Abstract
The aim of the current study was to fabricate a pH-sensitive hydrogels for controlled release of Bovine serum albumin (BSA) protein. In this research work, polyvinylalcohol grafted poly(acrylic acid-co-2-acrylamido-2-methylpropane sulfonic acid) (PVOH-g-poly(AA-co-AMPS)) hydrogels with varying AA/AMPS molar ratio were synthesized through free radical graft copolymerization of partially neutralized acrylic monomers and characterized by Fourier transform infrared spectroscopy, Elemental analysis, X-ray diffraction, and Scanning electron microscopy, thermogravimetric analysis. Then, mechanical proprieties of hydrogels were investigated, and drug release behavior with Bovine serum albumin (BSA) as model drug was studied in vitro. The effect of AA/AMPS molar ratio, pH media on the swelling property of hydrogels was optimized. Results indicated that the strong interaction in the hydrogels resulted in the formation of a more stable copolymer. The high values of the grafting percentage which exceeds 800% confirmed the high performance of the grafting and the successful synthesis. Grafting AMPS onto PVOH/AA improved both thermal stability and mechanical properties of hydrogel. The hydrogel is simultaneous sensitive to pH and ionic strength; the swelling ratio maximizes at pH 6.8 medium and shrinks at pH 1.2, and decreases as the ionic strength increases; the swelling dynamic mechanism was explained well by Fickian diffusion and Schott’s pseudo-second-order models. Different kinetic models were applied, and it has been observed that release profile of BSA best followed the Hixson–Crowell for the release of drug in all release media. However, Zero order and Korsmeyer–peppas models only for pH 1.2 and pH 7.4. The swelling of the hydrogels and release of BSA from the drug loaded hydrogels occurred through non-Fickian diffusion mechanism. It is believed that this hydrogel could be potentially used for localized drug delivery.
Similar content being viewed by others
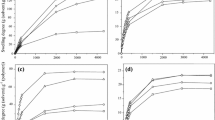
Explore related subjects
Discover the latest articles, news and stories from top researchers in related subjects.Avoid common mistakes on your manuscript.
Introduction
Superabsorbent polymers or hydrogels are defined as three-dimensional network structures that can hold large amounts of water or biological fluids even up to thousand times of their dry weight without dissolution [1, 2]. According to the formation mechanism, ionic charge and physical structure features, hydrogels can be roughly classified in a number of ways [3]. However, a special class of hydrogels, which are called ‘intelligent’ or stimuli-responsive hydrogels, go through reversible volume transitions in response to changes in temperature, pH, light, electric, salt concentration and ionic strengths, made it attractive in various fields [4].
In the last decades, the hydrogels have been applied in hygienic products, agriculture, industry, civil engineering. Nowadays, hydrogels are an appealing type of targeted drug delivery systems and have been used in many branches of medicine and biomedical engineering [5] including controlled drug and protein delivery, wound dressing, tissue engineering because of their outstanding properties including high swelling/deswelling ratio, non-toxicity, biocompatibility, and biodegradability [6]. Therefore, a variety of natural and synthetic polymers, such as starch [7], cellulose [8] agar [9], chitosan [10], alginate [11] polyvinyl alcohol [12], polyurethane [13], poly(ethylene glycol) [14], have been used to prepare polymeric hydrogels for biomedical application.
Polyvinyl alcohol (PVOH) is a cheap, biocompatible, nontoxic polymer, and due to its ease of film formation, water solubility, chemical stability, biodegradability, gel forming, and physical properties, and it is widely used for biomaterial and pharmaceutical applications such as protein/enzyme immobilization, wound dressing and contact lenses [6, 15]. In the other hand, pure polyvinylalcohol hydrogels are not sensitive to environmental stimuli for that it needs to combine the polyvinylalcohol with another polymer to produce a new kind of materials. One of the best methods for the synthesis of these materials is a chemical grafting of vinylic monomers such as 2-Acrylamido-2-methylpropane sulfonic acid and acrylic acid. Moreover, graft copolymerization is a technique, which improves the properties of natural and synthetic polymers and gives them a new property. On grafting, the host polymer gains some of the desired properties of the monomer used for grafting [16]. Overall, hydrogels derived from AMPS and acrylic acid exhibit pH-dependent swelling behavior.
Acrylic acid (AA) is a synthetic monomer, and the presence of carboxylic acid (–COOH) groups makes it responsive to the surrounding medium. The pKa value of poly (acrylic acid) is between 4.5 and 5.0, and thus, the hydrogels swell significantly at physiological pH due to ionization of the carboxylic acid groups [17]. Acrylic acid (AA) is a water-soluble polymer used mostly in stimuli-sensitive polymeric carrier systems, especially in pH-sensitive hydrogels. Due to its pH-sensitive nature, it has widespread biomedical and pharmaceutical applications as it delivers the maximum concentration of drug at a target site. Acrylic acid exhibits a maximum swelling index at basic medium due to deprotonation of functional groups (COOH) as compared to acidic medium and, therefore, more rapidly releases the drug at basic medium [18].
2-Acrylamido-2-methylpropane sulfonic acid (AMPS) is a vinyl monomer with a hydrophilic sulfonic acid group and a nonionic amide group. The sulfonic acid groups of AMPS have a high ionization constant (Ka = 2 × 10–1), and it was proven that the introduction of AMPS into the polymer networks could increase the swelling ratio, pH sensitivity, and salt resistance of hydrogel. AMPS is one of ionic hydrophilic monomer which plays a vital role in synthesis of hydrogels for drug delivery. Chondroitin sulfate-based hydrogels were prepared by the free radical polymerization technique for the controlled release of loxoprofen where AMPS was used as monomer and exhibited maximum swelling and drug release in both acidic and basic media [19].
In the past few years, the proteins are widely used as therapeutic agents in treatment of many diseases such as cancer, autoimmune and metabolic disorders. However, proteins have some drawbacks as physical and chemical instabilities, short half-life immune responses, and poor absorption efficiency through the gastrointestinal tract. Thus, protein drugs are traditionally administered by parenteral route rather than oral route. Consequently, many scientific research groups over the world have dedicated their efforts to prepare smart protein drug carriers that could solve the above-mentioned problems, and to control the protein release and also, to improve its therapeutic efficacy [20, 21]. Nowadays, pH-sensitive hydrogels have drawn extensive attention to design proteins oral delivery due to their capability to change their characteristics when exposing to external different pH media.
Bovine serum albumin (BSA) is a water-soluble natural albumin protein, and it has good features: abundance, low cost, biodegradability, biocompatibility, non-toxicity, non-immunogenicity, good stability and unusual ligand binding characters [20]. Furthermore, it is the most important protein in plasma and it represents 52–62% of total protein [22]. However, in the human body, BSA helps eliminate free radicals and deactivates the metabolism of several toxic lymphocytes [23], and it is the globular protein with distinct isoelectric point (pI) 4.7. Thus, the BSA molecules are carrying negative charge at physiological pH (pH 7.4) [22] and have the ability to bind especially strongly negatively charged support materials. For this reason, it takes an active role in immobilization studies [24].
In this study, a pH-sensitive hydrogel was synthesized by graft copolymerization of AA/AMPS onto polyvinylalcohol, using KPS as an initiator, and NMBA as cross-linker in an aqueous solution. The prepared hydrogels were characterized by FTIR, Elemental analysis, SEM, XRD and TGA. The effect of reaction parameters such as the mass ratio of AA to AMPS on the network structure and water absorbency was studied. Moreover, swelling behavior in different pH media, the deswelling and mechanical properties of the hydrogels were also studied. Finally, the drug loading and release studied were conducted using BSA as protein drug model.
Experimental
Materials
Polyvinylalcohol (PVA ou PVOH) powder (molecular weight 124,000 g/mol was obtained from Biochem. 2-Acrylamido-2-methylpropane sulfonic acid (AMPS) was purchased from Merck. Both products were used without purification. Acrylic acid (AA) was supplied from Biochem and used after purification by a purification column.
The initiator potassium persulfate (KPS) was purchased from Panreac. The cross-linking agent N,N’-Methylenebisacrylamide (NMBA) was supplied by Aldrich.
NaCl, KCl, CaCl2, MgCl2, AlCl3 were obtained from Panreac and used as received for the preparation of 0.9% saline solutions.
Potassium dihydrogen phosphate (KH2PO4), HCl (0.1 N), NaOH were purchased from Biochem and used for preparation of buffer solutions:
-
-Simulated gastric fluid (SGF, pH = 1.2) was prepared with mixing 2 g of NaCl in 11 ml of HCl (37%) and diluted up to 750 ml with distilled water. Intestinal fluid (SIF, pH = 6.8) was prepared by mixing 6.8 g of KH2PO4 and 0.92 g of NaOH in 1 L of distilled water. Phosphate buffer (PBS, pH = 7.4) was prepared from 250 ml of KH2PO4 (0.1 M) added to 195.5 ml of NaOH (0.1 M).
Distilled water was used for the preparation of all solutions.
BSA was chosen as a model molecule to characterize the release behavior from the hydrogels.
Synthesis of polyvinylalcohol-g-poly(AA-co-AMPS) hydrogels
Polyvinyl alcohol-g-poly(acrylic acid-2-acrylamido-2-methylpropane sulfonic acid) hydrogel was prepared through free radical polymerization method. At the first, polyvinylalcohol solution has been prepared; weighted amount of PVOH (2 g) was dissolved in distilled water (100 ml) at 80–90 °C for 1 h in a four necked flask equipped with a mechanical stirrer, a reflux condenser, and a thermometer, nitrogen line and then cooled to 60 °C. Secondly, a series of AA / AMPS with different molar ratios were neutralized in an ice bath with a 20 wt% NaOH solution and added into PVOH solution. Afterward, 195 mmol of NMBA and 208.26 mmol of KPS were added (Table.1) in the reaction mixture with vigorous stirring. Then, the reaction of polymerization was carried out at 75 °C for 2.5 h in the presence of N2 atmosphere. The synthesized hydrogels were immersed in acetone to remove water and unreacted monomers. Finally, the product was cut into disks about 3 mm thick and dried to constant weight at 40 °C [25].
Gel content
Gelation can be quantified by determining the amount of gel formed. After synthesis and obtaining the hydrogel, the weight of the hydrogel is measured before purification Wi, then the hydrogel is purified and dried and the final weight is measured Wd. The gel content of hydrogel was determined as follows [26]:
where \({\textit{Wd}}\) is the weight of purified and dried hydrogel, \( {\textit{Wi}}\) is the initial weight of hydrogel.
Grafting percentage and grafting efficiency
The grafting percentage (\(G\% )\) and the grafting efficiency (\(E\% )\) are calculated according to Eqs. (2) and (3), respectively [27].
where \(W0, W1, W2 \) denote the weight of polyvinylalcohol, final weight of the grafted hydrogel and weight of monomers, respectively.
Characterization
FTIR spectra were determined with a Thermo Scientific Nicolet IS 10 Model Spectrophotometer equipped with ATR Thermo Scientific Smart ITR module. Analysis was carried out with 40 scans and 2 cm−1 of resolution, in range of 4000–600 cm−1.
Elemental analysis was carried out by Vario Micro-Elementar (Allemagne). All the samples have been purified in acetone.
The X-ray diffraction (XRD) patterns of the hydrogels were recorded using a Panatical X’Pert Pro X-ray diffractometer. Scanning of samples was performed over the range of 5—50°, 2 \(\theta\) at a rate of 1°2 \(\theta\)/min.
Thermogravimetric analysis (TGA) was recorded using a Thermogravimetric Analyzer (SHIMADZU) TGA-51 Sat from 0 to 500 °C temperature range and with a heating rate of 20 °C/min under nitrogen atmosphere.
The surface morphology of the synthesized hydrogels was analyzed using scanning-electron microscopy (SEM). FEI-Quanta 650 (w) Tungsten at 05 kV voltage acceleration. The composition of the sample was further analyzed with X-ray energy-dispersive analysis system (EDS). Dried samples were cut into optimum size particles than coated with gold to enhance conductive of the hydrogels for SEM observation.
Tensile test of all samples was measured using a 10 KN universal testing machine (Zwick Roell 8306) at a uniaxial stretching speed of 100 mm/min. The size of cylindrical sample was 13 mm in diameter and 10 mm in length.
Compression experiment was performed on a 10KN testing machine (Zwick Roell—8306). The cylindrical samples of 12–20 mm2 (diameter–height) were placed on the lower plate and compressed by the upper plate at a compression speed of 20 mm/min. The tests of all samples were conducted in triplicate.
Swelling behavior
The swelling behavior of the hydrogels was investigated in excessive distilled water. For this purpose, weighted amount of dried gels was kept in water at 37 °C, hydrogels were removed from water at regular time intervals, and the weight of hydrogels was measured after removing the surface water with a filter paper, and then placed in the same bath. This process was continued until a constant weight was achieved. The equilibrium swelling can be calculated by using the following equation [28]:
where M0 and Mt are the initial mass and mass at different time-intervals, respectively.
pH-sensitivity study
To study the pH effect on the swelling of hydrogels, we prepared aqueous solutions of different pH in the range [1.2–13] using standard solutions: HCl (0.1 M) and NaOH (0.1 M), a sample of 0.1 g of hydrogel is soaked in 100 ml of each solution at 37 °C for 24 h. Swelling ratio is determined using formula.4 cited in “Swelling behavior” [18, 27, 29].
Deswelling behavior
The hydrogels were swollen in distilled water and in buffer solution pH = 6.8 at 37 °C until equilibrium state, and then, both hydrogels were transferred into acidic solutions (pH = 1.2) to study their deswelling.
The following equation can be used to estimate the deswelling degree of hydrogels [30].
where \({\textit{Wt}}\) is the total mass of the hydrogels that are measured at a time interval and \({\textit{Ws}}\) and \({\textit{Wd}}\) are the weight of swollen hydrogels in the swollen and dried state, respectively.
Effect of ionic strength on absorption capacity
For the purpose of studying the effect of ionic strength on absorption capacity of polyvinylalcohol-g-poly(AA-co-AMPS), a weighed quantity (0.1 g) of the hydrogels is submerged in 100 ml of various saline solutions NaCl, KCl, CaCl2, MgCl2 and AlCl3 at 0.9 wt%. The absorption capacity of each solution is determined according to the above methods described in “Swelling behavior” [29, 31, 32].
Drug loading and release
Drug loading
The hydrogels disks were steeped in 100 ml of drug solution (2 g/bovine serum albumin (BSA) in 1L of biological solution) for 48 h at 37 °C. After, the loaded disks were taken out and dried at 40 °C. The drug loading capacity was calculated using the following equation [33]:
where \(V1\) is the volume of initial drug solution (100 ml), \(C1\) is the initial concentration of the drug solution (2.10−3 g/ml), \(V\) is the remaining volume of the drug solution (ml) after loading of hydrogel, \( C\) is the concentration of remaining drug solution (g/ml) after loading of hydrogel, The concentrations of BSA were determined using UV–Visible spectrophotometer (Thermo Scientific—Evolution 201) at 280 nm. And \({\text{Wd}}\) is the weight of the dry hydrogel (g) after drug loading.
Biological solutions are: SGF (pH 1.2), SIF (pH 6.8) and PBS solutions (pH 7.4).
Drug release
In vitro release of BSA from the drug loaded hydrogels was carried out in buffer solutions of pH 6.8, 7.4, 1.2 at 37 °C and 150 rpm. After 24 h of incubation in shaking incubator, the samples (3 ml) were withdrawn at periodic intervals and were immediately replaced by the same volume of fresh medium.
The drug concentration in the hydrogel sample was determined using a UV–Vis spectrophotometer at 280 nm, and the cumulative percent drug release of the hydrogel was calculated using Eq. 7 [34].
where \(V\) and \({\textit{Vi}}\) are the volume of release medium (40 ml) and the volume of release medium removed (3 ml), respectively, m is a hydrogel weight (mg), \({\textit{Cn}}\) and Ci are the initial drug concentration (2 mg/ml) and drug concentration in release medium, respectively. All the absorbance values at different times were measured three times.
Results and discussion
Synthesis mechanism of polyvinylalcohol-g-poly(AA-co-AMPS)
The polyvinylalcohol-g-poly(AA-co-AMPS) hydrogel with varying (AMPS/AA) molar ratio was synthesized by graft copolymerization of acrylic acid and 2-Acrylamido-2-methyl-1-propanesulfonic acid onto polyvinylalcohol in the presence of NMBA as a cross-linking agent and KPS as free radical initiator. Firstly, the persulfate initiator is decomposed under heating to generate sulfate anion-radicals. The radicals extract hydrogen from the hydroxyl group of the polyvinylalcohol to form alkoxy radicals on the substrate. The monomer molecules, which are in close vicinity of the reaction sites, become acceptor of polyvinylalcohol radicals resulting in chain initiation and thereafter themselves become free radical donor to neighboring molecules. In this way, grafted chain grows. Since a cross-linking agent NMBA is presented in the system, the end vinyl groups of cross-linker NMBA may react synchronously with polymer chains during the chain propagation. The copolymer is comprised of a cross-linked structure. The plausible proposed mechanism for the synthesis of polyvinylalcohol-g-poly(AA-co-AMPS) hydrogels is given in Fig. 1.
Gel content, grafting percentage and grafting efficiency
The three test parameters: gel content (Gel%), grafting percentage (\(G\% ),\) grafting efficiency \(\left( {E\% } \right)\), are calculated according to Eqs. (1), (2), and (3), respectively. The results represented in Table 2 indicate that the gel content increase proportionally with the increase in quantity of AMPS, because the introduction of AMPS monomer in the reaction medium causes its copolymerization with acrylic acid which increases the cross-linking of the polymer thanks to its ionic groups and subsequently the increase in molecular weight of the latter, which increases the gel content. In the other hand, the high values of the grafting percentage (\(G\% ),\) which exceeds 800% and the grafting efficiency (\(E\% )\) which exceeds 70% confirmed the high performance of the grafting and the successful synthesis, and these two parameters also increase proportionally with the increase in the rate of AMPS monomer; this is due to the increase in molecular weight of the hydrogel after grafting due to the copolymerization of AMPS with acrylic acid and its fixing onto polyvinylalcohol..
FT-IR spectral analysis
The structure of polyvinylalcohol hydrogels was confirmed by FTIR analysis, as shown in Fig. 2.
The spectrum of pure polyvinylalcohol gives a broad absorption band in the range of 3014–3635 cm−1 is assigned to the presence of O–H stretching vibrations of polyvinylalcohol and water. The peak at 2921 cm−1 is attributed to asymmetric –CH2 group stretching vibration. While peak at 1413 cm−1 corresponds to deformation of hydroxyl groups –OH. The absorption peak at 1083 cm−1 is related to C–O– typical secondary alcohols. The absorption peak at 833 cm−1 is attributed to C–C stretching vibration [35].
In the spectrum of the hydrogel polyvinylalcohol-g-poly(AA), a new peak appeared at 1764 cm−1 corresponded to –C=O of carboxylic acid groups of acrylic acid. And the peaks at 868 cm−1 are due to deformation OH groups of carboxylic acid. Another bands at 1513 and 1476 cm−1 corresponded to symmetric and asymmetric R–COO−, respectively, of AA, –OH groups in the PVOH form ether bonds with PAA, which consequently reduces the content of –OH groups and appearance of the peak at 1234 cm−1 corresponded de C–O–C ether group. The peak at 2955 cm−1 is due to C–H symmetric stretching vibration. These results confirmed the successful grafting of acrylic acid onto the polyvinylalcohol chains [17].
On comparing the FTIR spectra of polyvinylalcohol, polyvinylalcohol-g-poly(AA) and polyvinylalcohol-g-poly(AA-co-AMPS) hydrogel, it has been observed that the spectrum of PVOH-g-poly(AA-co-AMPS) shows variation intensity and shifting of an absorption band in the range of 3696–3096 cm−1 due to O–H stretching of water and N–H amide. The peak at 1185 cm−1 is assigned to C–O–C ether group. The vibration band observed at 2927 cm−1 is ascribed to the –CH2 –group on the polymeric chain. The peak at 1648 cm−1 represented the stretching vibration of C=O amide. While the double peaks at 1299 and 1042 cm−1 were ascribed to the asymmetric and symmetric vibration of S=O, respectively, which were the characteristic peaks of AMPS. However, the characteristic peak at 1552 cm−1 is attributed to the vibration of N–H and that at 1450 cm−1 is the vibration of C–N [36, 37]. These results confirmed introduction of AMPS onto hydrogel structure and the successful cross-linking of reactant.
Further confirmation of the composition hydrogels, polyvinylalcohol-g-poly(AA-co-AMPS) was conducted with elemental analysis. The result showed that the measured mass percent of the element C/H/N /S/O was 34.44%, 10.06%, 2.83%, 1.36% and 40.40%, respectively.
Thermal analysis
The TGA curves of hydrogels are shown in Fig. 3. The thermogravimetric analysis showed that the thermal decomposition for PVOH-g-poly(AA) hydrogel follows the following stage: the weight loss about 45% within the temperature of 397.36 °C. However, for the hydrogels PVOH-g-poly(AA-co-AMPS) with molar ratios of AA/AMPS: 80/20, 65/35, 50/50 and 20/80 the 45% weight loss correspond to 397.04 °C, 452, 455 °C and 458 °C, respectively. Moreover, the results have indicated that the thermal stability of hydrogel with 20/80 seemed a little higher than other hydrogel. It can be concluded that the grafting of AMPS onto polyvinylalcohol-g-poly(AA) backbone enhances the thermal stability, which suggests that the grafted hydrogel is synthesized successfully [27].
X-ray diffraction (XRD) analysis
Figure 4 shows the X-ray diffraction patterns of polyvinylalcohol, polyvinylalcohol-g-poly(AA) and polyvinylalcohol-g-poly(AA-co-AMPS) at different ratio of AMPS. The X-ray diffraction pattern of polyvinylalcohol shows two peaks at 2θ = 19.48° and 2θ = 22.60°; the latter corresponds to its crystalline structure. Whereas, polyvinylalcohol start loss part of its crystallinity when the acrylic acid is grafted into polyvinylalcohol. The PVOH-g-poly(AA-co-AMPS) losses its crystallinity because crystal degree of polyvinylalcohol chains is reduced through breaking down of inter chain hydrogel bonding along the polyvinylalcohol chains.
Morphological SEM and EDS analysis
The SEM micrograph of the cryogenically fractured surfaces of the PVOH-g-poly(AA-co-AMPS) hydrogel prepared with different AMPS/AA molar ratio is shown in Fig. 5. The homogeneous structure and a porous and coarse structure are clearly observed which confirmed the grafting. Porosity increased with increasing of AMPS content; the high content of AMPS could promote the hydrogel to form the more uniform and dense network structure. It can be seen that the pore size of the hydrogel decreased with increasing AA/AMPS molar ratio and the porous structure became more uniform and dense, less compact which confirmed the less crystallinity of hydrogel seen by XRD analysis.
The hydrogel (80/20) presented a porous structure with large pore size and non-uniform distribution, while in hydrogel (20/80), the pore shrank which resulted from the high grafting percentage with AMPS.
Figure 6 indicates the EDS analysis of PVOH-g-poly(AA-co-AMPS) corresponding to the surface of samples shown in Fig. 5. The EDS spectrum shows the existence of the S.N and Na elements which confirmed that AA/AMPS were grafted onto polyvinylalcohol hydrogel.
Mechanical behavior
Polyvinylalcohol-g-poly(AA-co-AMPS) hydrogels exhibit excellent mechanical properties that can withstand various deformations. Fig. 7 illustrates photographs of hydrogels; it was observed that the hydrogels show their ability to withstand stretching (a), knotting (b) and compression (c). However, it was notable that the hydrogels were able to recover its original shape without obvious damages; these phenomena indicate that the hydrogels have a certain shape recovery property. Then, the hydrogels were investigated by tensile and compression tests. The stress–strain and tensile-strain curves of PVOH-g-poly(AA-co-AMPS) hydrogels are obtained and shown in Fig. 8 with various AA/AMPS ratios. The curves indicate that for the hydrogels 80/20, the tensile strength and elongation at break are 25Kpa and 159.63 mm/mm, respectively. Then, by increasing the AMPS content, tensile strength of hydrogels is always increasing while the elongation at break increases and then decreases. The tensile strength of PVOH-g-poly(AA-co-AMPS) with 80% of AMPS content maximum 216 Kpa and its elongation at the breaks is 147 mm/mm. Hydrogel with 50/50 molar ratio has maximum elongation at the breaks 200.63 mm/mm. For compressive properties, it is observed that the compressive strength of PVOH-g-poly(AA-co-AMPS) increases and then, decreases as the AMPS ratio increases; the compressive strength of hydrogel with 65/35 of AA/AMPS is the maximum and could reach 284 Kpa at stain of 100 mm/mm. These results may be due to the formation of strong hydrogen bonds between the hydroxyl groups of polyvinylalcohol and carboxyl groups of polyacrylic acid chains, and sulfonate groups of AMPS, which can significantly improved mechanical properties of the hydrogel networks.
Swelling properties
The water absorbency depends on the solvent nature, network density, and interaction of polymer–solvent parameter [30].
Mass ratio of AA to AMPS
The effect of monomers ratio on water absorbency is shown in Fig. 9. The results indicate that increasing AMPS ratio in (AA/AMPS) improved the absorbance capacity of hydrogels and hydrogel with ratio 65/35 gives the maximum absorption capacity. The increase in AMPS content from 0 to 35 increases the swelling ability of hydrogels due to the high hydrophilicity of the synthesis hydrogel, which caused by the increase in hydrophilic groups such as –CONH and –SO3H, and the synergistic effect produced by –CONH and –SO3H of AMPS together with that of –COOH on AA increase. While, water absorbency decreased when the AMPS amount increased from 50 to 80 in respect that the electrostatic repulsion between ions weakened and the three-dimensional network compacted; this may be due to the high grafting rate of (AA-AMPS) on polyvinylalcohol. In addition, as AMPS contained quaternary carbon atoms –SO3H groups which were enormous in size, stretching of the polymer chains would be obstructed and absorbency would decrease accordingly [29].(Fig. 10).
Effect of the ionic strength on water absorbency
The swelling capacity of ionic hydrogels is significantly affected by various factors of external solution such as charge valencies and salt concentration [29]. The swelling of hydrogels in salt solutions NaCl, KCl, CaCl2, MgCl2 and AlCl3 with the concentration of 0.9% shows that the swelling ratio clearly decreased comparing to the distilled water. This well-known phenomenon commonly observed in the swelling of ionic hydrogels [31] that is often attributed to a charge screening effect of the additional cations causing a non-perfect anion–anion electrostatic repulsion led to a decreased of difference osmotic pressure ( ionic pressure) between the hydrogel network and the external solution [29, 38].
Table 3 depicts the effect of cation charge on swelling; it can be seen that the water absorbency of PVOH-g-poly(AA-co-AMPS) in the saline solution from high to low k+ > Na+ > Ca+2 > Mg+2 > Al+.3 While, the ionic strength of isotonic salt solution is Al+3 > Mg+2 > Ca+2 > Na+ > k+. The effect of the ionic strength on the swelling can be expressed by Flory’s equation [39]:
where Q is the degree of swelling, i/Vu is the charge density of polymer, S is the ionic strength of solution, \(\left( {1/2\, - \,x1} \right)/V1\) is the polymer–solvent affinity, \({\text{VE/V0}}\) is the cross-linking density.
Results show that water absorbency decreased with increasing of the ionic strength of saline solutions, so the experimental results obey Flory’s equation. However, the swelling ratio increases when the content of AMPS to AA is high, and the swelling capacity of these hydrogels in salt solutions was not decreased; this anti-salt behavior is due to the presence of high ionization tendency and law salt sensitivity characteristics of lots of sulfonate groups in AMPS parts [32]. As can be seen, the swelling strongly depends on the type of salt solution. It was found that the swelling in salt solution was decreased considerably in the order of monovalent, divalent, and trivalent; that is may be expressed by complexing ability arising from the coordination of trivalent and bivalent cations with hydrogels groups [32, 40].
Effect of pH on equilibrium swelling ratio
Studies have indicated that water absorption of hydrogels is sensitive to environmental pH, and it indicates that ionic hydrogels exhibit swelling under various pHs, ranged from 1.2 to 13 [18]. So, the pH effect on the equilibrium swelling behaviors of PVOH-g-poly(AA-co-AMPS) hydrogel was investigated. As displayed in Fig. 11, the swelling ratio of hydrogels in acidic pHs (pH ≤ 4) is lower than that in alkaline solution; because the most of the carboxylate (–COO−) and sulfonate anions (–SO3−) are protoned. Therefore, the hydrogen bonding between –COOH, SO3H and OH was strengthened and additional physical cross-linking was generated. Hence, the network tends to shrink and as result of which equilibriums swelling were low [26, 29]. With increasing pH (9 > pH > 5), some of carboxylate and sulfonate groups are converted to –COO− and -SO3−, and the hydrogen bonding interaction was broken. Then, the electrostatic repulsion between –COO− and –SO3− caused an enhancement of the swelling capacity [26] and reaches the maximum value 4715.2 g/g (20/80) at pH 6.8. At high pHs (13 > pH > 8), the swelling capacity of hydrogels significantly decreases due to the charge screening of excess Na+ in the solution, which shields the carboxylate and sulfonate anions and prevent effective anion–anion repulsion [41].
Models of swelling dynamic mechanism
The swelling kinetics of hydrogel can be summarized into two stages: water molecules enter the internal gaps of the hydrogel molecules under the osmotic pressure, and then, the polymer chains disperse and expand after absorbing the water.
The swelling mechanism in distilled water was studied by using Fickian diffusion model (Eqs. 9, 10) and Schott's second-order-kinetic model (Eq. 11) which can be expressed as:
where \(F\) is the fractional active at given time \(t\) (hour), \(Q_{t} \) and \(Q_{w}\) are the weight of water absorbed at time \(t\) and equilibrium, respectively. \( K\) is the characteristic constant of the hydrogels and \(n\) is the diffusion index-linked to transport manner of infiltration, three theoretical modes can be assumed to the limit of \(n\).
Case I: \(n <\) 0.5 Fickian diffusion is leading, which can be governed by the transport power of concentration gradient.
Case II: 0.5 < \(n <\) 1 non-Fickian diffusion, where the water uptake is controlled collaboratively by water diffusion and relaxation of polymer chains.
Case III: \(n\) ≥ 1 anomalus diffusion; the stretch and extension of polymer chains would control the system [42].
The determined kinetics parameters are listed in Table 4. As shown, the n values were in the range of 0.5 ~ 1.0, with good linear correlation coefficient (R2 > 0.99), indicating its easier relaxation of polymer chains for the water accommodation the domination of non-Fickian diffusion model.
The swelling process of the hydrogels could be successfully described by the Schott’s pseudo-second-order kinetics model. Results showed a good linear coefficient (R2 > 0.99).
Deswelling properties in acidic solution
For the deswelling measurements in acidic solution as mentioned above, the hydrogels were swollen in distilled water and in simulated intestinal fluid pH 6.8, and then, they were transferred into acidic solution (pH 1.2). It is clearly seen from Fig. 12a that with increasing in AMPS content, the desweling rate of all hydrogels swollen in distilled water increases, and tended to lose more than 80% of water in pH 1.2 for (20/80).
A results represented in Fig. 12b indicate that the hydrogel without AMPS content, swollen in simulated intestinal fluid pH 6.8, has a faster deswelling and reduced about 80% in pH 1.2, while with increasing of AMPS content, the deswelling rate decrease (20% for 20/80, 30% (50/50), 35% (65/35) 70% (80/20)). This behavior at pH1.2 makes the PVOH-g-poly(AA-co-AMPS) as a suitable candidate for designing controlled drug delivery [43]; because release of water-soluble drugs from hydrogel involves water penetration and swelling of hydrogel, and further dissolution and diffusion of drugs [44].
The pH reversibility
The synthesized hydrogel PVOH-g-poly(AA-co-AMPS) exhibits different swelling behaviors at various pH value at 37 °C; we investigate the reversibility swelling- deswelling in aqueous solutions adjusted at pH 6.8 and pH 1.2 buffer solutions, respectively. The time interval between the pH changes was 24 h. Thus, in pH 6.8 the network structure of the hydrogel expands because of the repulsion of similar charges (COO−, SO3−) in its structure resulting in extensive swelling in water. While at pH 1.2, it shrink due to protonation of carboxylate and sulfonate groups. Fig. 13 proves that PVOH-g-poly(AA-co-AMPS) hydrogels show excellent pH stability even after four reputed cycles of swelling–deswelling behavior. The sharp on–off switching behavior makes them as a smart material for controlled drug delivery.
Drug loading and release
The drug loading and release behavior of hydrogels were studied in simulated gastric and intestinal fluids, and the BSA was used as a model drug in this study. Drug loading capacity of the hydrogels is shown in Table 5. It can be seen that the loading capacity of PVOH-g-poly(AA-co-AMPS) was determined in the range from 186.49 to 361.42 mg/g, 218.24 to 369.07 mg /g and 248.91 to 363.47 mg/g after 48 h for pH = 1.2, 6.8 and 7.4, respectively.
Results of drug release of hydrogels at different times are represented in Fig. 14. It can be seen that the drug release pattern for all hydrogels tested can be divided into two phases; the first shows high initial release due to loosely attached BSA molecules on the surface of the hydrogels. The hydrogels showed a rapid burst, releasing in the first five hours 53.88% in SGF (pH 1.2), 26.86 in SIF (pH 6.8) and 38.19% in PBS (pH 7.4) of the drug. The second phase is the continuous drug release process, the later attaint 95% for (20/80) and 85% for 65/35 in SGF (pH 1.2) after 10 h, 30% for (20/80) and 50% for 65/35 in SIF (pH 6.8), and 80% for (20/80) and 75% for 65/35 in PBS (pH 7.4). These drug loading and release performance clearly indicate that the rate of release of BSA from the hydrogels depends on the amount of drug concentration and drug load of hydrogel and composition of hydrogels, swelling and deswelling. The drug release increase when the swelling and the desweling rate of hydrogels in distilled water increase. Also, polyvinylalcohol- g-poly(AA-co-AMPS) hydrogel gives a better release rate than polyvinylalcohol-g-poly(acrylic acid) hydrogel. Therefore, the AMPS plays an important role in the release mechanism of BSA as it improves their swelling, and the best release medium is gastric pH = 1.2.
These results could be attributed to the higher swell ability and more pores structure of polyvinylalcohol-g-poly (AA-co-AMPS) hydrogels than polyvinylalcohol-g-poly(acrylic acid) hydrogel [45].
Kinetic of drug release
To better understand the release mechanism of drug molecules from the synthesized hydrogel, it is necessary to fit the release data into a suitable mathematical models named as Zero-order model (Eq. 12), first-order model (Eq. 13), Higuchi model (Eq. 14), Korsmeyer–Peppas model (Eq. 15) and Hixson–Crowell model (Eq. 16) [34].
where: \( \frac{{{\text{Mt}}}}{M\infty } \) is cumulative fraction of drug release, t is the release time, n represents drug release exponent, \(Q0\) is the amount of drug loaded in hydrogel, \({\text{Qt}}\) is amount of drug released at time t and k0 (mg/h), k1 (h−1), k2 (h−1/2), k3 (h−n), and k4 (h−1/3) are release rate constants.
The release data were plotted according to the equations of relevant models. The best model for each formulation was selected on the basis of regression coefficient (R2) value near to 1 as shown in Table 6. Results indicate that BSA was released following Hixson–Crowell kinetic model in all release media. However, it has been observed that drug release from hydrogels followed by Zéro-order and Korsmeyer–Peppas models only for pH 1.2 and 7.4. Whereas, the release rate constant depends upon the initial concentration of drug within the matrix and also upon the nature of hydrogels [46]. The diffusional exponent ‘n’ value for Korsmeyer–Peppas was between 0.67 and 1.28 suggesting a non-Fickian or anomalous diffusion for all hydrogel. Conclusively, the drug release mechanism kinetics is both diffusion and polymer relaxation controlled.
Conclusion
pH-sensitives hydrogel-based polyvinyl alcohol-g-poly(AA-co-AMPS) were successfully prepared by graft copolymerization and chemical cross-linking with methylene bis-acrylamide, and they were evaluated as controlled drug delivery of bovine serum albumin (BSA). The structure of this pH-sensitive hydrogel was confirmed by FTIR and elemental analysis. The XRD patterns indicate that the crystallinity of PVOH-g-poly(AA-co-AMPS) is lower than polyvinylalcohol. The TGA revealed that the thermal stability of hydrogels was improved with the grafting of AMPS onto polyvinylalcohol-g-poly(acrylic acid). Characterization by SEM spectroscopy indicates that the surface morphology of hydrogel has a coarse and porous microstructure. Mechanical properties demonstrated that mechanical strength was enhanced by increasing the amount of AMPS, so PVOH-g-poly(AA-co-AMPS) hydrogels exhibit excellent mechanical properties that can withstand various deformations.
PVOH-g-poly(AA-co-AMPS) super-absorbents are ionized hydrogels that their swelling capacity depends on the properties of the physical and chemical structure and the medium. However, the swelling and deswelling kinetics of these hydrogels in water, acidic solution were investigated at 37 °C. Besides, swelling behavior in different conditions shows that hydrogel is pH and ionic strength sensitive. The swelling ratio of the hydrogel in distilled water is increased by increasing the molar ratio of AMPS from 00 to 35 due to the electrostatic repulsion between ions weakened. Also, the swelling ratio decreases with the increase in ionic strength of salt solution. The hydrogel shows a higher swelling ratio in pH value from pH 6.8 to 7.4 resulting from –COO− and -SO3− groups on the polymers chains.
Furthermore, the hydrogels exhibited remarkable stretch ability, deformation recoverability and reversible swelling behaviors.
Finally, BSA loading onto hydrogels mainly depends on the swelling behavior of the hydrogel; higher swelling degree suggests larger amount of BSA adsorbed. So, the swelling and release of drug occurred through non-Fickian diffusion mechanism. It has been found that the hydrogel with high drug loading efficiency displayed faster and higher release rate than that of hydrogel containing a smaller amount of drug. Also, PVOH g-poly(AA-co-AMPS) hydrogel gives a better release rate than PVOH-g-poly(acrylic acid) hydrogel. Therefore, the AMPS plays an important role in the release mechanism of BSA, and the best release medium is gastric pH = 1.2. The release kinetics of the prepared systems followed Hixson–Crowell kinetic.
The aim of the present work is to add a pH-sensitive hydrogel with better performance to the wide range of biomaterials available for controlled drug release.
References
Bounabi L, Mokhnachi NB, Haddadine N, Ouazib F, Barille R (2016) Development of poly(2-hydroxyethyl methacrylate)/clay composites as drug delivery systems of paracetamol. J Drug Delivery Sci Technol 33:58–65. https://doi.org/10.1016/j.jddst.2016.03.010
Mahinroosta M, Jomeh Farsangi Z, Allahverdi A, Shakoori Z (2018) Hydrogels as intelligent materials: a brief review of synthesis, properties and applications. Mater Today Chem 8:42–55. https://doi.org/10.1016/j.mtchem.2018.02.004
Kalagasidis Krušić M, Ilić M, Filipović J (2009) Swelling behaviour and paracetamol release from poly (N-isopropylacrylamide-itaconic acid) hydrogels. Polym Bull 63:197–211. https://doi.org/10.1007/s00289-009-0086-3
Das R, Pal S (2013) Hydroxypropyl methyl cellulose grafted with polyacrylamide: application in controlled release of 5-amino salicylic acid. Colloids Surf B 110:236–241. https://doi.org/10.1016/j.colsurfb.2013.04.033
Bordbar-Khiabani A, Gasik M (2022) Smart hydrogels for advanced drug delivery systems, review. Int J Mol Sci 23:1–23. https://doi.org/10.3390/ijms23073665
Gholamali I, Asnaashariisfahani M, Alipour E (2020) Silver Nanoparticles Incorporated in pH-sensitive nanocomposite hydrogels based on carboxymethyl chitosan-poly (Vinyl Alcohol) for use in a drug delivery system. Regen Eng Transl Med 6:138–153. https://doi.org/10.1007/s40883-019-00120-7
Elvira C, Mano JF, San Román J, Reis RL (2002) Starch-based biodegradable hydrogels with potential biomedical applications as drug delivery systems. Biomaterials 23:1955–1966. https://doi.org/10.1016/S0142-9612(01)00322-2
Muchová M, Münster L, Capáková Z, Mikulcová V, Kuřitka I, Vícha J (2020) Design of dialdehyde cellulose crosslinked poly(vinyl alcohol) hydrogels for transdermal drug delivery and wound dressings. Mater Sci Eng C 116:211–242. https://doi.org/10.1016/j.msec.2020.111242
Olak T, Turan A, Alpaslan D, Dudu TE, Aktaş N (2020) Developing poly (Agar-co-Glycerol-co-Thyme Oil) based organo-hydrogels for the controlled drug release applications. J Drug Delivery Sci Technol 60:88–102. https://doi.org/10.1016/j.jddst.2020.102088
Yang J, Chen J, Pan D, Wan Y, Wang Z (2013) pH-sensitive interpenetrating network hydrogels based on chitosan derivatives and alginate for oral drug delivery. Carbohydr Polym 92:719–725. https://doi.org/10.1016/j.carbpol.2012.09.036
Apoorva A, Rameshbabu AP, Dasgupta S, Dhara S, Padmavati M (2020) Novel pH-sensitive alginate hydrogel delivery system reinforced with gum tragacanth for intestinal targeting of nutraceuticals. Int J Biol Macromol 147:675–687. https://doi.org/10.1016/j.ijbiomac.2020.01.027
Rac V, Lević S, Balanč B, Olalde Graells B, Bijelić G (2019) PVA Cryogel as model hydrogel for iontophoretic transdermal drug delivery investigations. comparison with PAA/PVA and PAA/PVP interpenetrating networks. Colloids Surf B 180:441–448. https://doi.org/10.1016/j.colsurfb.2019.05.017
Cherng JY, Hou T, Shih MF, Talsma H, Hennink WE (2013) Polyurethane-based drug delivery systems. Int J Pharm 450:145–162. https://doi.org/10.1016/j.ijpharm.2013.04.063
Wu J, Wei W, Wang L-Y, Su Z-G, Ma G-H (2007) A thermosensitive hydrogel based on quaternized chitosan and poly(ethylene glycol) for nasal drug delivery system. Biomaterials 28:2220–2232. https://doi.org/10.1016/j.biomaterials.2006.12.024
Anwar H, Ahmad M, Minhas MU, Rehmani S (2017) Alginate-polyvinyl alcohol based interpenetrating polymer network for prolonged drug therapy, optimization and in-vitro characterization. Carbohydr Polym 166:183–194. https://doi.org/10.1016/j.carbpol.2017.02.080
Mohamed SF, Mahmoud GA, Taleb MFA (2013) Synthesis and characterization of poly(acrylic acid)-g-sodium alginate hydrogel initiated by gamma irradiation for controlled release of chlortetracycline HCl. Monatsh Chem 144:129–137. https://doi.org/10.1007/s00706-012-0776-7
Ullah K et al (2019) Facile synthesis of chitosan based-(AMPS-co-AA) semi-IPNs as a potential drug carrier: enzymatic degradation, cytotoxicity, and preliminary safety evaluation. Curr Drug Deliv 16:242–253. https://doi.org/10.2174/156720181566618102415210
Suhail M, Fang CW, Minha MU (2021) Preparation, characterization, swelling potential and in-vitro evaluation of sodium poly (styrene sulfonate)-based hydrogels for controlled delivery of ketorolac tromethamine. Pharmaceuticals 14:350. https://doi.org/10.3390/ph14040350
Suhail M, Khan A, Rosenholm JM, Minhas MU (2021) Fabrication and characterization of diclofenac sodium loaded hydrogels of sodium alginate as sustained release carrier. Gels 7:1–16. https://doi.org/10.3390/gels7010010
Abu Elella MH, Magdy W, Sabaa D, Hanna H, Abdel-Aziz MM, Mohamed RR (2020) Antimicrobial pH-sensitive protein carrier based on modified xanthan gum. J Drug Delivery Sci Technol 57:101. https://doi.org/10.1016/j.jddst.2020.101673
El-Sherbiny I, Khalil I, Ali I, Yacoub M (2017) Updates on smart polymeric carrier systems for protein delivery. Drug Dev Ind Pharm 43:1567–1583. https://doi.org/10.1080/03639045.2017.1338723
Çalımlı MH, Demirbaş Ö, Aygün A, Alma MH, Nas MS, Şen F (2018) Immobilization kinetics and mechanism of bovine serum albumin on diatomite clay from aqueous solutions. Appl Water Sci 8:209–215. https://doi.org/10.1007/s13201-018-0858-8
Varnier K, Vieira T, Wolf M, Belfiore LA, Tambourgi EB, Paulino AT (2018) Polysaccharide-based hydrogels for the immobilization and controlled release of bovine serum albumin. Int J Biol Macromol 120:522–528. https://doi.org/10.1016/j.ijbiomac.2018.08.133
Kudelski A (2003) Influence of electrostatically bound proteins on the structure of linkage monolayers: adsorption of bovine serum albumin on silver and gold substrates coated with monolayers of 2-mercaptoethanesulphonate. Vib Spectrosc 33:197–204. https://doi.org/10.1016/j.vibspec.2003.09.003
Meng Y, Ye L (2017) Synthesis and swelling property of the starch-based macroporous superabsorbent. J Appl Polym Sci 134:88–99. https://doi.org/10.1002/app.44855
Fan L, Yang H, Yang J, Peng M, Hu J (2016) Preparation and characterization of chitosan/gelatin/PVA hydrogel for wound dressings. Carbohydr Polym 146:427–434. https://doi.org/10.1016/j.carbpol.2016.03.002
Mohammad N, Atassi Y, Tally M (2017) Synthesis and swelling behavior of metal-chelating superabsorbent hydrogels based on sodium alginate-g-poly (AMPS-co-AA-co-AM) obtained under microwave irradiation. Polym Bull 74:4453–4481. https://doi.org/10.1007/s00289-017-1967-5
Bashir S, Teo YY, Ramesh S, Ramesh K (2018) Synthesis and characterization of karaya gum-g-poly (acrylic acid) hydrogels and in vitro release of hydrophobic quercetin. Polym 147:108–120. https://doi.org/10.1016/j.polymer.2018.05.071
Bao Y, Ma J, Li N (2011) Synthesis and swelling behaviors of sodium carboxymethyl cellulose-g-poly (AA-co-AM-co-AMPS)/MMT superabsorbent hydrogel. Carbohydr Polym 84:76–82. https://doi.org/10.1016/j.carbpol.2010.10.061
Yu Z, Liu J, He H, Ma S, Yao J (2021) Flame-retardant PNIPAAm/sodium alginate/polyvinyl alcohol hydrogels used for fire-fighting application: Preparation and characteristic evaluations. Carbohydr Polym 255:117–128. https://doi.org/10.1016/j.carbpol.2020.117485
Zhao Y, Su H, Fang L, Tan T (2005) Superabsorbent hydrogels from poly (aspartic acid) with salt-, temperature-and pH-responsiveness properties. Polym 46:5368–5376. https://doi.org/10.1016/j.polymer.2005.04.015
Mirdarikvande S, Sadeghi H, Godarzi A, Alahyari M, Shasavari H, Khani F (2014) Effect of pH, and Salinity onto swelling properties of hydrogels based on H-alginate-g-poly (AMPS). Biosci Biotechnol Res Asia 11:205–209. https://doi.org/10.13005/bbra/1256
Jeong D, Kim H, Jeong J, Dindulkar S, Cho D, Y.-H.Yang E, Jung S (2016) Cyclosophoraose/cellulose hydrogels as an efficient delivery system for galangin, a hydrophobic antibacterial drug. Cellulouse 23:2609–2625. https://doi.org/10.1007/s10570-016-0975-1
Duru Kamacı U, Kamacı M (2020) Preparation of polyvinyl alcohol, chitosan and polyurethane-based pH-sensitive and biodegradable hydrogels for controlled drug release applications. Inter J Polym Mater Polym Biomater 69:1167–1177. https://doi.org/10.1080/00914037.2019.1670180
Mahdavinia GR, Soleymani M, Etemadi H, Sabzi M, Atlasi Z (2018) Model protein BSA adsorption onto novel magnetic chitosan/PVA/laponite RD hydrogel nanocomposite beads. Inter J Biol Macromol 107:719–729. https://doi.org/10.1016/j.ijbiomac.2017.09.042
Zhu L, Zhang L, Tang Y (2012) Synthesis of montmorillonite/poly (acrylic acid-co-2-acrylamido-2-methyl-1-propane sulfonic acid) superabsorbent composite and the study of its adsorption. Bull Korean Chem Soc 33:1669–1674. https://doi.org/10.5012/bkcs.2012.33.5.1669
Hui B, Ye L (2016) Structure of polyvinyl alcohol-g-acrylic acid-2-acrylamido-2-methyl-1-propanesulfonic acid hydrogel and adsorption mechanism for advanced Pb (II) removal. J Ind Eng Chem 35:309–317. https://doi.org/10.1016/j.jiec.2016.01.010
Sadeghi M, Yarahmadi M (2011) Synthesis of a novel pH- and salt-responsive super absorbent hydrogel based on collagen-g-poly(AA-co-IA). Orient J Chem 27:453–460
Lanthong P, Nuisin R, Kiatkamjornwong S (2006) Graft copolymerization, characterization, and degradation of cassava starch-g-acrylamide/itaconic acid superabsorbents. Carbohydr Polym 66:229–245. https://doi.org/10.1016/j.carbpol.2006.03.006
Zheng Y, Li P, Zhang J, Wang A (2007) Study on superabsorbent composite XVI. synthesis, characterization and swelling behaviors of poly (sodium acrylate)/vermiculite superabsorbent composites. Eur Polym J 43:1691–1698. https://doi.org/10.1016/j.eurpolymj.2007.02.023
Kalaleh H.-A, Tally M, Atassi Y( 2013) Preparation of a clay based superabsorbent polymer composite of copolymer poly (acrylate-co-acrylamide) with bentonite via microwave radiation. arXiv preprint arXiv:1311.6445
Chen M, Ni Z, Shen Y, Xiang G, Xu L (2020) Reinforced swelling and water-retention properties of super-absorbent hydrogel fabricated by a dual stretchable single network tactic. Colloids Surf A Physicochem Eng Asp 602:125133. https://doi.org/10.1016/j.colsurfa.2020.125133
Angar N-E, Aliouche D (2018) Deswelling of hydrogels in aqueous and polyethylene glycol solutions. a new approach for drug delivery application. Period Polytechn Chem Eng 62:137–143. https://doi.org/10.3311/PPch.11132
Singh B, Bala R (2014) Polysaccharide based hydrogels as controlled drug delivery system for GIT cancer. Inter J Biol Macromol 65:524–533. https://doi.org/10.1016/j.ijbiomac.2014.02.004
Nochos A, Douroumis D, Bouropoulos N (2008) In vitro release of bovine serum albumin from alginate/HPMC hydrogel beads. Carbohydr Polym 74:451–457. https://doi.org/10.1016/j.carbpol.2008.03.020
Sethi S, Kaith BS, Kaur M, Sharma N, Kumar V (2020) Cross-linked xanthan gum–starch hydrogels as promising materials for controlled drug delivery. Cellulose 27:4565–4589. https://doi.org/10.1007/s10570-020-03082-0
Acknowledgements
This work was supported by the University M’hamed Bouguara, Boumerdes-Algeria, and we thank our colleagues from the university who provided insight that greatly assisted the research. Mr.Hamza Senoussaoui (Laboratory of soft Technologies, Valorization, Physicochemistry of Biological Materials and Biodiversity) is gratefully acknowledged for providing some partial supports.
Author information
Authors and Affiliations
Corresponding author
Ethics declarations
Conflict of interest
The authors declare that they have no known competing financial interests or personal relationships that could have appeared to influence the work reported in this paper.
Additional information
Publisher's Note
Springer Nature remains neutral with regard to jurisdictional claims in published maps and institutional affiliations.
Rights and permissions
Springer Nature or its licensor (e.g. a society or other partner) holds exclusive rights to this article under a publishing agreement with the author(s) or other rightsholder(s); author self-archiving of the accepted manuscript version of this article is solely governed by the terms of such publishing agreement and applicable law.
About this article
Cite this article
Hocine, S., Ghemati, D. & Aliouche, D. Synthesis, characterization and swelling behavior of pH-sensitive polyvinylalcohol grafted poly(acrylic acid-co-2-acrylamido-2-methylpropane sulfonic acid) hydrogels for protein delivery. Polym. Bull. 80, 12591–12617 (2023). https://doi.org/10.1007/s00289-022-04664-7
Received:
Revised:
Accepted:
Published:
Issue Date:
DOI: https://doi.org/10.1007/s00289-022-04664-7