Abstract
Aims
Grasslands are among the largest ecosystems in the world and store up to 30% of the global reserves of carbon. Decomposition processes have a crucial role in maintaining carbon balance, but few studies have investigated the heterogeneity of this process at small scale, especially in alpine ecosystems. We aimed at investigating the interactions between decomposition and environmental heterogeneity at microscale (i.e. elevation gradient <1 m) in a subalpine grassland on the western Italian Alps characterised by the presence of parallel hummock and hollow areas.
Methods
In the study area we monitored microenvironmental drivers (soil temperature and soil water content), plant distribution and decomposition. The latter was studied through a litter bags approach followed by elemental analysis, 13C NMR and FT-IR spectroscopies.
Results
Microtopography exerted a direct and indirect control over litter decomposition by affecting plant species distribution and microclimatic conditions. The different elemental and biochemical properties of plants, interacting with microtopography, led to a higher decomposition rate of forb than grass litter, and in hollow than in hummock areas. The observed differences were both quanti- and qualitative.
Conclusions
Decomposition processes bridge the gap between plant community structure and ecosystem functioning, determining a feedback mechanism that maintains ecosystem heterogeneity at the microscale.
Similar content being viewed by others
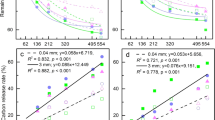
Explore related subjects
Discover the latest articles, news and stories from top researchers in related subjects.Avoid common mistakes on your manuscript.
Introduction

Grasslands are among the largest ecosystems in the world, covering more than one third of the terrestrial surface (Leith 1978; Suttie et al. 2005). They represent a source of ecosystem goods and services, such as forage, and contribute greatly to biodiversity conservation. Furthermore, grasslands are an important carbon sink, storing up to 30% of the global reserves of carbon (C) (Anderson 1991; Eswaran et al. 1993).
The balance between C uptake and release in terrestrial ecosystems is strongly affected by climatic conditions. Climate may affect decomposition directly thorugh its effect on the activity of bacteria and fungi, and is considered the main driver of decomposition processes at global scale (Aerts 1997; Lavelle et al. 1993; Swift et al. 1979). Moreover, climate may have an indirect effect on C balance through its impact on plant community composition and consequently on litter chemistry that drives decomposition at local scale (Aerts 1997; Lavelle et al. 1993). In this framework, studying alpine and subalpine ecosystems is essential because climate change is predicted to have a major effect on these ecosystems, which are ecologically sensitive (Seddon et al. 2016) and at the forefront of climate change impacts.
Despite the large number of studies focused on grassland ecosystems, very few have analysed the environmental heterogeneity of grasslands as a driver of their own functionality (Deák et al. 2015; Güsewell et al. 2005; Pintaldi et al. 2016; Risch et al. 2007; Yu et al. 2011). Grasslands are both structurally and functionally heterogeneous habitats (Risch et al. 2007), where soil moisture and temperature vary in space and time, as well as soil resources. Environmental heterogeneity may occur at different spatial scales, but for alpine grassland communities some resources (e.g. water, nutrients, light) are likely heterogeneous at a scale smaller than 1 m2 (Rose and Malanson 2012). Such a scale is included in the definition of microtopography, that is considered as soil surface variability on the scale of individual plants, within an elevation range from one centimeter to as much as one meter (Moser et al. 2007). Microtopographic relief is often the main driver of small-scale environmental heterogeneity (Deák et al. 2015; Rose and Malanson 2012), affecting properties such as soil organic matter quality, nutrient content, moisture and temperature. Spatial variability of resources can result in different microsites being occupied by species with different ecological niche requirements (Baer et al. 2004; Tilman 1993). These small-scale spatial patterns of plant distribution can influence the rates of both physical and biogeochemical processes that control ecosystem C and nitrogen (N) balance (Schlesinger et al. 1990). Plant-induced heterogeneity in soil resources significantly affects plant growth and survival (Hook et al. 1991), so that a feedback occurs between community structure and ecosystem functioning, which enhances spatial variability of soil resources.
Decomposition processes bridge the gap between plant community structure and ecosystem functioning by affecting soil properties, which are the main source of biotic heterogeneity, and have a crucial role in the aforementioned feedback and, consequently, in vegetation dynamics. Generally, C and nutrient turnover in soils is mainly controlled by litter (including root litter) and soil organic matter decomposition processes, which account for about 70% of total annual carbon fluxes (Raich and Schlesinger 1992). The decomposition of organic matter is driven by litter biochemical composition (e.g. Aerts 1997; Kögel-Knabner 2000), temperature, water and nutrient availability, communities of soil organisms (e.g. Hättenschwiler et al. 2005) and site-specific microenvironmental factors (Berg and McClaugherty 2014). Thus, litter decomposition rate may vary considerably within grasslands, according to the heterogeneity of soil properties and plant distribution. However, little is known about the spatial heterogeneity of soil decomposition rates within grassland ecosystems (Epstein et al. 2002; Risch et al. 2007), and to our knowledge this variation has never been investigated at a microtopographic scale.
Alpine and subalpine microtopography is frequently characterised by hummocky surfaces, resulting from cryoturbation processes (Pintaldi et al. 2016). Hummocks are dome-shaped mounds (Sharp 1942) less than 150 cm high (Grab 2005), generally covered by grasses. The presence of hummocks and hollows (i.e. interhummock areas) may determine small-scale variability in soil properties. However, studies investigating the biotic and abiotic properties of hummocks and hollows in alpine ecosystems are few. Bragazza et al. (1999) studied the microtopography of a poor mire in the Southern Italian Alps and observed that hummocks and hollows were characterised by two well-differentiated plant communities. Moreover, they found that hummocks were drier than hollows and had lower soil pH. Similar results were found more recently by Pintaldi et al. (2016) who analysed the effects of hummocky microtopography on soil properties and soil-vegetation relations in a subalpine grassland. This study highlighted that hummocks were characterised by a lower soil pH and nitrate (NO3−) concentration, and were richer in silt and clay content compared to hollows. Moreover, a differential distribution of plant species was observed, with hummocks dominated by grasses and hollows by forbs, as observed in other areas with similar microtopography (e.g. Güsewell et al. 2005).
Most studies investigating microtopography have focused on plant community structure, whereas only a few have analysed the effects of microtopography on decomposition processes (Beatty and Stone 1986; Dwyer and Merriam 1981) and none has been conducted in alpine or subalpine grasslands. Thus, this study aims to cast light on the feedbacks between soil properties and plant species distributions in a microtopographically heterogeneous subalpine grassland, by investigating plant community structure, microclimatic conditions, and decomposition rates at an elevation gradient smaller than 1 m. In this study we hypothesised that: (i) forb litter decomposes faster than grass litter both in hummock and hollow areas due to their different biochemical composition; (ii) litter decomposition rate is faster in hollows than in hummocks due to different microclimatic conditions and soil properties; (iii) the qualitative decomposition pattern of forb and grass litter differs between litter types but also between microhabitats; (iv) a positive feedback between litter quality and soil properties occurs in hummocks and hollows.
Materials and methods
Study area
The study was carried out in a subalpine grassland, in the north-western Italian Alps, from September 2014 to June 2016. The site is an abandoned pasture that was grazed until 2007 and is located in the Aosta Valley region at a short distance from the village of Torgnon at an elevation of 2160 m asl (45° 50′ 40” N, 7° 34′ 41″ E). In the 0.3 ha area used for the study the dominant vegetation consists of Nardus stricta L., Festuca nigrescens All., Arnica montana L., Carex sempervirens Vill., Geum montanum L., Anthoxanthum alpinum L., Potentilla aurea L. and Trifolium alpinum L. The terrain is undulating, with an average slope of 4°, and is south facing (195°). The soil is classified as Cambisol (FAO/ISRIC/ISS) and shows a microtopographical pattern characterised by the presence of cryogenic hummock and hollow areas. Where the surface is flat, hummocks are organised in a non-sorted net, whereas they develop into parallel stripes along the slope direction, when the slope angle increases (Pintaldi et al. 2016). The site is characterised by an intra-alpine semi-continental climate, with mean annual temperature of 3.1 °C and mean annual precipitation of 880 mm. On average, from the end of October to late May, the site is covered by a thick snow cover (90–120 cm) which limits the growing period to about 5 months during the year (Galvagno et al. 2013).
The study focused on the dominant microtopographical pattern observed in the study area that is characterised by the presence of parallel convex stripes 15–30 cm above the ground level (i.e. hummocks) alternating with parallel concave stripes 15–30 cm below the ground level (i.e. hollows), which both cover the great majority of the grassland surface (Fig. 1). An area of hummocks (Hu), 70-m long with a surface of ~25 m2, was randomly selected among the convex stripes together with the area of hollows (Ho) downline of the selected hummock area. Within each area (i.e. Hu and Ho) we identified three plots with a surface of ~10 m2 which were 20 m far apart (A, B, C), where we investigated decomposition processes. Finally, microenvironmental drivers and plant species distribution were monitored within the inter-plot areas ~10 m before and after each plot (Fig. 1).
Photo showing the microtopographical pattern that characterised the study area; the dashed and solid lines mark respectively an example of hollow and hummock area (above). The sampling design is schematised below: the grey squares are the plots (i.e. A-C) used for litter bags installation (n = 3); empty and solid circles represent the locations of microclimatic monitoring (n = 4); vegetation surveys were carried out in correspondence with solid circles (n = 2)
Microenvironmental drivers
Measurements of soil temperature (Ts) (PT100, Campbell Scientific) and soil water content (wcs) (CS616, Campbell Scientific) at 5 cm depth were recorded every 30 min from May 2015 (Day Of the Year 121) to May 2016 (DOY 130) at four replicate locations within each area (8 locations in total), so that two sensors (i.e. Ts + wcs) were installed 10 m before and after each plot (Fig. 1). Temperature and moisture data were used to describe the microenvironmental conditions in Hu and Ho. Mean annual soil temperature and soil moisture were calculated along with mean seasonal values representing the growing season (May to December 2015) and the winter period (January to May 2016)-.
Temperature data were also used to calculate: (i) Diurnal Temperature Range (DTR = monthly mean difference between daily max and min temperature), (ii) Growing Degree Days (GDD = Σ (daily mean temperature – T0) for all days when mean temperature > T0), where T0 was set to 5 °C, and (iii) Freezing Hours (FH = annual number of hours for which mean temperature < 0 °C).
Vegetation survey
Vegetation surveys were carried out to confirm the presence of the same plant species distribution pattern already observed by Pintaldi et al. (2016). Vegetation surveys were performed every ~15 days to an amount of 10 surveys from May 2015 to September 2015 in 30 × 30 cm2 vegetation plots with two replicates for each area type; both in Hu and Ho one vegetation plot was located between the plots A and B, and the other one between the plots B and C (Fig. 1). During each survey the percentage cover of all plant species occurring in the plot was recorded. The mean seasonal cover of each species was calculated for each vegetation plots in Hu and Ho to give a general overview of the plant community occurring in each microhabitat. Moreover, the species were grouped in two functional types, grasses (G) and forbs (F), which are the same used to investigate litter decomposition.
Decomposition rates
Litter decomposition rates were measured over a 2-year period (2014–2016) using surface-placed litter bags collected every ~30 days during the growing season 2015 and once after snowmelt in 2016 (6 harvests). Fresh aboveground biomass was collected in the grassland in September 2014 immediately before senescence in order to ensure adequate representation of the plant community composition occurring immediately before the snowfall, when ~90% of the green grass biomass was represented by Nardus stricta, and green forb biomass was composed of Trifolium alpinum, Geum montanum and Arnica montana with other species representing less than 10% of the biomass. Early developing species such as Crocus vernus, Ranunculus pyrenaeus and Pulsatilla alpina were not present in the samples since their decomposition had already started. Biomass collection was carried out within an area without any microtopographical pattern, where twelve samples of biomass were collected using a wooden quadrat (900 cm2), randomly located at least 10 m far from previous sampling points, and a cordless grass shear (Makita-UM-104 DW). Senescent material and seeds were removed in order to recover all the green biomass, which was then sorted into grasses and forbs by hand. The material was then oven dried to a constant weight at 60 °C for 24 h. Thirty-six litter bags (6 harvests × 2 litter types × 3 replicates) were prepared for each microhabitat (Hu and Ho), using 10 × 8 cm bags made from 0.5 mm nylon mesh, into which ca. 4 g of green dried biomass was accurately weighed. For each microhabitat, half of the bags were filled with forb litter (FL) and the remaining half with grass litter (GL) according to the above cited community composition. Two additional 4 g samples of biomass from each functional type were retained for the determination of initial litter characterisation. Litter bags were divided in three replicate groups (12 litter bags each: 6 FL + 6 GL) and each group was installed in a different plot (i.e. A, B, C) in October 2014 (DOY 283), pinned to the soil surface ensuring good contact between the soil and the litter.
Following winter incubation under the snow cover, 1 bag per litter type was collected from each of the three plots (3 replicates × litter type) both in Hu and Ho after the snowmelt (1st May 2015; DOY 121) and then every ~30 days, from May 2015 to September 2015. Twelve bags (3 replicates × 2 litter types × 2 microhabitats) were left in field and incubated for a second winter period and then collected in June 2016 after the snowmelt (10th May 2016; DOY 130). After each collection, the bags were immediately opened and the samples sorted, removing any visible plant roots, soil animals and soil particles. The remaining litter was oven dried at 65 °C for 48 h, its dry weight recorded to investigate remaining litter mass (RLM) and then milled prior to chemical analyses.
All the milled litter samples, including initial samples (n = 72 + 6), were analysed for total C and N content (CN Analyser, CE Instruments NA2100, Rodano, Italy). Total silicon content was also determined by extraction with 50%NaOH and spectrophotometric analysis at 820 nm (Elliott and Snyder 1991), only for three time points (i.e. 0, 228 and 257 days of incubation).
Then, the three replicates of milled litter (i.e. A, B, C) were mixed in order to obtain one total sample per each combination of litter type, time point and microhabitat. Three key time points were selected to describe the qualitative decomposition pattern of Fl and GL both in Hu and Ho: (1) initial, (2) winter incubation (228 days), and (3) winter + summer incubation (357 days). According to these time points, 10 of the total samples were selected to determine biochemical composition and dynamics of decomposition by carrying out solid-state 13C Nuclear Magnetic Resonance (13C NMR) and Fourier-Transform Infrared Spectroscopy (FT-IR).
13C CPMAS spectra were collected on a Jeol ECZR 600 instrument, operating at a frequency of 600.13 and 150.91 MHz for 1H and 13C, respectively. Samples were packed in cylindrical zirconia rotors (3.2 mm o.d.), with a sample volume of 60 μl. The spectra were acquired at room temperature with a rotation frequency of 20 kHz. All experiments employed the RAMP-CP pulse sequence (1H 90° pulse = 2.1 μs; contact time = 1 ms; optimized relaxation delays of 2 s; 5000 scans) with the TPPM 1H decoupling (rf field = 112 kHz) during the acquisition period. 13C chemical shift scale was referenced with the resonance of glycine as external standard. The 13C NMR spectra were divided into the following regions: 0–45 ppm (C in straight chain, branched, and cyclic alkanes); 46–60 ppm (C in branched aliphatics, amino acids, and OCH3 groups); 61–110 ppm (C in carbohydrates and aliphatics containing C bonded to OH); 111–155 ppm (aromatic C); 156–165 ppm (C in phenolic groups); 166–190 ppm (C in carboxyl, amide, and ester groups) (Almendros et al. 2000; Celi et al. 1997; Kögel-Knabner 2002; Spaccini et al. 2006). Spectra were elaborated using TOPSPIN 1.3 software (Bruker BioSpin GmbH, Rheinstetten, Germany). Fourier Transform Infrared (FT-IR) spectra of litter samples were acquired between 4000 and 400 cm−1 with a resolution of 4 cm−1 (PerkinElmer Spectrum 100, USA) on pellets obtained by pressing 2.0 mg of finely milled litter samples with 200 mg of KBr. The following peaks and bands were identified and used to describe the qualitative decomposition pattern: 3400 cm−1 (hydrogen bonded–OH of saccharide groups); 2920 cm−1 (–CH2– stretching of aliphatic compounds); 2852 cm−1 (–CH– stretching of aliphatic compounds); 1720 cm−1 and 1200 cm−1 (–COOH functional groups); 1512 cm−1 (skeletal aromatic stretching of lignin); 1150–1050 cm−1 (alcoholic and polysaccharide C–O stretching) (Piccolo and Stevenson 1982).
Data analysis
Differences in soil temperature and soil water content between Hu and Ho were analysed with the Student’s t test, as well as the intial differences in C, N and Si content, and C:N ratio between litter types. Pearson’s correlation coefficient was calculated to test total N correlation with the integration values of saccharidic C and paraffinic C. The effects of microhabitat and litter type on RLM, C:N ratio, C and N losses were analysed using generalized linear models (GLMs), and pairwise comparison were performed with Tukey’s post-hoc test. The package ‘caret’ was used to evaluate the importance score of predictor variables included in the models. Before analyses, data were transformed when necessary. Finally, Detrended Correspondence Analysis (DCA) and Principal Component Analysis (PCA) were performed in order to visualise the differences between plant communities in Hu and Ho, and between the chemical properties of FL and GL before and during decomposition. The change (X) in RLM, as percentage of the initial value (X0) at time t (days of incubation) was described by the following equation (Olson 1963):
where k (day−1) is the decomposition rate constant (Olson 1963; Swift et al. 1979). Finally, C and N loss was expressed as mg of element released from 1 g of initial litter according to the following equation:
where EL is the amount of C or N released from each gram of litter at time t, E0 and Et are the total content (mg) of C or N at the beginning and during decomposition at different times respectively; and X0 is the initial value of litter mass (g), which in our experiment was always 4.0 g.
All the statistical analyses and plots were performed using the computing environment R 3.4.0 (R Development Core Team, 2005) and the following packages: ‘ggplot2’, ‘multcomp’, ‘Rmisc’, and ‘vegan’.
Results
Microenvironmental drivers
Soil temperature (Ts) did not differ significantly between hollows (Ho) and hummocks (Hu) (Online Resource 1a). Mean annual Ts was 5.1 ± 0.3 °C and 5.2 ± 0.3 °C for Ho and Hu respectively. Mean Ts during the growing season (May–December 2015) was 7.6 ± 0.3 °C in Ho and 7.8 ± 0.3 °C in Hu, while under the snow cover (January–May 2016) a mean soil temperature of 0.2 ± 0.05 °C was observed in both microhabitats. Moreover, no significant differences were found in Diurnal Temperature Range (DTR) and Growing Degree Days (GDD) between Hu (DTR = 1.38 ± 0.15 °C; GDD = 1074 ± 48) and Ho (DTR = 1.29 ± 0.33 °C; GDD = 1125 ± 36). However, Hu showed a significantly (t = −3.03; P < 0.05; n = 4) higher number of Freezing Hours (FH = 1295 ± 392) compared to Ho (FH = 607 ± 179).
A completely different pattern was observed for the soil water content (wcs) of Hu versus Ho (Online Resource 1b). The mean annual wcs measured in Hu (16 ± 0.05%) was significantly lower (t = 3.23; P < 0.5; n = 4) than the mean annual water content within Ho (26 ± 0.02%). Mean wcs remained constant in Ho with no differences between growing season and winter, whereas Hu showed slightly different values of mean wcs during growing season (18%) and under the snow cover (16%), although the difference was not significant.
Plant community composition
Plant community composition strongly differed between Hu and Ho (Online Resource 2) as already observed in Pintaldi et al. (2016). In Hu grass cover was dominant (up to 88%) and mainly composed by Nardus stricta, while forbs dominated in Ho (up to 85% cover), with Geum montanum, Arnica montana and Crocus vernus the most abundant species (Table 1). Almost half of the species identified during the vegetation survey were found in both microhabitats but with different percentage covers, while the other species occurred in only one of the two microhabitats.
Chemical composition of grasses and forbs, and changes with incubation
Litter mass, carbon and nitrogen
In the initial litter samples, forb litter (FL) had a significantly higher N content than grass litter (GL) (t = −9.39; P < 0.01; n = 3). Nitrogen content averaged 0.9 ± 0.02% and 1.4 ± 0.1% in FL and in GL, respectively. Similar results (t = 52.44; P < 0.001; n = 3) were obtained for silicon (Si) which comprised 1.13 ± 0.05% in GL and 0.08 ± 0.01% in FL. Carbon content did not significantly differ between GL (44.3 ± 0.7%) and FL (43.0 ± 0.4%), leading to a significantly higher (t = 11.56; P < 0.01; n = 3) C:N ratio in grasses (50.1 ± 1.4) compared to forbs (31.5 ± 2.4).
After the first winter incubation (228 days) FL mass decreased by 75.6 ± 1.6% in Ho and 70.5 ± 0.5% in Hu, whereas GL mass decreased by 48.8 ± 0.2% in Ho and 49.3 ± 0.1% in Hu (Fig. 2a). After the second winter incubation (628 days) FL mass decreased by 85.4 ± 1.4% in Ho and 79.8 ± 4% in Hu, whereas GL mass decreased by 65.2 ± 1.1% and 65.5 ± 1.7% in Ho and Hu, respectively (Fig. 2a). The analysis of RLM showed that the difference between microhabitats was significant (P < 0.001) only for FL. On the contrary, the difference between FL and GL decomposition rates within the same microhabitat was always significant (P < 0.001). The decomposition rate (k) over the whole period was calculated according to the single exponential model (Eq. 1) by fitting an exponential curve to the percentage of remaining litter mass (RLM), with the origin forced to 100 (Fig. 2a). Decomposition rate decreased in the order: FL in Ho > FL in Hu > GL in Ho > GL in Hu, with k’ (k’ = k × 105) respectively equal to 419 (R2 = 0.54), 339 (R2 = 0.59), 218 (R2 = 0.69) and 206 (R2 = 0.79). The litter mass loss occurring under the snow during the first winter incubation (228 days) represented the main part of the process, with a relative percentage of the total decomposition of 89.4 and 88.7% for FL, and 75 and 74% for GL, in Ho and Hu respectively occurring over the first winter.
Parallel to the decrease of RLM, no significant differences were found in the C:N ratio between the two microhabitats. Nevertheless, during the decomposition process the C:N ratio of GL was always significantly higher (P < 0.001) than the one of FL. After the firs winter incubation (228 days) the C:N ratio of FL decreased from 31.5 ± 2.4 to 16.1 ± 0.5 in Ho and 13.4 ± 1.3 in Hu, whereas C:N of GL decreased from the initial value of 50.1 ± 1.4 to 28.6 ± 0.4 in Ho and 28.7 ± 2.6 in Hu. Finally, after 628 days of incubation, the C:N ratio decreased up to 14.7 ± 1.9 in Ho and 13.1 ± 0.4 in Hu for FL, and up to 25.1 ± 1.6 in Ho and 23.3 ± 0.9 in Hu for GL (Fig. 2b). The decrease of C:N ratio was mainly due to the increase of total N content (%) occurring both in FL and GL. Values of total percentage content of C and N before and during decomposition are reported in Table 2.
Considering the absolute loss of C expressed in mg released from 1 g of litter mass (mg g−1), after the first winter incubation (228 days) FL released 315.6 ± 4.3 mg g−1 of C in Ho and 289.6 ± 3.0 mg g−1 in Hu, whereas GL released 216.6 ± 8.6 mg g−1 of C in Ho and 217.2 ± 7.6 mg g−1 in Hu. After 628 days of incubation, FL released 361.2 ± 9.5 mg g−1 of C in Ho and 335.7 ± 21.9 mg g−1 in Hu, and GL released 291.0 ± 10.2 mg g−1 and 293.0 ± 8.1 mg g−1 respectively in Ho and Hu (Fig. 2c). The difference between microhabitats in terms of C loss was significant (P < 0.001) only for FL. In general the amount of C released into the soil by the litter was always significantly higher (P < 0.001) in FL than GL within the same microhabitat.
In terms of N, after 228 days, FL released 6.6 ± 1.5 mg g−1 of N in Ho and 3.1 ± 0.6 mg g−1 in Hu, whereas GL released 0.9 ± 0.3 mg g−1 of N in Ho and 1.0 ± 0.9 mg g−1 in Hu. After 628 days of incubation, FL released 8.9 ± 0.6 mg g−1 of N in Ho and 6.5 ± 0.7 mg g−1 in Hu, and GL released 2.8 ± 0.1 mg g−1 and 2.4 ± 0.4 mg g−1 respectively in Ho and Hu (Fig. 2d). Thus, FL released a significantly (P < 0.001) higher amount of N in Ho than in Hu, whereas GL did not release significantly different amounts of N in the two microhabitats. In general the amount of N released into the soil by the litter was always significantly higher (P < 0.001) in FL than GL within the same microhabitat.
Litter type had a significant effect (P < 0.001) on decomposition rate in terms of RLM, C:N ratio, C and N loss. Similarly, microhabitat showed a significant effect (P < 0.001) on RLM, and C and N loss, but no significant effect of this factor on C:N was observed. Moreover, the interaction between litter type and microhabitat was always significant (P < 0.05). As expected, time was negatively correlated with the amount of RLM (P < 0.001). Generally, decomposition of the surface-placed litter was faster for FL than for GL, and faster in Ho than in Hu within the same litter type. Time, litter type and microhabitat were used as predictor variables of RLM in a generalised linear model that was able to explain the 96.9% of the deviance. The predictor variables of the model were sorted on the basis of their importance score as following: litter type > time > microhabitat. Since litter type was the most important predictor, the following model was built only on FL data in order to exclude litter type effect and investigate the importance of microenvironmental drivers. A new linear model was built using number of freezing hours, soil temperature and soil water content as predictor variables of the response variable RLM. The percentage of explained deviance in this model was 50.9%, and the predictor variables were sorted on the basis of their importance score as following: time > soil water content > soil temperature > freezing hours. Time (P < 0.001) and soil water content (P < 0.01) were the only variables showing a significative effect on RLM.
13C NMR
Solid-state 13C NMR spectra and the relative C distribution of FL and GL before incubation are shown in Figs. 3, 4, and 5 and Table 2. The 13C NMR region between 166 and 190 ppm was dominated by a signal at 172 ppm (carboxyl C), which was much more prominent in forbs (6.0% of total C) compared to grasses (2.4%) in the initial samples (Table 2; Fig. 3a). During decomposition, FL incubated in Hu became enriched in carboxyl groups, reaching 9.6% after 228 days before slightly declining after 357 days of incubation (Table 2; Fig. 4c), whereas FL incubated in Ho underwent a soft variation during decomposition (Table 2; Fig. 4a). Grass litter showed an opposite trend with greater changes in Ho than in Hu (Table 2; Fig. 5a,c).
In the phenolic and aromatic region, respectively at 156–165 ppm and 111–155 ppm, the initial litter samples exhibited weak signals at 148 and 133 ppm, more pronounced in FL (Table 2; Fig. 3a). Higher and broader signals were observed in the aromatic region of the decomposed materials, especially after 228 days in FL incubated in Hu (Table 2; Fig. 4c), although the integration area showed a progressive increase only in GL in both microhabitats, while in FL it remained nearly constant after winter incubation and slightly decreased during summer incubation (Table 2; Fig. 4a,c).
The most prominent signals occurred in the 61–110 ppm region, which represented more than 60% of total C in the initial FL samples and reached 82.5% of total C in GL. The signal at 73 ppm is associated with the simultaneous resonance of C–2, C–3 and C–5 of pyranoside rings in cellulose and hemicellulose, accompanied by the signals at 88 ppm (C-1) and 83 ppm (C-4 in amorphous cellulose) (Table 2; Fig. 3a). The signals at 65 ppm (C–6) and at 105 ppm (anomeric C) were more pronounced in GL than in FL, highlighting the presence of crystalline cellulose. The integration values in the 61–110 ppm region drastically decreased for FL in Hu, from 64.2 to 39.7%, after 228 days of incubation, and then increased to 50.1% during summer incubation (Table 2; Fig. 4c), whereas in Ho, saccharidic C had undergone a more intense decline in the summer period (Table 2; Fig. 4a). Grass litter showed instead a slow continuous reduction with no differences between Hu and Ho (Table 2; Fig. 5a,c). A shoulder at 56 ppm attributable to lignin methoxyl C and more defined in GL than in FL indicated the presence of lignin in the initial samples. In FL this signal was accompanied by a smaller one at 54 ppm, which could be due to C-N groups. With incubation, the latter signal strongly increased in FL, especially in Hu (Table 2; Fig. 4c) and to a less extent in Ho (Table 2; Fig. 4a), whereas in GL the signal at 56 ppm remained well defined and showed just a weak increase (Table 2; Fig. 5a,c).
In the 0–45 ppm region the signals at 33, 31 and 21 ppm, most likely attributable to paraffinic C of lipids and waxes, were not so sharp but their integration area was very different in FL and GL before decomposition, being 14.7 and 5.1%, respectively (Table 2; Fig. 3a). These signals underwent a trend similar to the one at 56 ppm, with a net increase in FL especially in Hu (Table 2; Fig. 4a,c) and a lower enrichment in GL (Table 2; Fig. 5a,c). As a consequence both the alkyl C/aryl C and alkyl C/O-alkyl C ratios increased more markedly in FL than in GL, and relatively to FL, more in Hu than in Ho.
The main chemical differences described above are visually summarised in Fig. 6. The PCA ordination analysis according to C-types data showed that the 62.8% of the variance between GL and FL was explained by the PC1 which was positively correlated with the saccaridic C (r = 0.87; P < 0.01; n = 10) and negatively correlated with –COOH (r = −0.95; P < 0.001; n = 10), phenolic C (r = −0.86; P < 0.01; n = 10), and aromatic C (r = −0.81; P < 0.01; n = 10).
Principal component analysis (PCA) of the integration values obtained from 13C NMR spectra of forb and grass litter before decomposition (0 days of incubation), and during incubation after 228 days (winter) and after 357 days (winter + summer); significantly correlated variables were not reported in the graph
Paraffinic C obtained by NMR spectra integration were tested for correlation with N content. Results showed a significant positive correlation between paraffinic C and total N content (Fig. 7a) both for FL (r = 0.91; P < 0.05; n = 5) and GL (r = 0.91; P < 0.05; n = 5), whereas saccharidic C was negatively correlated with N content (Fig. 7b) although the correlation was significant only for GL (r = −0.89; P < 0.05; n = 5).
FT-IR
FT-IR spectra confirmed the main differences between FL and GL and the effects of decomposition processes. The spectra showed bands, centered at 3400 cm−1, due to hydrogen bonded-OH of saccharidic groups. Although the hydration water present in the samples may mask the contribution of the –OH containing molecules, these bands were much more pronounced in the initial GL than in FL (Fig. 3b) and decreased with decomposition, similarly to the behaviour observed in the 13C NMR spectra. This is further confirmed by the bands at 2920 and 2852 cm−1 due to -CH2- and -CH- stretching of aliphatic compounds and by the bands at 1150 to 1050 cm−1, due to alcoholic and polysaccharidic C-O stretching which were more pronounced in initial GL than in FL (Fig. 3b). The latter may be attributed also to vibrations of Si-O groups (Socrates 2004), in line with Si data (Table 2). The bands in the 1150 to 1050 cm−1 decreased with decomposition more in FL than in GL and more in Hu than in Ho (Fig. 4b,d and 5b,d). Similarly, the peaks at 1720 and 1200 cm−1, indicating the presence of -COOH functional groups (Celi et al. 1997), were sharper in initial FL than in GL (Fig. 3b) and increased in the former with decomposition, again more in Hu than in Ho (Fig. 4b,d and 5b,d). A small sharp peak at 1512 cm−1, assigned to skeletal aromatic stretching of lignin, was present only in initial GL (Fig. 3b) and apparently did not vary with decomposition (Fig. 4b,d and 5b,d). In FL, a new absorption shoulder, slightly shifted to higher frequencies (1540 cm−1, amide II) appeared with decomposition: in Ho (Fig. 4b) it increased progressively with time whereas in Hu it increased during winter incubation (228 days) and then decreased during summer incubation (Fig. 4d). Broad absorption from 1260 to 1200 cm−1, observed in all spectra, suggests C-O and C-O-C vibrations of a wide variety of materials, but without a clear trend.
Discussion
This study highlighted that hummock-hollow microtopography is an important source of heterogeneity that influences both the structure and functioning of a subalpine grassland. Above all, we demonstrated that decomposition processes can show variability even at a microscale (i.e. elevation gradient lower than 1 m) due to the presence of hummocks and hollows. The control of microtopography over decomposition processes can be exerted directly, by influencing abiotic factors, such as microclimatic conditions and soil properties, and indirectly, by means of biotic factors, such as plant species distribution. Decomposition processes bridge the gap between the abiotic and biotic components in the study ecosystem, enhancing the soil properties of hummocks and hollows through differential decomposition rates and nutrient turnover. Indeed, the more eutrophic soil conditions of hollows, which are dominated by forbs producing high quality litter, maintain a high nutrient content thanks to faster decomposition rates. On the other hand, more dystrophic soil conditions of hummocks, which are dominated by grasses with low nutrient requirements producing recalcitrant litter, create hostile habitats for forbs where oligotrophic grasses as N. stricta are much more competitive.
Most studies investigating the structural and functional heterogeneity of alpine and arctic ecosystems have focused on mesotopographical gradients. At this scale, different species composition and consequent changes in litter quality have been reported as a consequence of gradients of temperature, soil moisture and snow cover duration (e.g. Baptist et al. 2010; Britton et al. 2011; Miller and Alpert 1984). Only a few studies have investigated the effects of topography at a smaller scale (i.e. microtopography) and all of them focused primarily on soil properties and/or plant community structure (Bragazza et al. 1999; Pintaldi et al. 2016; Sebastiá 2004), rather than ecosystem functioning. Moreover, most studies addressing the role of topography at a small scale have been carried out in peatlands (e.g. Bragazza et al. 2007) whereas alpine and subalpine grasslands have received less attention despite their ecological and economic value. To our knowledge, our study is the first to investigate the direct and indirect control of microtopography over the ecosystem functioning of a subalpine grassland, considering in detail the influence on decomposition processes.
Direct control of abiotic factors over litter decomposition
The presence of hummock and hollow areas significantly affected forb litter decomposition which was faster in hollows than in hummocks, however, this difference was not observed for grasses. We suggest that the effect of microhabitat was not detected for grasses because of the low decay rate of their litter, which hid the potential effect of microenvironmental conditions. This observation conforms to suggestions that litter quality is the major driver of decomposition at a small scale (Lavelle et al. 1993; Swift et al. 1979). However, it is well known that climate exerts its direct control especially over early-stage decomposition (Berg and McClaugherty 2014) thus, a faster decay of forbs in hollows than in hummocks can be explained by reference to microenvironmental drivers, such as soil temperature and moisture.
The model used suggested that microclimatic control of decomposition processes was likely due to soil moisture since water content was the only predictor among microenvironmental variables (i.e. soil temperature, number of freezing hours and soil water content) having a significant effect on remaining litter mass. This finding is consistent with the microclimatic conditions we observed in the two microhabitats: the water content in hollows was significantly higher than in hummocks during the whole experiment, and it may have resulted in greater nutrient illuviation and higher activity of microbial community in hollows. On the contrary, no significant differences in soil temperature were observed between the two microhabitats, neither during winter nor summer, whereas the number of freezing hours was significantly higher in hummocks than in hollows. Soil moisture is essential for guarantying an active microbial and biochemical decomposition. Indeed, plant litter may contain significant amounts of soluble compounds (i.e. sugars, phenolics, aminoacids, and glycerides) that may be leached out of the litter at a rate depending on water availability and litter species (Berg and McClaugherty 2014; Swift et al. 1979). Moreover, it is well known that generally low soil moisture constrains decomposition by slowing down microbiological activity (Sanaullah et al. 2012; Santonja et al. 2015; Swift et al. 1979). Thus, our results are consistent with the idea that soil moisture is the main limiting climatic factor of decomposition processes, as previously observed in xeric habitats (Seastedt et al. 2001) as well as in the cool, wet, oceanic alpine environment (Britton et al. 2011). However, the effect of soil moisture heterogeneity produced by microtopography on decomposition processes is not well documented, and the few studies available on this topic are often contradictory (Beatty and Stone 1986; Dwyer and Merriam 1981). The inconsistency in reported effects of hummocks/mounds and hollows/pits on litter decay is the outcome of the non-linear relationship between decomposition rate and soil water content. An optimum decomposition rate was found at intermediate moisture ranges in alpine ecosystems (Bryant et al. 1998; O’Lear and Seastedt 1994; Seastedt et al. 2001), while values of soil saturation above 80% may lead to anoxic conditions that hamper decomposition. The values of soil moisture we observed in hollows fell within an intermediate moisture range, with values always above 10% water-holding capacity contrary to hummocks. This percentage can be considered as a threshold under which soil moisture can become very limiting for decomposition (Berg and McClaugherty 2014).
On the other hand, even though the number of freezing hours did not show a significant effect on remaining litter mass in the model, we observed that the amount of hours with temperature < 0 °C in hummocks was about double the amount in hollows. This suggests that the snow cover was either shorter in duration or less thick in hummocks than in hollows, and this may have played a role in slowing down the decay processes in hummocks. Many studies have reported the importance of snowpack, both in terms of duration and depth, for insulating alpine soils from low winter temperatures, and have highlighted that up to 80% of mass loss may occur under the snow during winter (e.g. Baptist et al. 2010; O’Lear and Seastedt 1994; Saccone et al. 2013). Freeze-thaw events, occurring when the insulating effect of the snowpack is removed, have the potential to negatively affect soil microbial biomass as a consequence of the harsh conditions experienced by soil organisms (Jefferies et al. 2010). Indeed, low temperatures represent an energy-limited system for bacteria and fungi which are forced to slow down their activity. This phenomenon can be explained by two factors: the direct effect of low temperature on microbial respiration rate and enzyme kinetics, and also the limiting availability of soil water solution in liquid phase (Gavazov 2010).
Plant species distribution and indirect control on litter decomposition
Microtopography influences water availability in soil and determines simultaneously the loss and accumulation of nutrients and soil particles in different sites, developing and maintaining a heterogeneous ecosystem. The differences in soil properties between hummocks and hollows observed in the study area (Pintaldi et al. 2016) led to a specific pattern of plant distribution, so that hummocks were dominated by grasses, especially Nardus stricta, and hollows by forbs, with Arnica montana, Geum montanum, Crocus vernus and Galium verum dominant. This pattern depends on the interaction of abiotic factors and complex biotic processes, such as competition or facilitation among species. It is well known that N. stricta is an oligotrophic species preferring acidic and poor substrates rich in fine fraction (i.e. silt and clay) that are similar to the soil of hummocks, and it does not compete successfully with other species where soils are more fertile (Chadwick 1960; Gerola and Gerola 1951-1954), as in the hollows in our study system. Moreover, Nardus can be considered a ‘nitrophobic’ species (Coulon 1923) since its yield may decrease as a consequence of high concentrations of N (Bradshaw et al. 1964). Regarding the microclimatic conditions, N. stricta is extremely frost-hardy, as its distribution in Europe suggests (Chadwick 1960), and exhibits some xeromorphic characteristics (Coulon 1923). These features are essential to colonize and dominate the hummock environment, characterised by a higher number of freezing hours and a lower water availability than in hollows. Moreover, Nardus is a tussock-forming graminoid and, where it dominates, invasion by other plant species is difficult because of the tightly packed and dense tussocks in which this grass grows (Tutin et al. 1964–1980).
As Cornelissen and Thompson (1997) demonstrated, the leaf traits that graminoids have developed as adaptations for growth and defense in specific environments are strongly linked to litter decomposability. Thus, the presence of different dominant species in hummocks and hollows leads to variability in the plant traits that drive decomposition processes. In other words, the heterogeneity of water and nutrient availability caused by microtopography influence decomposition processes by generating a patchy distribution of plant traits relating to litter quantity (i.e. biomass) and quality (i.e. chemical properties), such as growth rate, C:N ratio, lignin, and silicon content (De Deyn et al. 2008). Therefore, as litter physico-chemical features are the main drivers of decomposition processes at a local scale (Aerts 1997; Cornelissen 1996; Swift et al. 1979), microtopography exerts an indirect control on decomposition through its influence on plant species distribution. It is well documented that grasses contain less foliar mineral nutrients (i.e. potassium, calcium, magnesium) than herbaceous forbs, but are richer in silicon (Cornelissen and Thompson 1997; Schaller et al. 2016). Moreover, compared with other grass species growing in acidic soils of grasslands and moorlands, N. stricta shows a an exceptionally high silicon content (Coulon 1923). Silicon content was very high in our study and resulted an important predictor of leaf decay, showing a negative correlation with decomposition rate. On the contrary, forbs are generally characterised by higher content of calcium, potassium and magnesium which are known to be positively related to decomposition (Cornelissen and Thompson 1997). These basic cations provide indeed an important nutrient resource and decrease soil acidity, enhancing the decomposability of forb litter (Swift et al. 1979). Moreover, forbs show specific plant traits that are related to a faster decomposition rate, such as low C:N ratio and high specific leaf area (Cornelissen et al. 1999). Our analyses confirmed a much higher saccharidic C concentration (i.e. hemicellulose and amorphous and crystalline cellulose) in grass litter (~80%) than in forb litter (~60%). The former litter was also characterised by a higher C:N ratio (~50) than forb litter (C:N ~30) leading to a more recalcitrant litter. On the other hand, forb litter showed slightly more pronounced peaks in the 111–155 ppm and 156–165 ppm regions due to non-substituted aromatic rings and phenolic groups, respectively (Bonifacio et al. 2015). Forb litter was also richer (14.6%) in paraffinic C of lipid and waxes compared to grass litter (5.1%). According to this, Nardus, due to the compact architecture of its fast-growing tussocks, can produce and accumulate a large amount of low-quality litter in hummocks, inducing spatial heterogeneity and consequently enhancing the differentiation process of hummocks and hollows. Finally, we suggest that Nardus structure may also have had a role in decomposition processes, even though of lesser importance. Indeed, the elevated tussocks base of N. stricta have the potential to affect microclimatic conditions toward a much drier environment and directly slow down decomposition processes, as observed for Carex sempervirens in a subalpine grassland in the Central Alps (Yu et al. 2011).
In general the chemical changes in forb litter during decomposition involved a strong increase of N content that was positively correlated with paraffinic C content. This increase was much lower for grasses which also showed weaker oxidation signals. Several studies have reported that nutrient accumulation, especially N, may occur during decomposition (e.g. Berg and McClaugherty 2014; Koukoura et al. 2003) as a consequence of microbial growth on readily decomposable litter. Our observations suggest that microbial N immobilization occurred on forb litter, parallel to the degradation of saccharidic and aromatic moieties. The N increase with time together with the band at 1540 cm−1 in the FT-IR spectra, due to the relative increase of microbially-derived proteins, account for N assimilation by microbes, which used forbs as a C source initially and then also as nutrient source. On the other side, the chemical transformation and microbial colonisation (i.e. increase of paraffinic C and total N) of grass litter was less pronounced, but the correlation between N and saccharides content was higher and more significant compared to forb litter. This observation suggests that grass litter decomposition was more directional and focused on saccharidic C, suggesting that microorganisms might use grasses only as a source of carbon and energy. Comparing the decomposition pattern of forbs in the two microhabitats, we observed a clear decrease of saccharidic C in hummocks during winter that did not occur in hollows. According to Bragazza et al. (2007), we suggest that the differences in the qualitative changes of forb litter chemistry between hummocks and hollows might be the evidence of microbial differences in litter exploitation.
Conclusion
Decomposition is the key point of the feedback between soil properties and plant distribution. In our study system, hummock abiotic conditions slow down the decay of the recalcitrant litter produced by the dominant species N. stricta, which shows lower decomposition rates compared to forbs even in hollows. As a consequence of low decay rates, availability of soil nutrients remains low, allowing oligotrophic species to dominate. The same feedback occurs in hollows, with the opposite result: forbs dominating hollows produce more labile litter that decomposes faster in a more suitable microhabitat for decomposition, leading to a soil richer in nutrients where forbs can grow and dominate. These two feedback mechanisms enhance the differences between microhabitats and maintain the ecosystem heterogeneity.
References
Aerts R (1997) Climate, leaf litter chemistry and leaf litter decomposition in terrestrial ecosystems: a triangular relationship. Oikos 79:439–449
Almendros G, Dorado J, Gonzales-Vila FJ, Blanco MJ, Lankes U (2000) 13C NMR assessment of decomposition patterns during composting of forest and shrub biomass. Soil Biol Biochem 32:793–804
Anderson JM (1991) The effects of climate change on decomposition process in grassland and coniferous forests. Ecol Appl 1:326–347
Baer SG, Blair JM, Collins SL, Knapp AK (2004) Plant community responses to resource availability and heterogeneity during restoration. Oecologia 139:617–629
Baptist F, Yoccoz NG, Choler P (2010) Direct and indirect control by snow cover over decomposition in alpine tundra along a snowmelt gradient. Plant Soil 328:397–410
Beatty SW, Stone EL (1986) The variety of soil micro-sites created by tree falls. Can J For Res 16:539–548
Berg B, McClaugherty C (2014) Plant litter. Decomposition, humus formation, carbon sequestration, Third edn. Springer-Verlag, Berlin
Bonifacio E, Petrillo M, Petrell F, Tambone F, Celi L (2015) Alien red oak affects soil organic matter cycling and nutrient availability in low-fertility well-developed soils. Plant Soil 395:215–229
Bradshaw AD, Lodge RW, Jowett D, Chadwick MJ (1964) Experimental investigations into the mineral nutrition of several grass species: IV. Nitrogen Level J Ecol 52:665–676
Bragazza L, Alber R, Gerdol R (1999) Chemistry of pore water in hummocks and hollows in a poor mire in the southern Alps (Italy). WETLANDS 18:320–328
Bragazza L, Siffi C, Iacumin P, Gerdol R (2007) Mass loss and nutrient release during litter decay in peatland: the role of microbial adaptability to litter chemistry. Soil Biol Biochem 39:257–267
Britton AJ, Helliwell RC, Lilly A, Dawson L, Fisher JM, Coull M, Ross J (2011) An integrated assessment of ecosystem carbon pools and fluxes across an oceanic alpine toposequence. Plant Soil 345:287–302
Bryant DM, Holland EA, Seastedt TR, Walker MD (1998) Analysis of decomposition in alpine tundra. Can J Bot 76:1295–1304
Celi L, Schnitzer M, Nègre M (1997) Analysis of carboxyl groups in soil humic acids by a wet chemical method, Fourier-transform infrared spectrophotometry, and solution-state carbon-13 nuclear magnetic resonance. A comparative study. Soil Sci 162:189–197
Chadwick MJ (1960) Nardus stricta L. J Ecol 48:255–267
Cornelissen JHC (1996) An experimental comparison of leaf decomposition rates in a wide range of temperate plant species and types. J Ecol 84:573–582
Cornelissen JHC, Thompson K (1997) Functional leaf attributes predict litter decomposition rate in herbaceous plants. New Phytol 135:109–114
Cornelissen JHC, Pérez-Harguindeguy N, Díaz S, Grime JP, Marzano B, Cabido M, Vendramini F, Cerabolini B (1999) Leaf structure and defence control litter decomposition rate across species and life forms in regional floras on two continents. New Phytol 143:191–200
de Coulon J (1923) Nardus stricta. Étude physiologique, anatomique, et embryologique. Mem Soc vaud Sci nat 6:245–332
De Deyn GB, Cornelissen JHC, Bardgett RD (2008) Plant functional traits and soil carbon sequestration in contrasting biomes. Ecol Lett 11:516–531
Deák B, Valkó O, Török P, Kelemen A, Miglécz T, Szabó S, Szabó G, Tóthmérész B (2015) Micro-topographic heterogeneity increases plant diversity in old stages of restored grasslands. Basic Appl Ecol 16:291–299
Dwyer LM, Merriam G (1981) Influence of topographic heterogeneity on deciduous litter decomposition. Oikos 37:228–237
Elliott CL, Snyder H (1991) Autoclave-induced digestion for the colorimetric determination of silicon in Rice straw. J Agric Food Chem 39:1118–1119
Epstein HE, Burke IC, Lauenroth WK (2002) Regional Patterns of Decomposition and Primary Production Rates in the U.S. Great Plains. Ecology 83:320–327.
Eswaran H, van den Berg E, Reich P (1993) Organic carbon in soils of the world. Soil Sci Soc Am J 57:192–194
Galvagno M, Wohlfahrt G, Cremonese E, Rossini M, Colombo R, Filippa G, Julitta T (2013) Phenology and carbon dioxide source/sink strength of a subalpine grassland in response to an exceptionally short snow season. Environ Res Lett 8:025008
Gavazov KS (2010) Dynamics of alpine plant litter decomposition in a changing climate. Plant Soil 337:19–32
Gerola FM, Gerola DU (1951-1954) Ricerche sui pascoli delle Alpi centro-orientali. Le praterie dell’Altipiano di Asiago, Museo Storia Naturale Venezia Tridentina
Grab SW (2005) Aspects of geomorphology, genesis and environmental significance of earth hummocks (thúfur, pounus): miniature cryogenic mounds. Prog Phys Geogr 29:139–155
Güsewell S, Jewell PL, Edwards PJ (2005) Effects of heterogeneous habitat use by cattle on nutrient availability and litter decomposition in soils of an alpine pasture. Plant Soil 268:135–149
Hättenschwiler S, Tiunov AV, Scheu S (2005) Biodiversity and litter decomposition in terrestrial ecosystems. Annu Rev Ecol Evol Syst 36:191–218
Hook PB, Burke IC, Lauenroth WK (1991) Heterogeneity of soil and plant N and C associated with individual plants and openings in north American shortgrass steppe. Plant Soil 138:247–256
Jefferies RL, Walker NA, Edwards KA, Dainty J (2010) Is the decline of soil microbial biomass in late winter coupled to changes in the physical of cold soils? Soil Biol Biochem 42:129–135
Kögel-Knabner I (2000) Analytical approaches for characterizing soil organic matter. Org Geochem 31:609–625
Kögel-Knabner I (2002) The macromolecular organic composition of plant and microbial residues as inputs to soil organic matter. Soil Biol Biochem 34:139–162
Koukoura Z, Mamolos AP, Kalburtji KL (2003) Decomposition of dominant plant species litter in a semi-arid grassland. Appl Soil Ecol 23:13–23
Lavelle P, Blanchart E, Martin A, Spain A, Toutain F, Barois I, Schaefer R (1993) A hierarchical model for decomposition in terrestrial ecosystems: application to soils of the humid tropics. Biotropica 25:130–150
Leith H (1978) Pattern of primary productivity in the biosphere. Hutchinson & Ross, Stroudsberg
Miller NG, Alpert P (1984) Plant association and edaphic features of a high arctic mesotopographic setting. Arctic Alpine Res 16:11–24
Moser K, Ahn C, Noe G (2007) Characterization of microtopography and its influence on vegetation patterns in created wetlands. Wetlands 27:1081–1097
O’Lear HA, Seastedt TR (1994) Landscape patterns of litter decomposition in alpine tundra. Oecologia 99:95–101
Olson JS (1963) Energy storage and the balance of producers and decomposers in ecological systems. Ecology 44:323–331
Piccolo A, Stevenson FJ (1982) Infrared spectra of Cu2+ Pb2+ and Ca2+ complexes of soil humic substances. Geoderma 27:195–208
Pintaldi E, D’Amico ME, Siniscalco C, Cremonese E, Celi L, Filippa G, Prati M, Freppaz M (2016) Hummocks affect soil properties and soil-vegetation relationships in a subalpine grassland (North-Western Italian Alps). Catena 145:214–226
R Development Core Team. R (2005) A Language and Environment for Statistical Computing. R Foundation for Statistical Computing, Vienna, Austria. http://www.R-project.org
Raich JW, Schlesinger WH (1992) The global carbon dioxide flux in soil respiration and its relationship to vegetation and climate. Tellus 44B:81–99
Risch AC, Jurgensen MF, Frank DA (2007) Effects of grazing and soil micro-climate on decomposition rates in a spatio-temporally heterogeneous grassland. Plant Soil 298:191–201
Rose JP, Malanson GP (2012) Microtopographic heterogeneity constrains alpine plant diversity, glacier National Park, MT. Plant Ecol 213:955–965
Saccone P, Morin S, Baptist F, Bonneville JM, Colace MP, Domine F, Faure M, Geremia R, Lochet J, Poly F, Lavorel S, Clément JC (2013) The effects of snowpack properties and plants strategies on litter decomposition during winter in subalpine meadows. Plant Soil 363:215–229
Sanaullah M, Rumpel C, Charrier X, Chabbi A (2012) How does drought stress influence the decomposition of plant litter with contrasting quality in a grassland ecosystem? Plant Soil 352:277–288
Santonja M, Fernandez C, Gauquelin T, Baldy V (2015) Climate change effects on litter decomposition: intensive drought leads to a strong decrease of litter mixture interactions. Plant Soil 393:69–82
Schaller J, Roscher C, Hillebrand H, Weigelt A, Oelmann Y, Wilcke W, Ebeling A, Weisser WW (2016) Oecologia 18:277–286
Schlesinger WH, Reynolds JR, Cunningham JL, Huenneke LF, Jarrell WM, Virginia RA, Whitford WG (1990) Biological measurements feedbacks in global desertification. Science 247:1043–1048
Seastedt TR, Walker MD, Bryant DM (2001) Controls on decomposition processes in alpine tundra. In: Bowman WD, Seastedt TR (eds) Structure and function of an alpine ecosystem: Niwot ridge. Colorado. Oxford University Press, New York, pp 222–236
Sebastiá MT (2004) Role of topography and soils in grassland structuring at the landscape and community scales. Basic Appl Ecol 5:331–346
Seddon AWR, Macias-Fauria M, Long PR, Benz D, Willis KJ (2016) Sensitivity of global terrestrial ecosystems to climate variability. Nature 531:229–232
Sharp RP (1942) Soil structures in the St. Elias range, Yukon territory. Geomorphology 5:274–301
Socrates G (2004) Infrared and Raman characteristic group frequencies: tables and harts. John Wiley & Sons Ltd., England
Spaccini R, Mbagwu JSC, Conte P, Piccolo A (2006) Changes of humic substances characteristics from forested to cultivated soils in Ethiopia. Geoderma 132:9–19
Suttie JM, Reynolds SG, Batello C (2005) Grasslands of the world. FAO, Plant production and protection series, No. 34
Swift MJ, Heal OW, Anderson JM (1979) Decomposition in terrestrial ecosystems. University of California Press, Berkeley
Tilman D (1993) Species richness of experimental productivity gradients: how important is colonization limitation? Ecology 74:2179–2191
Tutin TG, Heywood VH, Burges NA, Moore DM, Valentine DH, Walters SM, Webb DA (1964–1980) Flora Europea. Cambridge University Press, Cambridge
Yu FH, Schütz M, Page-Dumroese DS, Krüsi BO, Schneller J, Wildi O, Risch AC (2011) Carex sempervirens tussocks induce spatial heterogeneity in litter decomposition, but not in soil properties, in a subalpine grassland in the Central Alps. Flora 206:373–379
Acknowledgements
A special thanks to Valentina Mello, Roberta Negri and Guido Teppa for their help during field work, to Marco Prati for his kind support in the lab, and to Compagnia San Paolo for funding my PhD project.
Author information
Authors and Affiliations
Corresponding author
Additional information
Responsible Editor: Cindy Prescott.
Rights and permissions
About this article
Cite this article
Oddi, L., Celi, L., Cremonese, E. et al. Decomposition processes interacting with microtopography maintain ecosystem heterogeneity in a subalpine grassland. Plant Soil 434, 379–395 (2019). https://doi.org/10.1007/s11104-018-3842-z
Received:
Accepted:
Published:
Issue Date:
DOI: https://doi.org/10.1007/s11104-018-3842-z