Abstract
Abiotic stressors may affect biogenic volatile organic compounds (BVOCs) involved in plant communication. We examined how certain environmental conditions affect plant signaling via BVOC emission. Specifically, we investigated the effects of elevated CO2 and temperature in situ on BVOCs produced by mechanically damaged leaves of Centaurea solstitialis L. (yellow starthistle), a major invasive weed in western North America, grown in grassland plots in the foothills of northern California. The headspace BVOCs of C. solstitialis were collected in situ by a customized Teflon® bag and solid-phase microextraction and analyzed by gas chromatography–mass spectroscopy. Damaging leaves led to the release of 14 volatile compounds, predominantly sesquiterpenes. The co-occurrence of five compound pairs was highly significant across all treatments, which may be explained through synthesis by the same enzyme. We found no significant effect of treatment on the levels of individual or total volatiles. The stability of volatile profile for this invasive under future conditions should therefore (1) not alter indirect defense signaling and (2) support the selection of biological controls on the basis of their specificity to the identified in-field host plant BVOCs.
Similar content being viewed by others
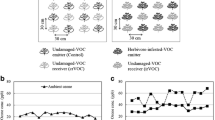
Explore related subjects
Discover the latest articles, news and stories from top researchers in related subjects.Avoid common mistakes on your manuscript.
Introduction
Global climate change is expected to increase in the current century and is shifting environment dynamics, invasive species abundance, and plant–insect communication (McFrederick et al. 2009; IPCC 2013a). Ecosystem response to climate change driven abiotic stressors is occurring in both terrestrial and marine communities around the world (IPCC 2014b). The consequences of anthropogenic CO2 and rising temperatures are affecting ecosystems and community composition (IPCC 2014a). Species restricted to a certain range have seen a dramatic range reduction, and due to recent climate change, have been the first to experience whole species extinctions (Beever et al. 2003; Descimon et al. 2005). Such stressors have disrupted synchronicity between interacting species at the flowering and breeding interface (Visser and Both 2005).
Because environmental changes involve multiple factors that may occur simultaneously, it is important to examine the potential for inhibitory or promoting effects of CO2 and temperature stressors both individually and in concert in the field. Such factors alter plant physiological properties, modifying herbivore consumption and influencing plant community composition (Peters et al. 2006). Generally, elevating mean temperature increases biomass production in natural systems in colder regions, either by speeding up metabolism or extending the growing season (Rustad et al. 2001). Increasing atmospheric CO2 often leads to increased net primary production and above-ground biomass (Norby et al. 2005; Field et al. 2007), though not always (Shaw et al. 2002; Norby et al. 2010). In general, increased decomposition offsets some or all of the increase in net primary production (van Groenigen et al. 2014). Focusing treatments on specific plant species in the field will complement work at the community level and provide further insight.
Invasive plant species continue to threaten ecosystem biodiversity and stability both regionally and globally (Mooney and Drake 1986; James et al. 2010; Groom et al. 2014). Their adaptive flexibility along with their ability to competitively exclude and displace native species has allowed them to flourish in new environments (Mooney and Cleland 2001). The growth of invasives could be favored under climatic change by increasing expansion rates through their inherent temperature tolerance and adaptability to extreme weather events (Dukes and Mooney 1999; Bradley et al. 2010; Wheeler and Schaffner 2013). Identifying specific factors that facilitate invasive success, such as elevated CO2, is key (Dukes 2000; Verlinden et al. 2014).
Yellow starthistle (YST), Centaurea solstitialis L. (Asteraceae), a winter annual native to the Mediterranean region, has established in North America and covers over 7 million hectares in at least 23 states. YST is most widespread in California (Wilson et al. 2003; DiTomaso et al. 2006; Pitcairn et al. 2006). This invasive weed is highly competitive and its thorny spines around the flowerhead are detrimental to grazing cattle. The establishment of YST in North America coincides with recent rapid increase of CO2 levels (Wilson et al. 2003). In a 40-year time period (1960–2000), atmospheric CO2 has increased by approximately 50 ppm, during which YST expansion grew exponentially from 1 to 14 million acres (IPCC 2014a; Pitcairn et al. 2006). CO2 positively affects YST growth. The invasive grew up to six times larger under elevated CO2 in grassland plots (Dukes et al. 2011). It also has great potential for displacing native species and reducing biodiversity, making effective biological control a top priority (Van Driesche et al. 2008).
Biogenic volatile organic compounds (BVOCs) provide multiple ecological functions (Tholl et al. 2006) where sesquiterpenes can figure prominently in some plant–insect signaling (Yuan et al. 2009). BVOC responses can also include plant communication during herbivory or abiotic stress responses such as heat and oxidative pressure (Sharkey et al. 2001; Dudareva et al. 2006; Peñuelas and Staudt 2010). No broad conclusive patterns have been demonstrated for climate change effects on plant direct defense via BVOCs (Peñuelas and Staudt 2010). However, a plant’s BVOC response depends on multiple factors such as the degree of temperature increase, time of day, season, plant species examined (Yuan et al. 2009), and method of collection (Beck et al. 2014). Several studies have detected and identified a suite of BVOCs emitted from YST (Buttery et al. 1986; Binder et al. 1990; Beck et al. 2008; Smith and Beck 2013). Buttery and Binder originally collected and examined ex situ volatiles from YST plants and detected compounds that may be unique to harvested plant parts and thus will not be discussed (Buttery et al. 1986; Binder et al. 1990). Yellow starthistle studies discussed in this paper have found mechanical leaf damage to generate a broad profile of volatile compounds (Beck et al. 2008; Smith and Beck 2013). A future comparison of methodology, plant part, and corresponding volatiles may be of benefit.
Volatiles induced during leaf herbivory, such as sesquiterpenes, attract natural predators and parasitoids by signaling that a plant is being consumed (Turlings et al. 1990; Dicke et al. 1990; Vet and Dicke 1992; Heil 2004). This plant–herbivore–carnivore interaction involving plant volatiles is known as indirect defense (Price et al. 1980; Dudareva et al. 2006). For YST, this defense mechanism may play a crucial role during the early growth stages when physical defense is low. During this time, gastropod herbivores such as Deroceras reticulatum (gray garden slug) have been shown to consume nearly half of above ground production (Peters et al. 2006). D. reticulatum feeding on young YST elicits BVOC emissions attractive to the predatory ground beetle Pterostichus melanarius (Oster et al. 2014). This dynamic between a natural control, its host, and predator may change if environmental conditions such as temperature and CO2 are altered (Thompson 2005; Wheeler and Schaffner 2013).
Studying indirect defenses through plant volatile response to abiotic stressors, such as climate change conditions, is a relatively unique approach. This is the first known report of the volatile composition of C. solstitialis grown under climate change treatments in the field. Our aims were to (1) determine if YST growth under elevated CO2 or temperature is associated with a change in BVOC emissions, thereby influencing plant–insect signaling, (2) compile a broad BVOC profile emitted from damaged YST, and (3) add to a growing volatile library which will assist in the selection of species-specific biological controls for invasive weeds.
Materials and methods
YST growth site
Plants were grown in the Jasper Ridge Global Change Experiment located in the Jasper Ridge Biological Preserve of Stanford University near the eastern foothills of the Santa Cruz Mountains (Latitude: 37.40524|Longitude: −122.2421). The Global Change Experiment explores responses of grassland to four environmental factors: warming, elevated CO2, increased precipitation, and increased nitrogen deposition. The study site contains 32 circular plots two meters in diameter, divided into four randomly distributed treatment quadrants exposed to ambient or elevated CO2 (additional 300 ppm for a total of 700 ppm, or twice the ambient level), elevated N (additional 7 g N per m2 per year), elevated precipitation (adding half of ambient rainfall shortly after the event), and elevated temperature (temperature raised an approximate 3 °C with infrared heaters) with all 16 possible combinations interspersed among the global change landblocks (Fig. 1). These treatments reflect a mid-range scenario expected globally for the twenty-first century (IPCC 2013b). The effects of two factors (temperature only, CO2 only, and temperature and CO2 combined) and an ambient control group on YST were studied.
Seedling growth and transfer
Seeds from wild YST collected from Jasper Ridge were germinated in November on petri dishes for 7 days and transferred to growth trays, which were placed into the greenhouse for 8 days with final transfer to the Global Change Experiment at 17 days after germination (December 11th, 2012). Seedlings were planted into established grassland in each quadrant (ambient, temp, CO2, temp + CO2) and allowed to grow until rosette stage. Beginning in April 2013, seedlings were given 20 ml of water once a week until 1 week prior to sampling.
Volatile sampling
The in situ volatile collection experiments were performed as described by Beck et al. (2008) and Smith and Beck (2013). Plants were sampled for volatiles upon reaching uniform physiological maturity, i.e. when reaching the rosette growth stage. Plants were sampled from ambient plots (N = 8), elevated CO2 plots (N = 15), elevated temperature (N = 9), elevated CO2 and temperature combined (N = 23); additional damaged plants were utilized for further compound verification via a second gas chromatography–mass spectroscopy (GC–MS) column. The 2-month sampling time spanned June 7th–August 7th. Sampling took place between 6:00 and 9:00 each morning to capture volatiles during a time of natural herbivore activity and to prevent condensation in the volatile collection medium at higher ambient temperatures. Prior to volatile collection, each plant was punctured a total of 30 times (leaves only) with a B-D 16G syringe needle. Control experiments were performed to determine the background volatile profiles of plants with undamaged leaves (N = 6) and empty bags (N = 6) using the same collection protocol described. Leaf puncture was chosen for consistency in damage amounts.
A 10.2 × 25.4 cm customized Teflon® bag (Welch Fluorocarbon, Dover, New Hampshire; heat sealed on three sides, with two Kynar portals) (Beck et al. 2008) was utilized for volatile collection. A solid-phase microextraction (SPME, Supelco, Bellefonte, PA; 100 μm, polydimethylsiloxane fiber) needle was inserted through the port septum and allowed to adsorb volatiles for an exposure period of 2 h (Fig. 2). At the end of exposure time, the fiber was retracted into the SPME and removed from the outlet port. The SPME was placed in an individual Kimax® 70 ml culture tube, sealed with a stopper, and tightly wrapped with Parafilm® sealing film. The tube was placed in a 3.79-L Ziploc® bag and stored upright in ice and transported to the lab for analysis. Storage time of the fiber prior to injection time onto the GC ranged between 2 and 8 h. All SPME cartridges remained in the cooler until injection time.
Volatile analysis
Collected volatiles were thermally desorbed on either a J & W Scientific (Folsom, CA) DB-Wax or DB-1 column (60 m × 0.32 mm i.d. × 0.25 μm) on a HP-6890N gas chromatograph (GC) coupled to HP-5975B mass selective detectors (MSD) (MS; Palo Alto, CA). Desorbed volatiles were analyzed using methods previously published (Beck et al. 2008; Smith and Beck 2013). NIST, Wiley, and internally generated databases were used for fragmentation pattern identification. The retention indices (RIs) were calculated using a homologous series of n-alkanes on DB-Wax and DB-1 columns. Volatile identifications were verified by injection of commercially available or isolated laboratory standard samples for comparison to retention times and fragmentation patterns while tentatively identified compounds were verified from comparison of RI values to elution from two different columns.
Statistical analysis
To test the presence of a relationship between treatment and peak relative abundance of both individual volatiles and the total sum of volatiles within each treatment, we used a non-parametric multivariate ANOVA: Manly’s method of unrestricted permutation (Manly 2007) and Edgington’s method of restricted permutation (Edgington and Onghena 2007). We performed a linear regression to test for the effect of sampling period on the relative abundance of each compound. Presence of compound co-occurrence was calculated with the Spearman correlation coefficient in R-project using the rcorr function.
Results
The in situ volatile emission profile of damaged C. solstitialis comprised 14 compounds of which 13 were sesquiterpenes (incl. two unidentified) and one was an alkene (Table 1). The compound germacrene D had a relative abundance greater than all other compounds by more than one order of magnitude (Fig. 3). Even without controlling for multiple testing, both Manly’s and Edgington’s methods revealed no significant relationship between treatment and relative abundance of volatiles either individually or as a total signal (P > 0.05). In-field control experiments of undamaged plants detected no volatile compounds, lending support to either trace level presence below detection limits in the field or an absence of volatiles for undamaged YST (the latter seen by Beck et al. 2008 for YST and its sister species). There were four compounds not detected in the ambient treatment but present in other treatments: α-humulene, present in both CO2 treatments; β-bisabolene, present in the CO2 and temperature treatments; β-sesquiphellandrene, only present in the temperature treatment; and 1,5,9-trimethyl-1,5,9-cyclododecatriene, present in the temperature and interaction treatments. However, the compounds were only detected for a few samples in these treatments; therefore, the treatments did not show a significant effect on the presence/absence of the compounds using a multivariate logistic regression. Using the full sample, multiple volatile compounds were significantly correlated (Spearman’s correlation coefficient (corr) >0.5, based on a conservative significance level of 0.0001). Five compound pairs exhibited highly significant co-occurrence (corr > 0.75): germacrene D with bicyclogermacrene (corr = 0.87), germacrene D with β-bourbonene (corr = 0.82), germacrene D with β-caryophyllene (corr = 0.80), β-caryophyllene with β-bourbonene (corr = 0.78), and β-bourbonene with an unknown sesquiterpene with RI of 1574 (corr = 0.76), as seen in Fig. 4.
Correlation of volatile compounds. Gradient legend shows Spearman correlation coefficient (corr) value; corr > 0.5 shows significance (P value <0.0001). Five compound pairs (circled) exhibited highly significant co-occurrence (corr > 0.75): germacrene D with bicyclogermacrene (corr = 0.87), germacrene D with β-bourbonene (corr = 0.82), germacrene D with β-caryophyllene (corr = 0.80), β-caryophyllene with β-bourbonene (corr = 0.78), and β-bourbonene with an unknown sesquiterpene with RI of 1574 (corr = 0.76)
Discussion
Treatment effects
The absence of significant treatment effects of elevated temperature and CO2 on volatile emission in YST may be the result of both a species-specific and terpene-specific response. In addition, natural environmental conditions acting as limiting factors and abiotic stressors may have also influenced emission. Different plant species display substantial variability in sesquiterpene emission even under similar treatment conditions (Duhl et al. 2008). Generally, elevated temperature tends to positively affect BVOC emission by increasing diffusion and enhancing enzymatic activity (Guenther et al. 1993). However, Mediterranean species such as yellow starthistle tend to be water rather than temperature limited (Monson et al. 2007; Fortunati et al. 2008; Llusià et al. 2008, 2009) and, under conditions of water stress, their terpene emissions have been shown to decrease (Vallat et al. 2005; Monson et al. 2007; Omeno 2007). Thus, either the temperature treatment did not influence volatile emission or the dry growing season experienced in this study may have acted in compensation to a possible elevation in emissions due to the temperature treatment, resulting in an apparently conserved emission signal.
CO2 concentration in the atmosphere is vital for plant carbon fixation and sequestration and one of the many effects of its elevation is often increased standing biomass, when acting as a limiting resource (Körner 2006; Peñuelas and Staudt 2010). Although it has been suggested that such productivity may increase BVOC synthesis and emission (Lerdau et al. 1994) in light of the carbon-nutrient balance hypothesis (Gershenzon 1994; Waterman and Mole 1989), there is conflicting evidence (Yuan et al. 2009). In fact, terpenoid emissions have not only been seen to decrease under elevated CO2, pointing to inhibited terpene synthase activity (Loreto et al. 2001; Rosenstiel et al. 2003), but have also shown no significant effects (Constable et al. 1999). In the latter case, a steady state of emission per unit biomass may be maintained due to lower stomatal density on the resulting larger-grown leaves (Woodward et al. 2002; Vuorinen et al. 2004). While elevated CO2 provides additional carbon for the production of secondary metabolites, terpene production is cost intensive and requires sufficient nutrients for the production of biosynthetic enzymes and storage structures (Gershenzon 1994). In fact, the naturally poor soil quality and the absence of fertilization in the field result in lower volatile emissions than from plants grown in nutrient rich greenhouse soil (Gouinguené and Turlings 2002). Additionally, terpene production may not only depend on carbohydrate or substrate supply, but also on terpene synthase enzyme activity (Chappell et al. 1991; Iijima et al. 2004). Hence, the BVOC emission response under elevated temperature and CO2 in yellow starthistle may be limited by precipitation and nutrient conditions in the field and may, therefore, not be directly coupled to the strong growth response of YST observed under elevated CO2 in other studies (Dukes et al. 2011).
Many of the existing studies have been performed under artificial conditions, yet free air CO2 enrichment (FACE) experiments are very limited (Yuan et al. 2009). Therefore, the complex dynamic of biotic and abiotic factors in the field may dampen the effects of treatment, providing a more accurate picture of their collective influence. Plants under laboratory conditions grow without competition for space, light, water, etc.—all of which in the field may represent a resource more limiting than carbon and of stronger effect than the treatment itself (Körner 2006).
Volatile compounds
In response to mechanical damage, field-grown YST released an array of volatiles, dominated by sesquiterpenes (Table 1; Fig. 3)—compounds known for their semiochemical activity in plants (Yuan et al. 2009). The absence of other volatiles, such as green leaf volatiles (GLVs), may be attributed to a species-specific response as well as to plant growth conditions: both Beck et al. (2008) and Smith and Beck (2013) also found a major presence of sesquiterpenes with only trace or minor levels of GLVs and monoterpenes. The most abundant volatile from all treatments was germacrene D, followed by β-bourbonene and bicyclogermacrene. These three compounds have been identified as semiochemicals in other systems (Weissbecker et al. 2000; Light and Knight 2005; Sidney et al. 2006; Ghirardo et al. 2012). The compounds α-gurjunene and the two unknown sesquiterpenes had the lowest relative abundance. Terpene production is a regulated response and plants may only emit enough volatiles to elicit a reaction from predators (Tholl 2006).
Sesquiterpenes are from the terpenoid family group comprised of three isoprene units and are synthesized by the mevalonate (MVA) pathway in the cytosol (Lichtenthaler 1999), possibly utilizing universal terpenoid intermediates from the parallel methylerythritol phosphate (MEP) pathway located in the plastid (Hemmerlin et al. 2012). Regulation of terpenoid biosynthesis is generally spatial and temporal. Spatially, sesquiterpene biosynthesis can be sequestered in glandular structures often found on leaves while temporally, volatile emission and enzyme activity have been shown to peak and decline during plant growth (Gershenzon et al. 1989; Grinspoon et al. 1991; McGarvey and Croteau 1995). A study examining terpenoid biosynthesis found that suites of enzymes are responsible for terpenoid formation in ginger and turmeric. In fact, nearly all sesquiterpenes identified by Koo and Gang (2012) were found to be produced by multiple terpene synthases, including eight of the thirteen sequiterpenes detected in our study. For example, one terpene synthase produced β-caryophyllene and 1,5,9-trimethyl-1,5,9-cyclododecatriene, in addition to α-humulene as a major product, while three enzymes produced both β-caryophyllene and germacrene D (Koo and Gang 2012).
Multiple pairs of compounds correlated across all treatments including the ambient treatment. The volatiles germacrene D and bicyclogermacrene had the highest correlation (corr = 0.87). These two compounds are candidates as signals in indirect defense based on their presence in slug-damaged YST (Oster et al. 2014). Both compounds belong to the germacrane family of sesquiterpenes (McMurry and Bosch 1985; Bülow and König 2000). Additionally, the third strongest co-occurring pair (corr = 0.80), germacrene D and β-caryophyllene, has been shown to be synthesized by the same enzymes in other plants (Koo and Gang 2012). Therefore, compound co-occurrence may be explained by synthesis from the same terpene synthases in general (Bohlmann et al. 1998; Steele et al. 1998). In fact, the formation of multiple products by a single terpene synthase is often seen in this enzyme family (Chen et al. 2003; Tholl et al. 2005) and may be due to the production of reaction intermediates with multiple metabolic destinies (Crock et al. 1997).
Several of the BVOCs emitted by YST in response to damage under simulated global changes are associated with indirect defense in other species. Colorado beetle-damaged potato plants emit β-caryophyllene, α-humulene, trans-β-farnesene, bicyclogermacrene, and germacrene D. These compounds are implicated in indirect defense by attracting the predaceous stinkbug Perillus bioculatus (Weissbecker et al. 2000). In other indirect defense systems, α-copaene attracts predatory mites to spider-mite-infested lima bean plants (Dicke 2009). The compounds trans-β-farnesene and α-copaene have been shown to arise from the same precursors during biosynthesis in grape berries (May et al. 2013). The eulophid wasp parasitoid of elm leaf beetle eggs is attracted to a trans-β-farnesene and α-humulene containing mixture released by egg-infested elms (Büchel et al. 2011). Finally, α-gurjunene, β-bisabolene, and β-sesquiphellandrene possess insect repellent properties in low doses (Wang and Kays 2002; Conti et al. 2011; Ray et al. 2012). Several of the detected sesquiterpenes have been reported as pheromones from various aphid species. For instance, trans-β-farnesene and α-gurjunene are minor components of Brevicoryne brassicae, and germacrene D, β-bourbonene, and α-copaene comprise the blend of pheromones of Euceraphis punctipennis (Francis et al. 2005).
Four of the compounds detected here were also observed in our earlier study using slug-damaged plants: trans-β-farnesene, germacrene D, bicyclogermacrene, and 1,5,9-trimethyl-1,5,9-cyclododecatriene (Oster et al. 2014). The compound trans-β-farnesene may play a role in indirect defense by attracting the predatory P. melanarius ground beetle to its slug prey (Oster et al. 2014). This compound has been shown to attract P. melanarius in other studies and is the main constituent of the alarm pheromone emitted from its aphid prey (Kielty et al. 1996). A compound of note was the detection of 1,5,9-trimethyl-1,5,9-cyclododecatriene. Reports of this unique sesquiterpene as a plant volatile are limited. One investigation lists it as a component of water lily flowers (Yuan et al. 2014) and a second investigation detects a minor amount of the compound in ginger and turmeric tissues (Koo and Gang 2012). No reports of semiochemical activity of this compound have been published to date.
Eight of the compounds detected in this experiment were in the portfolio for damaged YST described by Beck and Smith (who found a similar number of total volatiles): α-copaene, α-gurjunene, β-caryophyllene, trans-β-farnesene, α-humulene, germacrene D, bicyclogermacrene, and pentadecene (Beck et al. 2008; Smith and Beck 2013; Beck et al. 2014). The detected alkene, pentadecene, possesses a wide range of activities. It acts as a trail pheromone for seed harvesting ants (Co et al. 2003) and is also found in beetle sex pheromones (Keville and Kannowski 1975). Additionally, pentadecene is a major component in volatiles emitted from the buds and flowers of plants in the Centaurea genus upon damage (Senatore et al. 2003).
The other six compounds, all sesquiterpenes, were not detected in the previous YST studies and include β-bourbonene, two unknowns, β-bisabolene, β-sesquiphellandrene, and 1,5,9-trimethyl-1,5,9-cyclododecatriene. Most notable was the relatively large amount of β-bourbonene produced in the current study and across all treatments, yet not detected by Smith and Beck (2013). Since β-bourbonene was detected in the ambient treatment, the only possible explanation for its appearance includes either the age of the plant (our study sampled younger plants than Smith and Beck (2013) or its growth under field conditions. Both β-bourbonene and α-copaene are reported pheromones for the European birch aphid (Francis et al. 2005) and β-bourbonene has exhibited semiochemical behavior for Lepidoptera (Sidney et al. 2006). The large amount of the sesquiterpene detected and the limited, but strong evidence for semiochemical activity in other systems warrant further investigation of this compound. Non-identical experimental conditions may contribute to these differences. Smith and Beck (2013) performed their experiment under lab conditions whereas our collection was performed in the field. In addition, year to year climate variation has been documented for this region (Zavaleta et al. 2003; Dukes et al. 2011); our experimental year experienced little precipitation and a dry spring.
Centaurea solstitialis is a dominant invasive weed that poses a growing challenge to land managers, farmers, and grassland diversity in North America (Wilson et al. 2003; DiTomaso et al. 2006). The stability in volatile profile should not directly affect YST control during early growth by its chief herbivore and recruitment of predators via indirect defense. Thus, for natural control purposes, attention should be given to monitoring both herbivore and predator populations during early growth of this weed, while supporting the selection of biological controls on the basis of their specificity to the identified in-field host plant BVOCs.
References
Beck JJ, Smith L, Merrill GB (2008) In situ volatile collection, analysis, and comparison of three Centaurea species and their relationship to biocontrol with herbivorous insects. J Agric Food Chem 56:2759–2764
Beck JJ, Smith L, Baig N (2014) An overview of plant volatile metabolomics, sample treatment and reporting considerations with emphasis on mechanical damage and biological control of weeds. Phytochem Anal 25:331–341
Beever EA, Brussard PF, Berger J (2003) Patterns of apparent extirpation among isolated populations of pikas (Ochotona princeps) in the Great Basin. J Mammal 84:37–54
Binder RG, Turner CE, Flath RA (1990) Comparison of yellow starthistle volatiles from different plant parts. J Agric Food Chem 38:764–767
Bohlmann J, Meyer-Gauen G, Croteau R (1998) Plant terpenoid synthases: molecular biology and phylogenetic analysis. Proc Natl Acad Sci USA 95:4126–4133
Bradley BA, Blumenthal DM, Wilcove DS, Ziska LH (2010) Predicting plant invasions in an era of global change. Trends Ecol Evol 25:310–318
Büchel K, Malskies S, Mayer M, Fenning TM, Gershenzon J, Hilker M, Meiners T (2011) How plants give early herbivore alert: volatile terpenoids attract parasitoids to egg-infested elms. Basic App Ecol 12:403–412
Bülow N, König WA (2000) The role of germacrene D as a precursor in sesquiterpene biosynthesis: investigations of acid catalyzed, photochemically and thermally induced rearrangements. Phytochemistry 55:141–168
Buttery RG, Maddox DM, Light DM, Ling LC (1986) Volatile components of yellow starthistle. J Agric Food Chem 34:786–788
Chappell J, VonLanken C, Vögeli U (1991) Elicitor-inducible 3-hydroxy-3-methylglutaryl coenzyme A reductase activity is required for sesquiterpene accumulation in tobacco cell suspension cultures. Plant Physiol 97:693–698
Chen F, Tholl D, D’Auria JC, Farooq A, Pichersky E, Gershenzon J (2003) Biosynthesis and emission of terpenoid volatiles from Arabidopsis flowers. Plant Cell 15:481–494
Co JE, Jones TH, Hefetz A, Tinaut A, Snelling RR (2003) The comparative exocrine chemistry of nine Old World species of Messor (Formicidae: Myrmicinae). Biochem Syst Ecol 31:367–373
Constable J, Litvak ME, Greenberg JP, Monson RK (1999) Monoterpene emission from coniferous trees in response to elevated CO2 concentration and climate warming. Glob Change Biol 5:252–267
Conti B, Canale A, Cioni PL, Flamini G, Rifici A (2011) Hyptis suaveolens and Hyptis spicigera (Lamiaceae) essential oils: qualitative analysis, contact toxicity and repellent activity against Sitophilus granarius (L.) (Coleoptera: Dryophthoridae). J Pest Sci 84:219–228
Crock J, Wildung M, Croteau R (1997) Isolation and bacterial expression of a sesquiterpene synthase cDNA clone from peppermint (Mentha piperita, L.) that produces the aphid alarm pheromone (E)-farnesene. Proc Natl Acad Sci 94:12833–12838
Descimon H, Bachelard P, Boitier E, Pierrat V (2005) Decline and extinction of Parnassius apollo populations in France. In: Kuhn E, Feldmann R, Settele J (eds) Studies on the ecology and conservation of butterflies in Europe (EBIE). Pensoft, Sofia, pp 114–115
Dicke M (2009) Behavioural and community ecology of plants that cry for help. Plant Cell Environ 32:654–665
Dicke M, Abelis MW, Takabayashi J, Bruin J, Posthumus MA (1990) Plant strategies of manipulating predator–prey interactions through allelochemicals: prospects for application in pest control. J Chem Ecol 16:3091–3117
DiTomaso J, Kyser GB, Pitcairn MJ (2006) Yellow starthistle management guide. In: Cal-IPC Publication 2006-03, California Invasive Plant Council, Berkeley, California. http://www.cal-ipc.org/ip/management/yst.php. Accessed 26 Nov 2011
Dudareva N, Negre F, Nagegowda DA, Orlova I (2006) Plant volatiles: recent advances and future perspectives. Crit Rev Plant Sci 25:417–440
Duhl TR, Helmig D, Guenther A (2008) Sesquiterpene emissions from vegetation: a review. Biogeosciences 5:761–777
Dukes JS (2000) Will the increasing atmospheric CO2 concentration affect the success of invasive species? In: Mooney HA, Hobbs RJ (eds) Invasive species in a changing world. Island Press, Washington, pp 95–113
Dukes JS, Mooney HA (1999) Does global change increase the success of biological invaders? Trends Ecol Evol 14:135–139
Dukes JS, Chiariello NR, Loarie SR, Field CB (2011) Strong response of an invasive plant species (Centaurea solstitialis L.) to global environmental changes. Ecol App 21:1887–1894
Edgington ES, Onghena P (2007) Randomization tests, 4th edn. Chapman & Hall, London
Field CB, Lobell DB, Peters HA, Chiariello NR (2007) Feedbacks of terrestrial ecosystems to climate change. Annu Rev Environ Resour 32:1–29
Fortunati A, Barta C, Brilli F, Centritto M, Zimmer I, Schnitzler JP, Loreto F (2008) Isoprene emission is not temperature-dependent during and after severe drought-stress: a physiological and biochemical analysis. Plant J 55:687–697
Francis F, Vandermoten S, Verheggen F, Lognay G, Haubruge E (2005) Is the (E)-β-farnesene only volatile terpenoid in aphids? J App Entomol 129:6–11
Gershenzon J (1994) Metabolic costs of terpenoid accumulation in higher plants. J Chem Ecol 20:1281–1328
Gershenzon J, Maffei M, Croteau R (1989) Biochemical and histochemical localization of monoterpene biosynthesis in the glandular trichomes of spearmint (Mentha spicata). Plant Physiol 89:1351–1357
Ghirardo A, Heller W, Fladung M, Schnitzler JP, Schroeder H (2012) Function of defensive volatiles in pedunculate oak (Quercus robur) is tricked by the moth Tortrix viridana. Plant Cell Environ 35:2192–2207
Gouinguené SP, Turlings TC (2002) The effects of abiotic factors on induced volatile emissions in corn plants. Plant Physiol 129:1296–1307
Grinspoon J, Bowman WD, Fall R (1991) Delayed onset of isoprene emission in developing velvet bean (Mucuna sp.) leaves. Plant Physiol 97:170–174
Groom SV, Ngo HT, Rehan SM, Skelton P, Stevens MI, Schwarz MP (2014) Multiple recent introductions of apid bees into Pacific archipelagos signify potentially large consequences for both agriculture and indigenous ecosystems. Biol Invas 16:1–10
Guenther AB, Zimmerman PR, Harley PC, Monson RK, Fall R (1993) Isoprene and monoterpene emission rate variability: model evaluations and sensitivity analyses. J Geophys Res Atmos 98:12609–12617
Heil M (2004) Direct defense or ecological costs: responses of herbivorous beetles to volatiles released by wild Lima bean (Phaseolus lunatus). J Chem Ecol 30:1289–1295
Hemmerlin A, Harwood JL, Bach TJ (2012) A raison d’être for two distinct pathways in the early steps of plant isoprenoid biosynthesis? Prog Lipid Res 51:95–148
Iijima Y, Davidovich-Rikanati R, Fridman E, Gang DR, Bar E, Lewinsohn E, Pichersky E (2004) The biochemical and molecular basis for the divergent patterns in the biosynthesis of terpenes and phenylpropenes in the peltate glands of three cultivars of basil. Plant Physiol 136:3724–3736
IPCC (2013a) Introduction. In: Stocker TF, Qin D, Plattner GK, Tignor M, Allen SK, Boschung J, Nauels A, Xia Y, Bex V, Midgley PM (eds) Climate change 2013: the physical science basis. Contribution of working group I to the fifth assessment report of the Intergovernmental Panel on Climate Change. Cambridge University Press, Cambridge, pp 119–158
IPCC (2013b) Summary for policymakers. In: Stocker TF, Qin D, Plattner GK, Tignor M, Allen SK, Boschung J, Nauels A, Xia Y, Bex V, Midgley PM (eds) Climate change 2013: the physical science basis. Contribution of working group I to the fifth assessment report of the Intergovernmental Panel on Climate Change. Cambridge University Press, Cambridge, pp 3–29
IPCC (2014a) Summary for policymakers. In: Field CB, Barros VR, Dokken DJ, Mach KJ, Mastrandrea MD, Bilir TE, Chatterjee M, Ebi KL, Estrada YO, Genova RC, Girma B, Kissel ES, Levy EN, MacCracken S, Mastrandrea PR, White LL (eds) Climate change 2014: impacts, adaptation, and vulnerability. Contribution of working group II to the fifth assessment report of the Intergovernmental Panel on Climate Change. Cambridge University Press, Cambridge, pp 3–20
IPCC (2014b) Terrestrial and inland water systems. In: Field CB, Barros VR, Dokken DJ, Mach KJ, Mastrandrea MD, Bilir TE, Chatterjee M, Ebi KL, Estrada YO, Genova RC, Girma B, Kissel ES, Levy EN, MacCracken S, Mastrandrea PR, White LL (eds) Climate change 2014: impacts, adaptation, and vulnerability. Contribution of working group II to the fifth assessment report of the Intergovernmental Panel on Climate Change. Cambridge University Press, Cambridge, pp 1–143
James JJ, Smith BS, Vasquez EA, Sheley RL (2010) Principles for ecologically based invasive plant management. Invasive Plant Sci Manag 3:229–239
Keville R, Kannowski PB (1975) Sexual excitation by pheromones of the confused flour beetle. J Insect Physiol 21:81–84
Kielty JP, Allen-Williams LJ, Underwood N, Eastwood EA (1996) Behavioral responses of three species of ground beetles (Coleoptera: Carabidae) to olfactory cues associated with prey and habitat. J Insect Behav 9:237–250
Koo HJ, Gang DR (2012) Suites of terpene synthases explain differential terpenoid production in ginger and turmeric tissues. PLoS One 7:e51481
Körner C (2006) Plant CO2 responses: an issue of definition, time and resource supply. New Phytol 172:393–411
Lerdau M, Litvak M, Monson R (1994) Plant chemical defense: monoterpenes and the growth-differentiation balance hypothesis. Trends Ecol Evol 9:58–61
Lichtenthaler HK (1999) The 1-deoxy-d-xylulose-5-phosphate pathway of isoprenoid biosynthesis in plants. Annu Rev Plant Biol 50:47–65
Light DM, Knight A (2005) Specificity of codling moth (Lepidoptera: Tortricidae) for the host plant kairomone, ethyl (2E,4Z)-2,4-decadienoate: field bioassays with pome fruit volatiles, analogue, and isomeric compounds. J Agric Food Chem 53:4046–4053
Llusià J, Peñuelas J, Alessio GA, Estiarte M (2008) Contrasting species-specific, compound-specific, seasonal, and interannual responses of foliar isoprenoid emissions to experimental drought in a Mediterranean Shrubland. Int J Plant Sci 169:637–645
Llusià J, Penuelas J, Prieto P, Estiarte M (2009) Net ecosystem exchange and whole plant isoprenoid emissions by a Mediterranean Shrubland exposed to experimental climate change. Russ J Plant Physl 56:29–37
Loreto F, Fischbach RJ, Schnitzler JP, Ciccioli P, Brancaleoni ENZO, Calfapietra C, Seufert G (2001) Monoterpene emission and monoterpene synthase activities in the Mediterranean evergreen oak Quercus ilex L. grown at elevated CO2 concentrations. Glob Change Biol 7:709–717
Manly BFJ (2007) Randomization, bootstrap, and Monte Carlo methods in biology, 3rd edn. Chapman & Hall, London
May B, Lange BM, Wüst M (2013) Biosynthesis of sesquiterpenes in grape berry exocarp of Vitis vinifera L.: evidence for a transport of farnesyl diphosphate precursors from plastids to the cytosol. Phytochemistry 95:135–144
McFrederick QS, Fuentes JD, Roulston TA, Kathilankal JC, Lerdau M (2009) Effects of air pollution on biogenic volatiles and ecological interactions. Oecologia 160:411–420
McGarvey DJ, Croteau R (1995) Terpenoid metabolism. Plant Cell 7:1015–1026
McMurry JE, Bosch GK (1985) Synthesis of bicyclogermacrene and lepidozene. Tetrahedron Lett 26:2167–2170
Monson RK, Trahan N, Rosenstiel TN et al (2007) Isoprene emission from terrestrial ecosystems in response to global change: minding the gap between models and observations. Philos Trans R Soc A 365:1677–1695
Mooney HA, Cleland EE (2001) The evolutionary impact of invasive species. Proc Natl Acad Sci 98:5446–5451
Mooney HA, Drake JA (1986) Ecology of biological invasions of North America and Hawaii. Springer, New York, pp 1–321
Norby RJ, DeLucia EH, Gielen B et al (2005) Forest response to elevated CO2 is conserved across a broad range of productivity. Proc Nati Acad Sci USA 102:18052–18056
Norby RJ, Warren JM, Iversen CM, Medlyn BE, McMurtrie RE (2010) CO2 enhancement of forest productivity constrained by limited nitrogen availability. Proc Natl Acad Sci USA 107:19368–19373
Ormeno (2007) Water deficit stress induces different monoterpene and sesquiterpene emission changes in Mediterranean species. Chemosphere 67:276–284
Oster M, Smith L, Beck JJ, Howard A, Field CB (2014) Orientation behavior of predaceous ground beetle species in response to volatile emissions identified from yellow starthistle damaged by an invasive slug. Arthropod Plant Interact 8:429–437
Peñuelas J, Staudt M (2010) BVOCs and global change. Trends Plant Sci 15:133–144
Peters HA, Cleland EE, Mooney HA, Field CB (2006) Herbivore control of annual grassland composition in current and future environments. Ecol Lett 9:86–94
Pitcairn M, Schoenig S, Yacoub R, Gendron J (2006) Yellow starthistle continues its spread in California. Calif Agri 60:83–90
Price PW, Bouton CE, Gross P, McPheron BA, Thompson JN, Weis AE (1980) Interactions among three trophic levels: influence of plants on interactions between insect herbivores and natural enemies. Annu Rev Ecol Syst 11:41–65
Ray DP, Srivastava S, Singh RP (2012) Gas chromatographic–mass spectrometric (GC–MS) analysis of marigold (Tagetes erecta L.) flower oil. Int J Agric Environ Biotechnol 5:215–218
Rosenstiel TN, Potosnak MJ, Griffin KL, Fall R, Monson RK (2003) Increased CO2 uncouples growth from isoprene emission in an agriforest ecosystem. Nature 421:256–259
Rustad L, Campbell J, Marion G et al (2001) A meta-analysis of the response of soil respiration, net nitrogen mineralization, and aboveground plant growth to experimental ecosystem warming. Oecologia 126:543–562
Senatore F, Rigano D, De Fusco R, Bruno M (2003) Volatile components of Centaurea cineraria L. subsp. umbrosa (Lacaita) Pign. and Centaurea napifolia L. (Asteraceae), two species growing wild in Sicily. Flavour Frag J 18:248–251
Sharkey TD, Chen XY, Yeh S (2001) Isoprene increases thermotolerance of fosmidomycin-fed leaves. Plant Physiol 125:2001–2006
Shaw MR, Zavaleta ES, Chiariello NR, Cleland EE, Mooney HA, Field CB (2002) Grassland responses to global environmental changes suppressed by elevated CO2. Science 298:1987–1990
Sidney M, Gries R, Danci A, Judd GJ, Gries G (2006) Almond volatiles attract neonate larvae of Anarsia lineatella (Zeller) (Lepidoptera: Gelechiidae). J Entomol Soc B C 103:3–10
Smith L, Beck JJ (2013) Effect of mechanical damage on emission of volatile organic compounds from plant leaves and implications for evaluation of host plant specificity of prospective biological control agents of weeds. Biocontrol Sci Technol 23:880–907
Steele CL, Crock J, Bohlmann J, Croteau R (1998) Sesquiterpene synthases from grand fir (Abies grandis): comparison of constitutive and wound-induced activities, and cDNA isolation, characterization, and bacterial expression of δ-selinene synthase and γ-humulene synthase. J Biol Chem 273:2078–2089
Tholl D (2006) Terpene synthases and the regulation, diversity and biological roles of terpene metabolism. Curr Opin Plant Biol 9:297–304
Tholl D, Chen F, Petri J, Gershenzon J, Pichersky E (2005) Two sesquiterpene synthases are responsible for the complex mixture of sesquiterpenes emitted from Arabidopsis flowers. Plant J 42:757–771
Tholl D, Boland W, Hansel A, Loreto F, Röse USR, Schnitzler JP (2006) Practical approaches to plant volatile analysis. Plant J 45:540–560
Thompson JN (2005) Coevolution: the geographic mosaic of coevolutionary arms races. Curr Biol 15:R992–R994
Turlings TCJ, Tumlinson JH, Lewis WJ (1990) Exploitation of herbivore-induced plant odors by host-seeking parasitic wasps. Science 250:1251–1253
Vallat A, Gu H, Dorn S (2005) How rainfall, relative humidity and temperature influence volatile emissions from apple trees in situ. Phytochemistry 66:1540–1550
Van Driesche R, Hoddle M, Center T (2008) The invasion crisis. Control of pests and weeds by natural enemies. Wiley-Blackwell, Malden, pp 69–79
van Groenigen KJ, Qi X, Osenberg CW, Luo Y, Hungate BA (2014) Faster decomposition under increased atmospheric CO2 limits soil carbon storage. Science 344:508–509
Verlinden M, De Boeck HJ, Nijs I (2014) Climate warming alters competition between two highly invasive alien plant species and dominant native competitors. Weed Res 54:234–244
Vet LEM, Dicke M (1992) Ecology of infochemical use by natural enemies in a tritrophic context. Annu Rev Entomol 37:141–172
Visser ME, Both C (2005) Shifts in phenology due to global climate change: the need for a yardstick. Proc R Soc B Biol Sci 272:2561–2569
Vuorinen T, Nerg AM, Ibrahim MA, Reddy GVP, Holopainen JK (2004) Emission of Plutella xylostella-induced compounds from cabbages grown at elevated CO2 and orientation behavior of the natural enemies. Plant Physiol 135:1984–1992
Wang Y, Kays SJ (2002) Sweetpotato volatile chemistry in relation to sweetpotato weevil (Cylas formicarius) behavior. J Am Soc Hortic Sci 127:656–662
Waterman PG, Mole S (1989) Extrinsic factors influencing production of secondary metabolites in plants. Insect Plant Interact 1:107–134
Weissbecker B, Van Loon JJA, Posthumus MA, Bouwmeester HR, Dicke M (2000) Identification of volatile potato sesquiterpenoids and their olfactory detection by the two-spotted stinkbug Perillus bioculatus. J Chem Ecol 26:1433–1445
Wheeler GS, Schaffner U (2013) Improved understanding of weed biological control safety and impact with chemical ecology: a review. Invasive Plant Sci Manag 6:16–29
Wilson LM, Jette C, Conett J, McAffrey J, Randall CB, Kuykendall C, Lake L (2003) Getting to know yellow starthistle. Biology and biological control of yellow starthistle. USDA Forest Service: Forest Health Technology Enterprise Team Publications, Idaho, pp 5–9
Woodward FI, Lake JA, Quick WP (2002) Stomatal development and CO2: ecological consequences. New Phytol 153:477–484
Yuan JS, Himanen SJ, Holopainen JK, Chen F, Stewart CN Jr (2009) Smelling global climate change: mitigation of function for plant volatile organic compounds. Trends Ecol Evol 24:323–331
Yuan R, Li S, Zheng X, Wu Q, Zhang H, Wang L (2014) Determination of volatiles in water lily flowers using gas chromatography–mass spectrometry. Anal Lett 47:1541–1551
Zavaleta ES, Shaw MR, Chiariello NR, Thomas BD, Cleland EE, Field CB, Mooney HA (2003) Grassland responses to three years of elevated temperature, CO2, precipitation, and N deposition. Ecol Monogr 73:585–604
Acknowledgments
We would like to express gratitude to Nona Chiariello of Jasper Ridge Biological Preserve at Stanford University, and Michael Dini and Todd Tobeck of the Carnegie Institution for Science for providing field advice and support. We also thank Wai Gee of the USDA Agricultural Research Service for providing technical knowledge. This Jasper Ridge Global Change experiment was supported by the National Science Foundation (Grant 091817), A. W. Mellon Foundation, Carnegie Institution for Science, and Stanford University.
Author information
Authors and Affiliations
Corresponding author
Ethics declarations
Conflict of interest
The authors have no conflict of interest to declare.
Additional information
Handling Editor: Liliane Ruess.
Rights and permissions
About this article
Cite this article
Oster, M., Beck, J.J., Furrow, R.E. et al. In-field yellow starthistle (Centaurea solstitialis) volatile composition under elevated temperature and CO2 and implications for future control. Chemoecology 25, 313–323 (2015). https://doi.org/10.1007/s00049-015-0200-y
Received:
Accepted:
Published:
Issue Date:
DOI: https://doi.org/10.1007/s00049-015-0200-y