Abstract
Purpose
The choice and timing of microorganisms added to soils for bioremediation is affected by the dominant bioavailable contaminants in the soil. However, changes to the concentration of bioavailable PAHs in soil are not clear, especially when several PAHs coexist. This study investigated the effects of PAH concentration and chemical properties on desorption in meadow brown soil after a 1-year aging period, which could reflect changes of PAH bioavailability during bioremediation.
Materials and methods
Based on the percentage of different molecular weights in a field investigation, high-level contaminated soil (HCS) and low-level contaminated soil (LCS) were prepared by adding phenanthrene (PHE), pyrene (PYR) and benzo(a)pyrene (BaP) to uncontaminated meadow brown soil. The concentrations of HCS and LCS were 250 mg kg−1 (PHE, PYR, and BaP: 100, 100, and 50 mg kg−1) and 50 mg kg−1 (PHE, PYR, and BaP: 20, 20, and 10 mg kg−1) respectively. The soils were aged for 1 year, after which desorption was induced by means of a XAD-2 adsorption technique over a 96-h period.
Results and discussion
The range of the rapidly desorbing fraction (F rap) for PHE, PYR, and BaP in HCS and LCS was from 1.9 to 27.8 %. In HCS, desorption of PYR was most difficult, and the rate constant of very slow desorption (K vs) of PYR was 8 orders of magnitude lower than that of BaP, which had similar very slow desorbing fractions (49.8 and 50.5 %, respectively). However, in LCS, desorption of PYR was the easiest; the Kvs of PYR was 8–10 orders of magnitude higher than those of PHE and BaP. In HCS, the time scale for release of 50 % of the PAHs was ranked as BaP > PYR > PHE, while in LCS this was BaP > PHE > PYR.
Conclusions
The combined effect of PAH concentrations and properties should be taken into account during desorption. The desorption of PAH did not always decrease with increasing molecular weight, and the desorption of four-ring PAHs might be special. These results are useful for screening biodegrading microbes and determining when they should be added to soils based on the dominant contaminants present during different periods, thus improving the efficiency of soil bioremediation.
Similar content being viewed by others
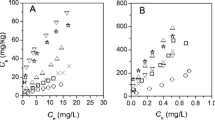
Explore related subjects
Discover the latest articles, news and stories from top researchers in related subjects.Avoid common mistakes on your manuscript.
1 Introduction
Bioremediation, realized by bioaugmentation and biostimulation, is one of the most promising methods for removing polycyclic aromatic hydrocarbons (PAHs) from contaminated soils (Colombo et al. 2011). Changes occur to the microorganisms used to effectively degrade special PAHs and the structure of the microbe community during bioremediation (Lors et al. 2010). Hence, selecting and adding different microbe communities to contaminated soil at different biodegradation stages of PAHs could significantly improve bioremediation (Singleton et al. 2011). Bioavailability, rather than total concentration, of PAHs are critical for defining the exposure, uptake and risk of PAHs to organisms in the environment, and for assessing the feasibility and effectiveness of bioremediation. Desorption indicates the bioavailability of PAHs and changes to PAH components during bioremediation. The bioavailable fraction of soil-associated PAHs are expressed as the rapidly desorbing fraction, and biphasic or triphasic empirical models have been successfully used to describe the rapid release of the labile fraction followed by a slow and more limited release of the resistant fraction (Chai et al. 2006; Rhodes et al. 2010).
Desorption is influenced by many factors such as the components and properties of contaminants, soil properties, aging time, contaminant concentrations, and sorbents, etc. However, the effect of PAH concentration or property on desorption was not always consistent in different studies. You et al. (2007) observed that most of the rapidly desorbing fractions of hydrophobic organic contaminants (HOC) were similar for all HOCs regardless of concentration. However, another study showed that phenanthrene generally desorbed least (3–50 %) for all sediments and size fractions compared to pyrene (40–80 %) and benzo(a)pyrene (20–80 %) in field samples (Oen et al. 2006). These make it difficult to select highly effective microorganisms for biodegradation in the field.
Hence, the aims in present study were as follows: (1) to identify the effect of PAH concentration on desorption; (2) to determine desorption of different PAHs in laboratory-spiked soil that has been aged for 1 year in the same meadow brown soil.
2 Materials and methods
2.1 Compounds
Phenanthrene (PHE), pyrene (PYR), and benzo(a)pyrene (BaP) used in this study were obtained from Fluka Corporation (Germany), and other chemicals were purchased from Concord Corporation of Tianjin, China. A pre-cleaned high purity Amberlite XAD-2 macroreticular styrenedivinylbenzene copolymer resin (XAD-2) was purchased from Sigma-Aldrich Corporation (USA). The physical and chemical properties of PHE, PYR, and BaP are listed in Table 1.
2.2 Soil collection
The meadow brown soil sample was collected from the surface layer (0–20 cm) of cultivated soil at the Shenyang Ecological Experimental Station, Chinese Academy of Sciences, in the Liaoning Province of China. The soil was air-dried in the dark, passed through a 2-mm sieve to remove roots and stones, and stored at 4 °C for further use. The basic characteristics of the collected soil were as follows: total C 1.78 %, total N 0.11 %, total P 0.035 %, total K 0.604 %, organic matter 2.64 %, and pH 6.8. Initial PAHs were non-detectable.
2.3 Experimental design
2.3.1 Soil spiking and aging
Air-dried soils were spiked with PHE, PYR, and BaP. Spiking standards of PHE, PYR, and BaP were prepared in acetone to deliver concentrations of 100, 100, 50 mg kg−1 respectively (high-level contaminated soil, HCS). To minimize solvent effects, standards of PAHs dissolved in acetone were uniformly sprayed onto a portion (approximately 10 % of soil used in the next stage) of uncontaminated soil. The soil was mixed every day and the acetone was allowed to evaporate before proceeding with the next stage. When the acetone volatilized completely the concentrations required for the low-level contaminated soil (LCS) were obtained by mixing the spiked soil with appropriate amounts of uncontaminated soil to achieve final concentrations of 20, 20, 10 mg kg−1 of PHE, PYR, and BaP, respectively. The spiked soils were sealed in the brown glass jar and stored for 1 year at 4 °C in the dark before they were assessed for desorption.
2.3.2 Desorption of PAHs in aged contaminated soil
Desorption was determined at room temperature (20–23 °C) by means of a XAD-2 adsorption technique described in Northcott and Jones (2001). Approximately 3.5 g of soil and 1 g of wet XAD-2 resin were weighed directly into a 50-ml polycarbonate centrifuge tube. Thirty-five milliliters of 0.01 M CaCl2/0.01 M NaN3 solution were added to prevent microbial growth and the dispersion of soil clay particles. These sealed tubes were placed in an end-over-end rotation shaker (100 rpm) at room temperature (20–23 °C). Triplicate tubes were removed at predetermined times for analysis. Sample tubes were allowed to stand vertically for 5 min to allow settling of the soil-XAD-2-water slurry. Afterwards, 2.7 g of K2CO3 was added to promote phase separation. The resealed tubes were then vigorously shaken for 1 min to mix the ingredients and centrifuged at 4,000 rpm for 15 min to separate the soil and XAD-2. This treatment increased the density of the ionic solution and allowed XAD-2 to float to the surface of the centrifuge tubes. The resin was recovered by vacuum filtration into an inverted glass Pasteur pipette, where it was retained by a plug of glass wool. The XAD-2 within the Pasteur pipette was rinsed with 20 ml of 0.01 M CaCl2/0.01 M NaN3 solution to remove K2CO3 and then dried under vacuum for approximately 2 min.
For each soil, extractions were performed sequentially after 0, 0.5, 1, 2, 4, 8, 24, 48, and 96 h of shaking, in triplicate. At each sampling time, replicate tubes were centrifuged (4,000 rpm), and the PAHs in soil were analyzed. Mass balance was determined by comparing the total amount of compound desorbed plus that remaining in the soil–water suspension to the initial amount in the sample, and was found to vary between 92 and 110 %.
2.4 PAH analysis
Extraction of PAHs in XAD-2 was performed according to Northcott and Jones (2001). After vacuum-drying, the XAD-2 resin was rinsed twice using 20 ml volumes of dichloromethane. PAHs adsorbed to the XAD-2 resin were eluted into a glass beaker and condensed by evaporation of dichloromethane under a stream of nitrogen. The remains were dissolved in 2 ml hexane and filtered through a 0.22-μm filter. The concentrations of PHE, PYR, and BaP were determined by gas chromatography (GC)-FID.
Extraction of PAHs was performed according to Li et al. (2009) where 20 ml of dichloromethane was added to each air-dried soil sample, and samples were extracted by sonication for 2 h. The slurry was centrifuged at 4,000 rpm for 10 min, and 5 ml of supernatant was removed and loaded into a clean-up column with silica gel and anhydrous sodium sulfate. Extracts were condensed by evaporation of dichloromethane under a stream of nitrogen, and the remains were dissolved in 1 ml hexane. The moisture in soil and XAD-2 was determined [by ISO 11465:1993] to allow data to be presented as dry matter.
The concentrations and profiles of PAHs were analyzed by an Agilent 6890(+) GC, equipped with a flame ionization detector. The capillary column used was a DB-5 (30 m × 0.32 mm i.d. × 0.25 μm film thickness). The initial column temperature of 80 °C for 1 min, 15 °C min−1 to 275 °C for 1 min, 10 °C min−1 to 285 °C, and then 5 °C min−1 to 295 °C for 1 min. The temperatures of the injector and detector were 250 °C and 300 °C respectively. The carrier gas was nitrogen at a constant flow rate of 1.5 ml min−1. The injection volume was 10 μl (Li et al. 2009). Three replicates were used in the analysis.
2.5 Data analysis
The following first-order kinetics were used to describe the desorption of PAHs with the collected data, and both the biphasic (two-compartment) and triphasic (three-compartment) models were used to evaluate the desorption, shown in Eqs. (1) and (2), respectively (Cornelissen et al. 1998a; Cornelissen et al. 1998b).
where S t corresponds to the amount of PHE, PYR, or BaP absorbed to the soil at desorption time t (in hour) and S 0 is the total amount of PHE, PYR, or BaP added in soil. F rap, F s, and F vs (%) are the rapidly, slowly and very slowly desorbing fractions respectively, and K rap, K s, and K vs (h−1) are the corresponding rate constants of rapid, slow and very slow desorption. The models assumed that K s was significantly less than K rap. S t /S 0 decreased as desorption time (in hour) increased, and the change of S t /S 0 was lower for longer desorption times. The F rap values used to estimate the rapidly desorbing fractions are believed to be related to bioavailability. Values of F rap, F s, F vs, K rap, K s, and K vs were determined by exponential curve fitting using SigmaPlot 9.0, using a non-linear least squares method.
3 Results
The XAD-2 extraction data were modeled using both biphasic and triphasic desorption models. In Table 2, the desorption fractions and the desorption rate constants of biphasic and triphasic desorption models were given for PHE, PYR, and BaP. Both models fitted the data well, with coefficient of determinations >0.9 except for the biphasic desorption model on high concentration BaP (coefficient of determination = 0.85). However, the triphasic model showed a slightly better fit across all compounds in the two-level concentrations, with an average coefficient of determination of 0.961 ± 0.013 compared to 0.943 ± 0.046 for the biphasic model (see Table 2). Therefore, the results from the triphasic model were discussed further.
3.1 Desorption fractions
Desorption curves for PHE, PYR, and BaP were plotted over a 96 h period (Fig. 1). After 1 year of aging, the range of rapidly desorbing fractions (F rap) for PHE, PYR, and BaP in HCS and LCS were from 1.9 to 27.8 % (see Table 2 and Fig. 1). In HCS, the F rap of PHE, PYR, and BaP after 96 h decreased as the molecular weight of the PAHs increased, and in LCS these were ranked as PHE > BaP > PYR. However, Chai et al. (2006) and Cornelissen et al. (1998b) observed the F rap of PYR to be highest among these PAHs in some native sediments. The F rap of PHE and BaP in HCS were significantly lower than those in LCS, whereas the F rap of PYR was lower in the LCS. However, due to the effect of initial concentration, the high F (F rap, F s, or F vs) (%) of PAH could not indicate if the actual release quantity was larger than the low F (%) of PAH in soil. A sample with a low F rap value in HCS released higher concentrations of PAHs than a sample with a high F rap value in LCS (Hawthorne et al. 2002). The slowly and very slowly desorbing fractions (F s and F vs) were the dominant fractions in these PAH-spiked soils. The F vs of PAHs were usually higher than F s, especially the F vs of PYR in HCS and BaP in LCS, which were 1.2- and 16.1-fold greater than the corresponding F s, respectively.
Desorption of phenanthrene (PHE), pyrene (PYR), benzo(a)pyrene (BaP) from contaminated soil aged for 1 year. S t corresponds to the amount of PHE, PYR, or BaP absorbed to the soil at desorption time t (in hour) and S 0 is the total amount of PHE, PYR, or BaP added in soil. Dotted lines are obtained by triphasic exponential curve fitting
3.2 Desorption rate constants
Based on the literature, the triphasic model rate constants were in the order of 10−1–100 h−1 for K rap, 10−2–10−4 h−1 for K s and 10−4–10−6 h−1 for K vs(Oen et al. 2006, Yang et al. 2008). The rapidly desorbing rate constants (K rap) of PAHs in this study were 1.5–1.8 h−1 in HCS, which is higher than those described in the literature. Conversely, the K rap of PHE and PYR in LCS were lower than those previously described. Compared to the order of F rap, the K rap of PAHs were ranked in order of increasing hydrophobicity in LCS, and as PHE > BaP > PYR in HCS. The K rap of PHE and PYR in HCS were 2 orders of magnitude higher than those in LCS, while the K rap of BaP was still in the same order of magnitude between HCS and LCS. The K s and K vs of PHE or BaP in HCS were equivalent, and the K s of PHE and BaP were 4.05 × 10−3 and 2.69 × 10−4 h−1, respectively. However, the K s of PYR was 9 orders of magnitude higher than K vs of PYR in HCS. In LCS, the K s and K vs of PYR were both equal to 1.1 × 10−4 h−1, and those of PHE were in the same order of magnitude. The K s of BaP was 10 orders of magnitude higher than K vs. The difference of K s and K vs indicated that the desorption sites of BaP in LCS and PYR in HCS were different from other PAHs.
3.3 The release time of 50 % PAHs in the two-level contaminated soils
Table 3 provided an overview of literature values of the time scale for a 50 % release of PHE, PYR, and BaP in the different sorbents according to the biphasic or triphasic model. In this study, the release time of 50 % PAHs in LCS was significantly higher than that in HCS. In HCS, the time scale for release of 50 % PAHs was ranked as BaP > PYR > PHE, whereas this was BaP > PHE > PYR in LCS. van Noort et al. (2003) also observed that the time scale of release of 50 % PAHs was ranked as BaP > PHE > PYR. The time scale of release of 50 % PHE, PYR, or BaP in HCS was lower than in other studies, while the time scale of release was higher in LCS than other studies except for those of PYR, which was lower than those of PCB200, PCB2000, NVS, and VS (see Table 3). This could be due to different soil aging times, sorbents and initial concentrations (extractable concentration in field and total in laboratory) (Cornelissen et al. 1997; Griffiths 2004).
4 Discussion
The extent of desorption for organic contaminants is a crucial factor in determining their bioavailability and toxicity in soil and sediment (Cornelissen et al. 1998b; Lin et al. 2011). The component change of organic contaminants during desorption caused by different desorption rates is crucial for selecting the dominant organisms for contaminated soil bioremediation (Cebron et al. 2011). Therefore, the current study examined the effects of PAH concentration on desorption when PHE, PYR, and BaP were present simultaneously.
The desorption kinetics in which the rapid, slow and very slow desorption stages are observed have gained much attention because this process indicates the bioavailability of contaminants in soil (Hawthorne et al. 2002). Three desorption rate constants correspond to these stages, which are ranked as K rap > K s > K vs, and at least a difference of one order of magnitude exist among them. However, only the K s of PYR in HCS and BaP in LCS were significantly higher than the corresponding K vs in present study, and the K s of other PAHs were similar or equal to their K vs. This might indicate that an aging period of 1 year was insufficient for them to go into the condensed organic matters-the difficult desorption sites except for PYR in HCS, PHE (the lower desorption rate constant than average) and BaP in LCS. Chai et al. (2006) found that the laboratory-spiked contaminants to age for periods up to 3 years yielded little difference in the rapidly desorbing fraction which of them was both higher than 90 %. The similar phenomenon was also founded in the study of Ghosh et al. (2001). In the present study, samples had the same aging time and soil properties, therefore differences in concentrations and the molecular properties of the PAHs were the dominant cause of differing desorption rates.
The effect of contaminant concentration on desorption in soil has been previously reported in many studies (Braida et al. 2002; Gao et al. 2010). Many authors concluded that concentration was a key factor for desorption rates, with high F rap or K rap values observed at high PAH concentrations in contaminated soil (Braida et al. 2002; Jonker et al. 2005; Yang et al. 2008). When the contamination level was high, either a large number of the contaminant molecules were located on “easily desorbing sites”, leading to an enhancement in the rapid desorption phase, or matrix binding sites were saturated, leaving most PAH molecules highly available (Zhou et al. 2010). However, some studies have also reported that the wide range in desorption rate constants for various samples was not always based on contaminant concentration (Hawthorne et al. 2002; Nichols et al. 2008; Oen et al. 2006; You et al. 2007). Relatively high concentrations might limit the observable influence of the desorption resistant fraction of contaminants, and the importance of the desorption–resistant fraction can be largest at low concentrations (Chai et al. 2006; Cornelissen et al. 2005). In the present study, desorption rate constants of PAHs in HCS were higher than those in LCS, with the exception of the K vs for PYR, and the K s and K rap for BaP. Compared to the F rap of PHE and BaP, the F rap of PYR in HCS was the only one higher than that in LCS. This implied that PAH concentration alone was inadequate for explaining the differences in fraction and rate constant between HCS and LCS.
The properties of PAHs might be another factor which had an effect on their desorption kinetics in the present study at the same soil. Differences of desorption rate constants between K s and K vs for PYR in HCS indicated these had entered the difficult desorption sites in advance as well as PHE and BaP in LCS. This is similar to other studies where a lower extent of desorption was observed for less hydrophobic PAHs, such as two- and three-ring PAHs, compared to more hydrophobic ones (Cornelissen et al. 1998b; Cornelissen et al. 2001; Hawthorne et al. 2002; Nichols et al. 2008; Oen et al. 2006; Shor et al. 2003; Yang et al. 2008). Samples used in those studies were predominantly collected in the field. Hence, the reasons for lower extent of desorption of less hydrophobic PAHs were always attributed to their release or degradation in these highly weathered sediments. The lower hydrophobic PAHs might have already been depleted relative to the higher hydrophobic, lower biodegradable PAHs due to the long time weather (Oen et al. 2006; Shor et al. 2003). If lower hydrophobic PAHs remained at high concentration, they could be sequestered in nanopores, trapped in small voids, or bound in high-energy sites and would therefore be unavailable for desorption (Oen et al. 2006; Shor et al. 2003; Zhou et al. 2010). However, in the current study samples were laboratory-spiked and no biodegradation existed during the storing. Thus, the properties of PAHs should be the dominant factors causing the observed desorption constants of PYR which was lower than that of BaP.
Desorption can be defined as the opposite of adsorption, with the aqueous concentration and contact time of sorption significantly altering the release of organic contaminants (Pan et al. 2011). In general, the adsorption of PAHs is a combination of two forces: van der Waals forces and a thermodynamic gradient determined by hydrophobicity, which drives them out of the solution (Brion and Pelletier 2005). Based on the above discussion, our results indicate the difference of adsorption could be expressed in terms of desorption. The initial PAH concentration should be taken into account because the difference in diffusion rates, as determined by the concentration of PAHs, could result in different absorption rates, especially in the initial stage. For example, based on the desorption constant of PYR, we could assume that the absorption of PYR is faster than BaP and PHE, and that high-energy sites were bounded first by PYR, causing desorption of PYR to be slower than BaP. It was different with general phenomenon in which the absorption of PAHs in soil increased significantly with the increasing molecular weights of PAHs (Guilloteau et al. 2010). Meanwhile, the dominant factors affecting absorption changed at different stages. The results observed by Shor et al. (2003) indicated that fast-domain diffusivity was affected by both PAH hydrophobicity and sediment properties, and slow-domain diffusivity was affected by only sediment physicochemical properties. However, the principle model of PAH adsorption and desorption under different spiked concentrations still need further research in the same soil.
5 Conclusions
The effect of PAH concentration and property on desorption differed with soil contamination and aging time. In this study, it was observed that the K vs of PYR was the lowest in HCS and the K s and K vs of PYR were the highest in LCS after 1 year aging of PAH in meadow brown soil. The desorption of PAHs did not always decrease with increasing molecular weight and the desorption of four-ring PAHs might be special. Hence, the combined effect of PAH concentrations and properties should be taken into account when modeling adsorption and desorption. These data will be useful for determining the dominant contaminants, screening degrading microbes and determining at what stage these microbes should be added during bioremediation.
References
Braida WJ, White JC, Zhao D, Ferrandino FJ, Pignatello JJ (2002) Concentration-dependent kinetics of pollutant desorption from soils. Environ Toxicol Chem 21:2573–2580
Brion D, Pelletier É (2005) Modelling PAHs adsorption and sequestration in freshwater and marine sediments. Chemosphere 61:867–876
Cebron A, Cortet J, Criquet S, Biaz A, Calvert V, Caupert C, Pernin C, Leyval C (2011) Biological functioning of PAH-polluted and thermal desorption-treated soils assessed by fauna and microbial bioindicators. Res Microbiol 162:896–907
Chai Y, Kochetkov A, Reible DD (2006) Desorption resistance of polycyclic aromatic hydrocarbons and duration of exposure. Environ Toxicol Chem 25:2827–2833
Colombo M, Cavalca L, Bernasconi S, Andreoni V (2011) Bioremediation of polyaromatic hydrocarbon contaminated soils by native microflora and bioaugmentation with Sphingobium chlorophenolicum strain C3R—a feasibility study in solid-and slurry-phase microcosms. Inter Biodeter Biodegr 65:191–197
Cornelissen G, Noort PC, Parsons JR, Govers HA (1997) Temperature dependence of slow adsorption and desorption kinetics of organic compounds in sediment. Environ Sci Technol 31:454–460
Cornelissen G, Pv N, Govers H (1998a) Mechanisms of slow desorption of organic compounds from sediments: a study using model sorbents. Environ Sci Technol 32:3124–3131
Cornelissen G, Rigterink H, Ferdinandy MMA, Noort PCM (1998b) Rapidly desorbing fractions of PAHs in contaminated sediments as a predictor of the extent of bioremediation. Environ Sci Technol 32:966–970
Cornelissen G, Rigterink H, Hulscher DE, Vrind BA, Noort PC (2001) A simple Tenax extraction method to determine the availability of sediment-sorbed organic compounds. Environ Toxicol Chem 29:706–711
Cornelissen G, Gustafsson O, Bucheli T, Jonker M, Koelmans A, Pv N (2005) Extensive sorption of organic compounds to black carbon, coal, and kerogen in sediments and soils: mechanisms and consequences for distribution, bioaccumulation, and biodegradation. Environ Sci Technol 39:6881–6895
Gao Y, Ren L, Ling W, Gong S, Sun B, Zhang Y (2010) Desorption of phenanthrene and pyrene in soils by root exudates. Bioresour Technol 101:1159–1165
Ghosh U, Talley JW, Luthy RG (2001) Particle-scale investigation of PAH desorption kinetics and thermodynamics from sediment. Environ Sci Technol 35:3468–3475
Griffiths RA (2004) Sorption and desorption by ideal two-compartment systems: unusual behavior and data interpretation problems. Chemosphere 55:443–454
Guilloteau A, Bedjanian Y, Nguyen M, Tomas A (2010) Desorption of polycyclic aromatic hydrocarbons from a soot surface: three- to five-ring PAHs. J Phys Chem A 114:942–948
Hawthorne SB, Poppendieck DG, Grabanski CB, Loshr RC (2002) Comparing PAH availability from manufactured gas plant soils and sediments with chemical and biological tests. 1. PAH release during water desorption and supercritical carbon dioxide extraction. Environ Sci Technol 36:4795–4803
Jonker MTO, Hawthorne SB, Koelmans AA (2005) Extremely slowly desorbing polycyclic aromatic hydrocarbons from soot and soot-like materials: evidence by supercritical fluid extraction. Environ Sci Technol 39:7889–7895
Li X, Lin X, Li P, Liu W, Wang L, Ma F, Chukwuka K (2009) Biodegradation of the low concentration of polycyclic aromatic hydrocarbons in soil by microbial consortium during incubation. J Hazard Mater 172:601–605
Lin T, Pan P, Young C, Chang J, Chang T, Cheng S (2011) Evaluation of the optimal strategy for ex situ bioremediation of diesel oil-contaminated soil. Environ Sci Pollut R 18:1487–1496
Lors C, Ryngaert A, Périé F, Diels L, Damidot D (2010) Evolution of bacterial community during bioremediation of PAHs in a coal tar contaminated soil. Chemosphere 81:1263–1271
Nichols EG, Gregory ST, Musella JS (2008) The impact of vegetation on sedimentary organic matter composition and PAH desorption. Environ Pollut 156:928–935
Northcott GL, Jones KC (2001) Partitioning, extractability, and formation of nonextractable PAH residues in soil. 2. Effects on compound dissolution behavior. Environ Sci Technol 35:1111–1117
Oen AMP, Breedveld GD, Kalaitzidis S, Christanis K (2006) How quality and quantity of organic matter affect polycyclic aromatic hydrocarbon desorption from Norwegian harbor sediments. Environ Toxicol Chem 25:1258–1267
Pan B, Tao S, Wu D, Zhang D, Peng H, Xing B (2011) Phenanthrene sorption/desorption sequences provide new insight to explain high sorption coefficients in field studies. Chemosphere 84:1578–1583
Rhodes AH, McAllister LE, Semple KT (2010) Linking desorption kinetics to phenanthrene biodegradation in soil. Environ Pollut 158:1348–1353
Shor LM, Rockne KJ, Taghon GL, Young LY, Kosson DS (2003) Desorption kinetics for field-aged polycyclic aromatic hydrocarbons from sediments. Environ Sci Technol 37:1535–1544
Singleton DR, Richardson SD, Aitken MD (2011) Pyrosequence analysis of bacterial communities in aerobic bioreactors treating polycyclic aromatic hydrocarbon-contaminated soil. Biodegradation 22:1061–1073
van Noort PCM, Cornelissen G, Hulscher TEM, Vrind BA, Rigterink H, Belfroid A (2003) Slow and very slow desorption of organic compounds from sediment: influence of sorbate planarity. Water Res 37:2317–2322
Yang Y, Cajthaml T, Hofmann T (2008) PAH desorption from river floodplain soils using supercritical fluid extraction. Environ Pollut 156:745–752
You J, Pehkonen S, Landrum PF, Lydy MJ (2007) Desorption of hydrophobic compounds from laboratory-spiked sediments measured by Tenax absorbent and matrix solid-phase microextraction. Environ Sci Technol 41:5672–5678
Zhou Z, Sun H, Zhang W (2010) Desorption of polycyclic aromatic hydrocarbons from aged and unaged charcoals with and without modification of humic acids. Environ Pollut 158:1916–1921
Acknowledgments
This study was supported by National Scientific Foundation of China (41101295), National Scientific Foundation of Liaoning Province (201102226) and Open Foundation of Key Laboratory of Industrial Ecology and Environmental Engineering (MOE) (KLIEEE-09-04).
Author information
Authors and Affiliations
Corresponding author
Additional information
Responsible editor: Jan Schwarzbauer
Rights and permissions
About this article
Cite this article
Li, X., Shuang, S., Li, X. et al. The effect of concentrations and properties of phenanthrene, pyrene, and benzo(a)pyrene on desorption in contaminated soil aged for 1 year. J Soils Sediments 13, 375–382 (2013). https://doi.org/10.1007/s11368-012-0629-3
Received:
Accepted:
Published:
Issue Date:
DOI: https://doi.org/10.1007/s11368-012-0629-3