biotechnology and Bioengineering, 44, 967–971.
Edahiro, J.-I., Nakamura, M., Seki, M., & Furusaki, S. (2005). Enhanced accumulation of anthocyanin in cultured strawberry cells by repetitive feeding of l-phenylalanine into the medium. Journal of Bioscience and Bioengineering, 99, 43–47.
Fait, A., Hanhineva, K., Beleggia, R., Dai, N., Rogachev, I., Nikiforova, V. J., et al. (2008). Reconfiguration of the achene and receptacle metabolic networks during strawberry fruit development. Plant Physiology, 148, 730–750.
Ayan, A. K., Çirak, C., & Yanar, O. (2006). Variations in total phenolics during ontogenetic, morphogenetic, and diurnal cycles in Hypericum species from Turkey. Journal of Plant Biology, 49, 432–439.
Chan, E. K. F., Rowe, H. C., Corwin, J. A., Joseph, B., & Kliebenstein, D. J. (2011). Combining genome-wide association mapping and transcriptional networks to identify novel genes controlling glucosinolates in Arabidopsis thaliana. PLoS Biology, 9, e1001125.
Ramirez-Estrada, K., Vidal-Limon, H., Hidalgo, D., Moyano, E., Golenioswki, M., Cusido, R. M., et al. (2016). Elicitation, an effective strategy for the biotechnological production of bioactive high-added value compounds in plant cell factories. Molecules, 21, 182.
Yousefzadi, M., Sharifi, M., Behmanesh, M., Ghasempour, A., Moyano, E., & Palazon, J. (2010). Salicylic acid improves podophyllotoxin production in cell cultures of Linum album by increasing the expression of genes related with its biosynthesis. Biotechnology Letters, 32, 1739–1743.
Namdeo, A. (2007). Plant cell elicitation for production of secondary metabolites: A review. Pharmacognosy Reviews, 1, 69–79.
Yousefzadi, M., Sharifi, M., Behmanesh, M., Ghasempour, A., Moyano, E., & Palazon, J. (2012). The effect of light on gene expression and podophyllotoxin biosynthesis in Linum album cell culture. Plant Physiology and Biochemistry, 56, 41–46.
Wasternack, C., & Strnad, M. (2017). Jasmonates are signals in the biosynthesis of secondary metabolites: Pathways, transcription factors and applied aspects: A brief review. New Biotechnology. https://doi.org/10.1016/j.nbt.2017.09.007.
Kempinski, C., Jiang, Z., Bell, S., & Chappell, J. (2015). Metabolic engineering of higher plants and algae for isoprenoid production. Advances in Biochemical Engineering/Biotechnology, 148, 161–199.
Russowski, D., Maurmann, N., Rech, S. B., & Fett-Neto, A. G. (2013). Improved production of bioactive valepotriates in whole-plant liquid cultures of Valeriana glechomifolia. Industrial Crops and Products, 46, 253–257.
Ho, T.-T., Lee, K.-J., Lee, J.-D., Bhushan, S., Paek, K.-Y., & Park, S.-Y. (2017). Adventitious root culture of Polygonum multiflorum for phenolic compounds and its pilot-scale production in 500 L-tank. Plant Cell, Tissue and Organ Culture (PCTOC), 130, 167–181.
Tian, L. (2015). Using hairy roots for production of valuable plant secondary metabolites. Advances in Biochemical Engineering/Biotechnology, 149, 275–324.
El Meskaaoui, A. (2012). Plant cell tissue and organ culture biotechnology and its application in medicinal and aromatic plants. Medicinal and Aromatic Plants, 2, e147.
Malik, S., Sharma, N., Sharma, U. K., Singh, N. P., Bhushan, S., Sharma, M., et al. (2010). Qualitative and quantitative analysis of anthraquinone derivatives in rhizomes of tissue culture-raised Rheum emodi Wall. plants. Journal of Plant Physiology, 167, 749–756.
O’Connor, S. E. (2015). Engineering of secondary metabolism. Annual Review of Genetics, 49, 71–94.
Zárate, R., el Jaber-Vazdekis, N., & Verpoorte, R. (2013). Metabolic engineering of plant cellular metabolism: methodologies, advances, and future directions. In S. Chandra, H. Lata, & A. Varma (Eds.), Biotechnology for medicinal plants: Micropropagation and improvement (pp. 359–393). Berlin: Springer.
Nascimento, N. C., & Fett-Neto, A. G. (2010). Plant secondary metabolism and challenges in modifying its operation: An overview. Methods in Molecular Biology, 643, 1–13.
Moldrup, M. E., Geu-Flores, F., de Vos, M., Olsen, C. E., Sun, J., Jander, G., et al. (2012). Engineering of benzylglucosinolate in tobacco provides proof-of-concept for dead-end trap crops genetically modified to attract Plutella xylostella (diamondback moth). Plant Biotechnology Journal, 10, 435–442.
Nour-Eldin, H. H., Geu-Flores, F., & Halkier, B. A. (2010). USER cloning and USER fusion: The ideal cloning techniques for small and big laboratories. In A. G. Fett-Neto (Ed.), Plant Secondary metabolism engineering: Methods and applications (pp. 185–200). Totowa: Humana Press.
De Geyter, N., Gholami, A., Goormachtig, S., & Goossens, A. (2012). Transcriptional machineries in jasmonate-elicited plant secondary metabolism. Trends in Plant Science, 17, 349–359.
Woodson, J. D., & Chory, J. (2008). Coordination of gene expression between organellar and nuclear genomes. Nature Reviews Genetics, 9, 383–395.
Petrussa, E., Braidot, E., Zancani, M., Peresson, C., Bertolini, A., Patui, S., et al. (2013). Plant flavonoids: Biosynthesis, transport and involvement in stress responses. International Journal of Molecular Sciences, 14, 14950–14973.
Ziegler, J., & Facchini, P. J. (2008). Alkaloid biosynthesis: Metabolism and trafficking. Annual Review of Plant Biology, 59, 735–769.
Heinig, U., Gutensohn, M., Dudareva, N., & Aharoni, A. (2013). The challenges of cellular compartmentalization in plant metabolic engineering. Current Opinion in Biotechnology, 24, 239–246.
Lu, Y., Rijzaani, H., Karcher, D., Ruf, S., & Bock, R. (2013). Efficient metabolic pathway engineering in transgenic tobacco and tomato plastids with synthetic multigene operons. Proceedings of the National Academy of Sciences of the United States of America, 110, E623–E632.
Schiml, S., & Puchta, H. (2016). Revolutionizing plant biology: Multiple ways of genome engineering by CRISPR/Cas. Plant Methods, 12, 8.
Chang, W. C., Lee, T. Y., Huang, H. D., Huang, H. Y., & Pan, R. L. (2008). PlantPAN: Plant promoter analysis navigator, for identifying combinatorial cis-regulatory elements with distance constraint in plant gene groups. BMC Genomics, 9, 561.
Negre-Zakharov, F., Long, M. C., & Dudareva, N. (2009). Floral scents and fruit aromas inspired by nature. In A. E. Osbourn & V. Lanzotti (Eds.), Plant-derived natural products: Synthesis, function, and application (pp. 405–431). New York: Springer.
Itkin, M., & Aharoni, A. (2009). Bioengineering. In A. E. Osbourn & V. Lanzotti (Eds.), Plant-derived natural products: Synthesis, function and application (pp. 435–473). New York: Springer.
Butelli, E., Titta, L., Giorgio, M., Mock, H.-P., Matros, A., Peterek, S., et al. (2008). Enrichment of tomato fruit with health-promoting anthocyanins by expression of select transcription factors. Nature Biotechnology, 26, 1301–1308.
Covington, M. F., Maloof, J. N., Straume, M., Kay, S. A., & Harmer, S. L. (2008). Global transcriptome analysis reveals circadian regulation of key pathways in plant growth and development. Genome Biology, 9, R130.
De Costa, F., Yendo, A. C., Fleck, J. D., Gosmann, G., & Fett-Neto, A. G. (2013). Accumulation of a bioactive triterpene saponin fraction of Quillaja brasiliensis leaves is associated with abiotic and biotic stresses. Plant Physiology and Biochemistry, 66, 56–62.
Nascimento, N. C., Menguer, P. K., Henriques, A. T., & Fett-Neto, A. G. (2013). Accumulation of brachycerine, an antioxidant glucosidic indole alkaloid, is induced by abscisic acid, heavy metal, and osmotic stress in leaves of Psychotria brachyceras. Plant Physiology and Biochemistry, 73, 33–40.
Peebles, C. A., Sander, G. W., Hughes, E. H., Peacock, R., Shanks, J. V., & San, K. Y. (2011). The expression of 1-deoxy-D-xylulose synthase and geraniol-10-hydroxylase or anthranilate synthase increases terpenoid indole alkaloid accumulation in Catharanthus roseus hairy roots. Metabolic Engineering, 13, 234–240.
Pan, Q., Wang, Q., Yuan, F., Xing, S., Zhao, J., Choi, Y. H., et al. (2012). Overexpression of ORCA3 and G10H in Catharanthus roseus plants regulated alkaloid biosynthesis and metabolism revealed by NMR-metabolomics. PLoS One, 7, e43038.
Yu, F., & De Luca, V. (2013). ATP-binding cassette transporter controls leaf surface secretion of anticancer drug components in Catharanthus roseus. Proceedings of the National Academy of Sciences of the United States of America, 110, 15830–15835.
Lv, H., Li, J., Wu, Y., Garyali, S., & Wang, Y. (2016). Transporter and its engineering for secondary metabolites. Applied Microbiology and Biotechnology, 100, 6119–6130.
Tarkowski, P., & Vereecke, D. (2014). Threats and opportunities of plant pathogenic bacteria. Biotechnology Advances, 32, 215–229.
Akhgari, A., Yrjonen, T., Laakso, I., Vuorela, H., Oksman-Caldentey, K. M., & Rischer, H. (2015). Establishment of transgenic Rhazya stricta hairy roots to modulate terpenoid indole alkaloid production. Plant Cell Reports, 34, 1939–1952.
Georgiev, M. I., Agostini, E., Ludwig-Muller, J., & Xu, J. (2012). Genetically transformed roots: From plant disease to biotechnological resource. Trends in Biotechnology, 30, 528–537.
Mehrotra, S., Srivastava, V., Ur Rahman, L., & Kukreja, A. K. (2015). Hairy root biotechnology—indicative timeline to understand missing links and future outlook. Protoplasma, 252, 1189–1201.
Sinharoy, S., Saha, S., Chaudhury, S. R., & Dasgupta, M. (2009). Transformed hairy roots of Arachis hypogea: A tool for studying root nodule symbiosis in a non-infection thread legume of the Aeschynomeneae tribe. Molecular Plant-Microbe Interactions, 22, 132–142.
Lefebvre, B., Timmers, T., Mbengue, M., Moreau, S., Herve, C., Toth, K., et al. (2010). A remorin protein interacts with symbiotic receptors and regulates bacterial infection. Proceedings of the National Academy of Sciences of the United States of America, 107, 2343–2348.
Bulgakov, V. P. (2008). Functions of rol genes in plant secondary metabolism. Biotechnology Advances, 26, 318–324.
Srivastava, S., & Srivastava, A. K. (2007). Hairy root culture for mass-production of high-value secondary metabolites. Critical Reviews in Biotechnology, 27, 29–43.
Veena, V., & Taylor, C. G. (2007). Agrobacterium rhizogenes: Recent developments and promising applications. In Vitro Cellular and Developmental Biology-Plant, 43, 383–403.
Crane, C., Wright, E., Dixon, R. A., & Wang, Z. Y. (2006). Transgenic Medicago truncatula plants obtained from Agrobacterium tumefaciens-transformed roots and Agrobacterium rhizogenes-transformed hairy roots. Planta, 223, 1344–1354.
Chandra, S., & Chandra, R. (2011). Engineering secondary metabolite production in hairy roots. Phytochemistry Reviews, 10, 371.
Ludwig-Müller, J., Jahn, L., Lippert, A., Püschel, J., & Walter, A. (2014). Improvement of hairy root cultures and plants by changing biosynthetic pathways leading to pharmaceutical metabolites: Strategies and applications. Biotechnology Advances, 32, 1168–1179.
Thakore, D., Srivastava, A. K., & Sinha, A. K. (2015). Model based fed batch cultivation and elicitation for the overproduction of ajmalicine from hairy roots of Catharanthus roseus. Biochemical Engineering Journal, 97, 73–80.
Liang, Y., Wu, J., Li, Y., Li, J., Ouyang, Y., He, Z., et al. (2015). Enhancement of ginsenoside biosynthesis and secretion by Tween 80 in Panax ginseng hairy roots. Biotechnology and Applied Biochemistry, 62, 193–199.
Zhang, L., Ding, R., Chai, Y., Bonfill, M., Moyano, E., Oksman-Caldentey, K.-M., et al. (2004). Engineering tropane biosynthetic pathway in Hyoscyamus niger hairy root cultures. Proceedings of the National Academy of Sciences of the United States of America, 101, 6786–6791.
Mirjalili, H. M., Fakhr-Tabatabaei, S. M., Bonfill, M., Alizadeh, H., Cusido, R. M., Ghassempour, A., et al. (2009). Morphology and withanolide production of Withania coagulans hairy root cultures. Engineering in Life Sciences, 9, 197–204.
Patra, N., & Srivastava, A. K. (2016). Artemisinin production by plant hairy root cultures in gas- and liquid-phase bioreactors. Plant Cell Reports, 35, 143–153.
Kim, Y. J., Zhang, D., & Yang, D. C. (2015). Biosynthesis and biotechnological production of ginsenosides. Biotechnology Advances, 33, 717–735.
Bulgakov, V. P., Shkryl, Y. N., Veremeichik, G. N., Gorpenchenko, T. Y., & Vereshchagina, Y. V. (2013). Recent advances in the understanding of Agrobacterium rhizogenes-derived genes and their effects on stress resistance and plant metabolism. Advances in Biochemical Engineering/Biotechnology, 134, 1–22.
Panda, B. M., Mehta, U. J., & Hazra, S. (2017). Optimizing culture conditions for establishment of hairy root culture of Semecarpus anacardium L. 3 Biotech, 7, 21.
Thwe, A., Valan Arasu, M., Li, X., Park, C. H., Kim, S. J., Al-Dhabi, N. A., et al. (2016). Effect of different Agrobacterium rhizogenes strains on hairy root induction and phenylpropanoid biosynthesis in tartary buckwheat (Fagopyrum tataricum Gaertn). Frontiers in Microbiology, 7, 318.
Cardillo, A. B., Otálvaro, A. Á. M., Busto, V. D., Talou, J. R., Velásquez, L. M. E., & Giulietti, A. M. (2010). Scopolamine, anisodamine and hyoscyamine production by Brugmansia candida hairy root cultures in bioreactors. Process Biochemistry, 45, 1577–1581.
Guillon, S., Trémouillaux-Guiller, J., Pati, P. K., Rideau, M., & Gantet, P. (2006). Hairy root research: recent scenario and exciting prospects. Current Opinion in Plant Biology, 9, 341–346.
Thimmaraju, R., Venkatachalam, L., & Bhagyalakshmi, N. (2008). Morphometric and biochemical characterization of red beet (Beta vulgaris L.) hairy roots obtained after single and double transformations. Plant Cell Reports, 27, 1039–1052.
Ono, N. N., & Tian, L. (2011). The multiplicity of hairy root cultures: Prolific possibilities. Plant Science, 180, 439–446.
Paddon, C. J., Westfall, P. J., Pitera, D. J., Benjamin, K., Fisher, K., McPhee, D., et al. (2013). High-level semi-synthetic production of the potent antimalarial artemisinin. Nature, 496, 528–532.
Galanie, S., Thodey, K., Trenchard, I. J., Filsinger Interrante, M., & Smolke, C. D. (2015). Complete biosynthesis of opioids in yeast. Science, 349, 1095–1100.
Moses, T., Pollier, J., Thevelein, J. M., & Goossens, A. (2013). Bioengineering of plant (tri)terpenoids: From metabolic engineering of plants to synthetic biology in vivo and in vitro. New Phytologist, 200, 27–43.
Dahm, P., & Jennewein, S. (2010). Introduction of the early pathway to taxol biosynthesis in yeast by means of biosynthetic gene cluster construction using SOE-PCR and homologous recombination. Methods in Molecular Biology, 643, 145–163.
Jeandet, P., Vasserot, Y., Chastang, T., & Courot, E. (2013). Engineering microbial cells for the biosynthesis of natural compounds of pharmaceutical significance. BioMed Research International ID 780145.
Malik, S., Hossein Mirjalili, M., Fett-Neto, A. G., Mazzafera, P., & Bonfill, M. (2013). Living between two worlds: Two-phase culture systems for producing plant secondary metabolites. Critical Reviews in Biotechnology, 33, 1–22.
Acknowledgements
Preparation of this manuscript was supported by grants from Conselho Nacional de Desenvolvimento Científico e Tecnológico – CNPq, Brazil (AGFN) (Grant No. 306079/2013-5), and Fundação de Amparo à Pesquisa e ao Desenvolvimento Científico e Tecnológico do Maranhão - FAPEMA, Brazil (10/2015 BEPP).
Author information
Authors and Affiliations
Contributions
AGFN and SM conceived, outlined the review, and did most of the writing. HNM and FDC assisted in compiling literature data, drafting late versions of the manuscript and making figures. MY, MHM, RA, ASB, MS, and MB collected and helped analyzing the literature, and participated in drafting of the text.
Corresponding author
Ethics declarations
Conflict of interest
The authors declare no conflict of interest.
Electronic supplementary material
Below is the link to the electronic supplementary material.
Rights and permissions
About this article
Cite this article
Matsuura, H.N., Malik, S., de Costa, F. et al. Specialized Plant Metabolism Characteristics and Impact on Target Molecule Biotechnological Production. Mol Biotechnol 60, 169–183 (2018). https://doi.org/10.1007/s12033-017-0056-1
Published:
Issue Date:
DOI: https://doi.org/10.1007/s12033-017-0056-1