Abstract
This study was performed to examine the effect of emulsifiers used to coat emulsion droplets containing β-carotene on the behavior of lipid digestion and bioaccessibility. Different emulsifiers (whey protein isolate, soy protein isolate, sodium caseinate, Tween 20, and soy lecithin) were used to prepare emulsions with similar sized droplets (200–400 nm). Protein-stabilized emulsions showed a similar behavior of digestion, and morphological change in the simulated gastrointestinal conditions. Soy lecithin-stabilized emulsions showed the lowest rate and extent of lipid digestion probably due to the low emulsifying capability of soy lecithin, showing coalesced droplets occurring after exposure to the gastric phase. Tween 20-stabilized emulsions had a lower rate and extent of lipid digestion than that of protein-stabilized emulsions, even though Tween 20-stabilized emulsions had a more stable structure to resistant to aggregation in gastric phase. Even though the difference in the digestion rate and extent, β-carotene bioaccessibility was not significantly different among emulsions stabilized by different emulsifiers at p < 0.05.
Similar content being viewed by others
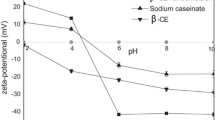
Explore related subjects
Discover the latest articles, news and stories from top researchers in related subjects.Avoid common mistakes on your manuscript.
Introduction
The incorporation of nutraceuticals into the food system is becoming an increasingly important strategy in promoting health. However, many nutraceuticals have poor solubility in water, chemical instability, and limited bioavailability; hence, they cannot simply be incorporated into food systems. It is useless to intake nutraceuticals if they cannot show efficacy in the human body [1,2,3,4]. As a way to overcome these constraints, several encapsulation technologies have been reported, such as liposomes, solid lipid nanoparticles, emulsions, nanoemulsions, and biopolymer-based particles [5,6,7]. Oil-in-water emulsions have been often used as a carrier vehicle for lipophilic nutraceuticals, since lipophilic materials can be solubilized into the oil droplets and can be isolated from the outer aqueous phase. This results in improved water-dispersing capacity, chemical stability, and oral bioavailability of nutraceuticals.
Recent researches have reported that an understanding of emulsion properties such as size of droplet, interfacial charge, viscosity, and the response of emulsions to various environmental conditions (e.g., pH, mechanical force, ionic strength, enzyme) is necessary for controlling the release, digestion, and bioaccessibility of nutraceuticals incorporated within an emulsion [8,9,10]. In summary, the structure and composition of emulsions can be designed to improve the bioavailability of incorporated materials, protect them from degradation, and control the release of incorporated materials.
Food emulsions can be stabilized by different types of emulsifiers, which can affect emulsion properties, and subsequently, the behaviors of emulsions during the lipid digestion process. For example, a different emulsifier may increase or decrease the surface area of lipid exposed to lipase by altering the aggregation stability of emulsion, or may alter the tendency for lipase/co-lipase adsorption. Consequently, understanding the role of emulsifier type in the lipid digestion process is important. The effect of various emulsifiers on the overall behavior of food emulsions in the gastrointestinal tract (GIT) has previously been studied [10,11,12,13]. However, the bioaccessibility of nutraceuticals among emulsions stabilized by different emulsifiers is not well characterized.
Therefore, the main purpose of the present study was to elucidate the effects of emulsifiers on the digestion of oil-in-water emulsions and the bioaccessibility of β-carotene contained in oil droplets of emulsions. We used four different natural emulsifiers {sodium caseinate (SC), whey protein isolate (WPI), soy protein isolate (SPI), and soy lecithin (SL)} and one synthetic emulsifier (Tween 20), which are in general use in the food industry. A highly lipophilic β-carotene was used as a model nutraceutical to be incorporated.
Material and Methods
Materials
WPI (product code: 9500) was provided by Protient, Inc. (St. Paul, MN, USA). SPI and SC were obtained from Suihua Jinlong Oil Co., Ltd. (Suihua, China) and Nanyung Commercial Co., Ltd., respectively. SL was obtained from Cargill Incorporated (Wayzata, MN). Tween 20, mucin (from porcine stomach), pancreatin (from porcine pancreas), pepsin (from porcine gastric mucosa), β-carotene, and bile extract (porcine) were purchased from Sigma Aldrich (St. Louis, MO). Soybean oil (Ottogi Corp., Pyeongtaek, Korea) was obtained from a local store.
Emulsion Preparation
β-Carotene (0.3 wt%) was dissolved in soybean oil. For complete dissolution, soybean oil containing β-carotene was sonicated (Ultrasonic Cleaner-Powersonic 410, Hwashin, Seoul, Korea) for 15 min and heated at 60 °C for 30 min with agitation. SC, WPI, SPI, SL, and Tween 20 solutions were prepare by dispersing the corresponding emulsifiers in phosphate buffer (10 mM, pH 7.0), and then stirred for 3 h. A stock emulsion (4 wt% oil) was fabricated by homogenizing emulsifier solution and soybean oil by using a high speed blender (ULTRA-TURRAX model T25 digital, IKA, Germany) for 2 min and then passing through a microfludizer (Picomax MN 250A, Micronox, Seongnam, Korea) four times at 6.89 MPa. Since mean size of the emulsion droplet could affect the lipid digestion and bioaccessibility of incorporated nutraceuticals [8, 14, 15], emulsions containing similar-sized lipid droplets were prepared for different emulsifiers. From preliminary experiments, the optimum ratio of oil to emulsifier of prepared emulsions showing desirable particle diameter and stability was determined to be 6:1 for WPI-, SC-, and Tween 20-stabilized emulsions, 3:1 for SPI emulsion, and 4:1 for SL emulsion.
Simulated Gastrointestinal Tract Model
The gastrointestinal fate of each emulsion was studied and compared to examine the effect of different emulsifiers on emulsion digestion. Samples were hydrolyzed using an in vitro GIT model as described in previous studies [14,15,16,17]. Mouth phase: Simulated saliva fluid (SSF) containing various salts and mucin [18] was preheated to 37 °C and mixed with emulsion samples at a 1:1 v/v ratio. SSF was prepared according to the composition used in the previous study [18]. More specifically, SSF consisted of sodium chloride (NaCl, 1.594 g/L), ammonium nitrate (NH4NO3, 0.328 g/L), potassium phosphate (KH2PO4, 0.636 g/L), potassium chloride (KCl, 0.202 g/L), potassium citrate (K3C6H5O7·H2O, 0.308 g/L), uric acid sodium salt (C5H3N4O3Na, 0.021 g/L), urea (H2NCONH2, 0.198 g/L), lactic acid sodium salt (C3H5O3Na, 0.146 g/L), and mucin (30 g/L). After adjusting the pH of the mixture to 6.8, it was reacted at 37 °C for 10 min with gentle agitation. Stomach phase: The sample from the mouth phase was added to simulated gastric fluid (SGF). After the pH was adjusted to 2.5, the mixtures was held at 37 °C for 2 h with gentle agitation. SGF contained 2 g of sodium chloride (NaCl) and 3.2 g of pepsin in 1 L of water, and pH was adjusted to 2.5 by using HCl. Intestinal phase: 30-mL samples from the gastric phase were transferred to 100-mL glass beakers and were placed in a shaking water bath (37 °C). The pH of the sample was set at 7.0. To mimic the small intestine phase, the salt solution (1.5 mL; 10 mM CaCl2 and 150 mM NaCl) and the bile extract solution (3.5 mL; 187.5 mg/3.5 ml) were added to the reaction vessel. Then, pancreatin suspension (187.5 mg/2.5 mL phosphate buffer) was added to the mixture and it was digested at 37 °C for 2 h.
Samples were taken at regular intervals of time (in minutes) during lipid digestion, and the volume of 0.25 M NaOH solution required to neutralize any free fatty acids (FFAs) released due to lipid digestion was measured using a pH meter (Orion 420A+, Thermo Electron Corporation, Massachusetts, USA). The percentage of FFAs released was calculated from the volume of sodium hydroxide solution (0.25 M) required to neutralize FFA, using the following eq. [16, 17].
where VNaOH is the volume of the titrant (NaOH) in liters, mNaOH is the molarity of NaOH, MLipid is the molecular weight of soybean oil, and WLipid is the weight of oil in the digestion system in grams. Blanks (samples without oil) were also run, and the volume of titrant used for these blank samples was subtracted from the corresponding test samples that contained oil.
Characterization of Emulsion Samples
The particle diameter and ζ-potential of the droplets was analyzed by the dynamic light scattering method using Zetasizer (ZS90, Malvern Instruments Ltd., Worcestershire, UK). Prior to measurement, emulsions (100 μL) were diluted with 10 mM phosphate buffer (4900 μL) to avoid multiple scattering. Mean particle diameter (Z-average) was obtained from the graph of signal intensity. Structural changes within different phases of the gastrointestinal tract (GIT) model were monitored under a microscope (Carl Zeiss, Axio Imager A1, Göttingen, Germany) at a magnification of 40 × .
Determination of β-Carotene Bioaccessibility
The bioaccessibility of β-carotene was determined after in vitro digestion processing of the samples [14, 19]. Raw digesta were collected and centrifuged at 6583 g for 20 min at 25 °C (Supra 22 K, Hanil Science Inc., Korea). The supernatant was collected and taken as a micelle fraction, in which the bioactive component was solubilized. Aliquots of 5 mL of the raw digesta or supernatant were mixed with 5 mL of chloroform, stirred, and then centrifuged at 1158 g for 10 min at 25 °C. Finally, solubilized β-carotene was collected from the bottom layer, and the process was repeated using the top layer to obtain more β-carotene. The bottom chloroform layer obtained after centrifugation was combined with the previous one and analyzed spectrophotometrically (UV-1650 PC, Shimadzu, Kyoto, Japan) at 450 nm. Chloroform was used as the reference.
The concentration of β-carotene extracted from a sample was determined from a calibration curve of absorbance versus the β-carotene concentration in chloroform. Bioaccessibility was then calculated according to the following equation:
where CMicelle and CRawDigesta are the concentrations of β-carotene in the micelle fraction and in the raw digesta, respectively [15, 19].
Statistical Analysis
All data presented were the mean ± standard deviation. Statistical analysis was performed using SPSS for windows (ver. 21.0, IBM Corp., Armonk, N.Y., USA). A one-way ANOVA test followed by a Duncan’s multiple range test was conducted to identify statistical significances (P < 0.05).
Results and Discussion
Influence of Emulsifier Type on Particle Size of Emulsions in Simulated GIT
It is well known that droplet size is one of the important factors in determining lipid digestibility and bioaccessibility of nutraceuticals entrapped within lipid droplets [8, 14, 15, 20]. The initial mean particle diameters of emulsions stabilized with SC, WPI, SPI, Tween 20, and SL were 207.9, 182.7, 233, 171.9, and 454.7 nm, respectively and the ratios of oil to emulsifier to obtain the corresponding mean particle diameters were 6:1 for SC, WPI, and Tween 20, 3:1 for SPI, and 4:1 for SL as determined from preliminary experiments. Unlike other emulsifiers, SL did not produce ~200-nm sized lipid droplets at experimental conditions used in this study.
Lecithins are surface-active and are prepared by extracting and purifying phospholipids from naturally occurring products such as soybeans, rapeseed, and eggs [21]. It has been reported that natural lecithin has intermediate solubility characteristics and hydrophile-lipophile balance numbers (~8), indicating that it is not particularly suitable as stabilizer for either O/W or W/O emulsions when used in isolation [21]. With SL, about 400-nm-sized lipid droplets could be manufactured at the ratio 4:1 (oil:lecithin) and these were the best for SL. SPI required relatively high oil to emulsifier ratio of 3:1 to produce emulsion with ~200 nm-sized droplets, compared to other emulsifiers used in this study. It has been reported that SPI has a relatively poor emulsifying capability compared to other proteins. It is well known that the production of commercial SPI involves alkali extraction, acid precipitation and spray drying and extraction processing itself leads to denaturation of the proteins. Therefore, most of SPI is present in the denatured and/or aggregated form, consequently, the emulsifying properties of SPI are relatively poor [22, 23].
Upon exposure to simulated oral conditions, all of the β-carotene-loaded emulsions prepared in this study showed an increase in their mean particle diameters, although not significantly different (Fig. 1). Simulated saliva solution contains mucin, a charged glycoprotein known to promote droplet flocculation through both depletion and bridging mechanisms [15, 18]. Previous researches have reported that bridging flocculation may have occurred between charged groups of the lipid droplet surfaces and oppositely charged groups of mucin polymers and also that depletion flocculation by mucin molecules located in the aqueous phase has occurred [15, 18, 24].
Influence of simulated gastrointestinal conditions on the mean droplet diameter (Z-average) of soybean oil-in-water emulsions stabilized by different emulsifiers. Different capital letters mean significant differences (p < 0.05) of the particle diameter of a sample between emulsifier types within the same digestion phase. Different lowercase letters mean significant differences (p < 0.05) of the particle diameter of a sample between digestion phases. WPI = whey protein isolate; SC = sodium caseinate; SPI = soy protein isolate; SL = soy lecithin
Emulsions stabilized by different emulsifiers showed different behavior after exposure to stomach conditions. There was an appreciable increase in the mean particle diameter of SC-, WPI-, SPI-, and SL-stabilized emulsions, however, the mean particle diameter of Tween 20 emulsions was similar to that of the oral phase. Microstructures observed using optical microcopy also showed highly aggregated droplets shown as large clumps for protein (SC, WPI, and SPI)-stabilized emulsions at stomach stage (Fig. 2). Previously, it has been reported that protein-stabilized emulsions are unstable under gastric phase [16, 25, 26]. This may be attributed to a number of reasons: reduction in pH, increase in ionic strength in the simulated stomach phase and hydrolysis of protein by pepsin included in the simulated gastric fluids [27]. After exposing to the stomach condition, SPI-stabilized emulsion showed the largest change in particle diameter among other protein-stabilized emulsions. This result might be related to the interfacial structure of SPI different from others. Previous researches suggested that proteins with high tendency to aggregate like SPI could form very thick but inhomogeneous interfacial films at the surface of droplets, and void spaces might exist in the interfacial films of aggregated proteins, which is vulnerable to rupture. Thus, it seems that the structural properties of SPI at the interface of oil droplet might be related to the largest change of particle diameter [28, 29].
The SL-stabilized emulsions were more apt to coalescence when emulsion was treated at simulated gastric conditions. While many clustered droplets were observed in the protein-stabilized emulsions, large individual droplets were observed in SL-stabilized emulsions after exposure to stomach condition (Fig. 2).
The high level of aggregation was not observed in the Tween 20-stabilized emulsion after exposure to simulated gastric phase, which was consistent with the particle size distribution data (Fig. 3). This result was in good agreement with previous studies reporting that the size of droplets coated by certain types of non-ionic surfactant was not significantly changed under gastric conditions [14, 15, 30, 31].
Our results suggested that the type of emulsifier used to stabilize the lipid droplets could have an influence on the response to oral and gastric conditions. For example, when emulsions were exposed to the simulated gastric phase, emulsions stabilized by proteins had droplet aggregations, whereas emulsion stabilized by smaller molecular weight surfactant (Tween 20) presented higher stability against flocculation and coalescence than protein and SL-stabilized emulsions. Owing to the complexity of digesta in the small intestine phase, it is difficult to describe the origin of particles, because the digesta would consist of various colloidal structures, such as undigested lipid droplets, micelles, vesicle, calcium soap, and other structures [10, 14,15,16].
Influence of Emulsifier Type on ζ -Potential of Emulsions in Simulated GIT
The ζ-potential of the emulsion samples was analyzed after exposure to gastrointestinal conditions (Fig. 4). The ζ-potential of the intact emulsions stabilized by SC, WPI, SPI, SL, and Tween 20 (pH 7.0) was −39.3, −45.4, −34.0, −56.8, and −15.7 mV, respectively. Protein-coated lipid droplets had a negative charge because the isoelectric point (pI) of casein, whey protein, and soy protein (pI ~5) is much lower than pH 7. The negative charge of the emulsions stabilized by SL could be caused by the presence of anionic phospholipids from the lecithin. The emulsions stabilized by non-ionic surfactant Tween 20 also showed negative value of ζ-potential, which might be due to anionic impurities present in the surfactant or oil, or due to adsorption of OH− from water to the droplet surfaces [32].
Influence of simulated gastrointestinal conditions on the particle charge (ζ-potential) of soybean oil-in-water emulsions stabilized by different emulsifiers. Different capital letters mean significant differences (p < 0.05) of the particle diameter of a sample between emulsifier types within the same digestion phase. Different lowercase letters mean significant differences (p < 0.05) of the particle diameter of a sample between digestion phases. WPI = whey protein isolate; SC = sodium caseinate; SPI = soy protein isolate; SL = soy lecithin
Among protein emulsifiers, the initial ζ-potential of the lipid droplets coated by WPI was the most negative. This finding could be due to a difference in the conformations and amino acid profiles of the different proteins [13]. The ζ-potential of the lipid droplets in the protein-stabilized emulsion became less negative after reaction with SSF and SGF in the simulated oral and gastric phase. The change in ζ-potential in the oral phase might be attributed to the interaction of the mucin contained in SSF with the droplet surface and also to various ions present in the simulated oral phase [33]. We expected that the emulsions might be positively charged in the gastric phase because the pH was below the pI of protein. However, the emulsions were negatively charged. This result could be attributed to electrostatic screening effects caused by pH changes, hydrolysis of protein, and displacement of protein by other molecules (e.g., pepsin and peptides produced by proteolysis). Following the 2-h digestion in the small intestine phase, the ζ-potential value of lipid droplets coated with different emulsifiers was around −30 mV, with little difference among different emulsions. According to previous studies, these negative charges might be caused by the presence of various anionic particulate matters in the digesta (e.g., vesicles, micelles, and undigested protein aggregates) which consist of negatively charged molecules (e.g., released free fatty acid or peptides) [13,14,15,16]. The microstructure of the emulsion samples in the intestinal phase also showed small particles distributed evenly, even though it was difficult to identify those particles by optical microscopy (Fig. 2).
Influence on Lipid Digestion
Figure 5 shows the percentage of FFAs released from β-carotene-loaded emulsions fabricated with different emulsifiers. The amount of FFA released from each emulsifier solution without soybean oil was also measured and subtracted from those of the emulsions.
Influence of emulsifiers on the calculated percentage of free fatty acids (FFA%) released during digestion in the simulated intestinal phase. Tween20 and SL-stabilized emulsions showed significant difference (P < 0.05) between them as well as with the other protein-stabilized samples in the amount of FFA after 2 h of digestion
The rates and extent of digestion of emulsions stabilized by proteins (SC, WPI, and SPI) were similar, whereas the Tween 20- and SL- stabilized emulsions had lower rates and extent of digestion. The digestion rate and extent of SL-stabilized emulsions measured after 2-h reaction in the simulated small intestinal phase was the lowest. These data suggest that the type of emulsifier can affect the rate and extent of lipid digestion in the emulsions. Several studies have reported that emulsifiers interacted with components present in the gastrointestinal tract, affecting the droplet sizes and subsequently had an impact on lipid digestion [11, 34].
As shown in Fig. 1 and 2, there were differences in the behaviors observed in the oral and gastric phases among proteins, Tween 20, and SL-stabilized emulsions. Tween 20-stabilized emulsions had a more stable structure resistant to aggregation in gastric phase but the protein-stabilized emulsion had extensive aggregations and the SL-stabilized emulsion had some coalesced droplets. Therefore, as previous researchers have reported, we expected that the Tween 20-stabilized emulsion would be digested more readily, because the surface area of lipids accessible to lipase is larger than those of other emulsifier-stabilized emulsions. However, the result was different from what we expect. The Tween 20-stabilized emulsion showed a lower rate and extent of lipid digestion compared to protein-stabilized emulsions. This result might be due to the resistance of the interface formed by Tween 20 to displacement by phospholipids and bile salts, which restricted the subsequent adsorption of the lipase/co-lipase complex necessary for lipid digestion [35].
In a previous study, similar result have been shown. The amount of fatty acid released after 2-h digestion with pancreatic lipase was higher in the presence of bile extract for protein-coated droplets than for Tween 20- or lecithin-coated droplets [32]. The small-molecule surfactant like Tween 20 is much more surface-active than other emulsifiers. Consequently, Tween 20 might affect the ability of bile extract, lipase, and other reactants found in the simulated digestion fluids to adsorb to the droplet surfaces.
Influence on β-Carotene Bioaccessibility
The effect of emulsifiers on the bioaccessibility of β–carotene incorporated in the oil phase of emulsions was investigated (Fig. 6). The bioaccessibility is the fraction of compound which is released from the food matrix in the GIT tract and then becomes available for absorption and in general, mixed micelle is considered as a form available for absorption [3, 14]. In this study, the bioaccessibility was obtained by measuring the amount of β-carotene in mixed micelles formed after lipid digestion and centrifugation.
The digestion of the emulsified lipid in the presence of phospholipids and bile acids could form the mixed micelles necessary for the transfer of β-carotene to the intestinal epithelia for uptake into the body. Many studies have reported that emulsifier-stabilized emulsions containing long chain triglycerides could improve the bioaccessibility of β–carotene and the solubilization capacity of the mixed micelle increased with fat content [14, 36]. This effect was attributed to the lipid digestion products (i.e., monoglycerides and free fatty acid) released during digestion. They could form mixed micelles in the small intestinal fluids for solubilization and transportation of the β–carotene molecules.
In the current study, the bioaccessibility was not significantly different (p > 0.05) among samples, despite the difference in the rate and extent of lipid digestion among emulsions. We expected that SL-stabilized emulsions would have lower bioaccessibility than other emulsions, since more undigested oil was remained in the SL-stabilized emulsion as shown in the result of lipid digestion (Fig. 5). It has been reported that improved lipolysis by lipase could generate more hydrolysis products (MAGs and FFAs), which could improve micelle-formation and, in turn, promote β-carotene incorporation within mixed micelles [37]. Therefore, an increase in undigested oil might work negatively to form β-carotene-contained mixed micelles. Moreover, some of the β-carotene might be trapped in the undigested oil. However, the bioaccessibility of SL-stabilized emulsion was not significantly different from those of protein-stabilized emulsions that showed a relatively higher digestion rate and extent.
SPI has been reported to form a complex with liphophilic bioactives such as curcumin [38]. Therefore, it was expected that the ability of SPI to form the complex with liphophilic compounds would affect the β-carotene bioaccessibility. However, there was no a significant impact.
There might be more complicated mechanism behind the bioaccessibility of β-carotene in emulsion system, which would require more intensive further study.
Conclusions
The characteristics of emulsions shown while they were digested (by in vitro digestion assay using the simulated GIT) and the lipolysis pattern of emulsions depended on emulsifier type. Electrical charge and aggregation stability of lipid droplets as emulsion passed through the simulated gastrointestinal tract varied depending on the type of emulsifier. The initial rate and extent of lipid digestion was appreciably the lowest for the SL-coated lipid droplets and then Tween 20-coated lipid droplets showed a lower rate and extent of lipid digestion than protein stabilized emulsions. Even though the Tween 20-stabilized emulsion was the only emulsion stable to aggregation in the gastric phase, it showed a reduced rate and extent of lipid digestion compared to those of protein-stabilized emulsion. The bioaccessibility of β-carotene in WPI-, SC-, and SPI-stabilized emulsions that showed similar rate and extent of lipid digestion was around 31%, 29%, and 41%, respectively. The bioaccessibility of β-carotene in Tween 20-, and SL-stabilized emulsions that showed the lower rate and extent of lipid digestion was around 27 and 23%, respectively. In spite of differences in digestion rate and extent, β-carotene bioaccessibility was not significantly different among emulsions stabilized by different emulsifiers at p < 0.05.
References
J.L. Tang, J. Sun, Z.G. He, Curr. Drug Ther. 2, 85 (2007)
R. Liang, C.F. Shoemaker, X. Yang, F. Zhong, Q. Huang, J. Agr. Food Chem. 61, 1249 (2013)
D.J. McClements, Expert Opin. Drug Deliv. 10, 1621 (2013)
E. Reboul, Nutrients 5, 3563 (2013)
I.J. Joye, D.J. McClements, Curr. Opin. Colloid In. 19, 417 (2014)
A. Maltais, G.E. Remondetto, M. Subirade, Food Hydrocoll. 23, 1647 (2009)
D.J. McClements, Y. Li, Adv. Colloid Interfac. Sci. 159, 213 (2010)
Z. Hou, Y. Liu, F. Lei, Y. Gao, LWT-Food Sci. Technol. 59, 867 (2014)
L. Mao, S. Miao, Food Eng. Rev. 7, 439 (2015)
R. Zhang, Z. Zhang, H. Zhang, E.A. Decker, D.J. McClements, Food Hydrocoll. 45, 175 (2015)
S.J. Hur, E.A. Decker, D.J. McClements, Food Chem. 114, 253 (2009)
J. Yi, Y. Li, F. Zhong, W. Yokoyama, Food Hydrocoll. 35, 19 (2014)
C. Qiu, M. Zhao, E.A. Decker, D.J. McClements, Food Chem. 175, 249 (2015)
T.L. Salvia, C. Qian, B.O. Martín, D.J. McClements, Food Chem. 139, 878 (2013)
T.L. Salvia, C. Qian, B.O. Martín, D.J. McClements, Food Chem. 141, 1472 (2013)
S.H. Mun, Y.R. Kim, D.J. McClements, Food Chem. 173, 454 (2015)
C.L. Lopez-Pena, B. Zheng, D.A. Sela, E.A. Decker, H. Xiao, D.J. McClements, Food Chem. 192, 857 (2016)
A. Sarkar, K.K.T. Goh, H. Singh, Food Hydrocoll. 23, 1270 (2009)
C. Qian, E.A. Decker, H. Xiao, D.J. McClements, Food Chem. 135, 1440 (2012)
E. Troncoso, J.M. Aguilera, D.J. McClements, J. Colloid Interf. Sci. 382, 110 (2012)
D.J. McClements, Food emulsions: Principles, practices and techniques (CRC Press, Boca Raton, 2005)
L. Chen, J. Chen, J. Ren, M. Zhao, Food Hydrocoll. 25, 887 (2011)
C.H. Tang, X.R. Li, Food Res. Int. 52, 419 (2013)
E. Silletti, M.H. Vingerhoeds, W. Norde, G.A. van Aken, Food Hydrocoll. 21, 596 (2007)
J. Li, A. Ye, S.J. Lee, H. Singh, Colloid Surf. B. 111, 80 (2013)
C. Yucel, V. Quagliariello, R.V. Iaffaioli, G. Ferrari, F. Donsì, Int. J. Pharm. 494, 357 (2015)
H. Singh, A.Q. Ye, D. Horne, Prog. Lipid Res. 48, 92 (2009)
C.H. Tang, Crit. Rev. Food Sci. Nutr. 57, 2636 (2017)
S. Damodaran, J. Food Sci. 70, R54 (2005)
G.A. Van Aken, E. Bomhof, F.D. Zoet, M. Verbeek, A. Oosterveld, Food Hydrocoll. 25, 781 (2011)
S.H. Mun, Y.R. Kim, M.S. Shin, D.J. McClements, Food Hydrocoll. 44, 380 (2015)
S.H. Mun, E.A. Decker, D.J. McClements, Food Res. Int. 40, 770 (2007)
H. Singh, A. Sarkar, Adv. Colloid Interfac. Sci. 165, 47 (2011)
Y. Chang, D.J. McClements, Food Hydrocoll. 61, 92 (2016)
G.A. Torcello, V.J. Maldonado, R.A. Martín, D.J. McClements, Soft Matter 7, 6167 (2011)
J.Ø. Christensen, K. Schultz, B. Mollgaard, H.G. Kristensen, A. Mullertz, Eur. J. Pharm. Sci. 23, 287 (2004)
T.A.J. Verrijssen, K.H.G. Smeets, S. Christiaens, S. Palmers, A.M. Van Loey, M.E. Hendrickx, Food Res. Int. 67, 60 (2015)
A. Tapal, P.K. Tiku, Food Chem. 130, 960 (2013)
Acknowledgements
This research was partly supported by Basic Science Research Program through the National Research Foundation of Korea (NRF) grants funded by the Ministry of Science, ICT, and Future Planning (NRF-2015R1A1A3A04001485). This work was also partly supported by Korea Institute of Planning and Evaluation for Technology in Food, Agriculture, Forestry and Fisheries (IPET) through High value-added Food Technology Development Program, funded by Ministry of Agriculture, Food and Rural Affairs (MAFRA), Republic of Korea (Project No. 315065-3).
Author information
Authors and Affiliations
Corresponding authors
Rights and permissions
About this article
Cite this article
Park, S., Mun, S. & Kim, YR. Emulsifier Dependent in vitro Digestion and Bioaccessibility of β-Carotene Loaded in Oil-in-Water Emulsions. Food Biophysics 13, 147–154 (2018). https://doi.org/10.1007/s11483-018-9520-0
Received:
Accepted:
Published:
Issue Date:
DOI: https://doi.org/10.1007/s11483-018-9520-0