Abstract
Agriculture productivity is severely affected by soil salinity. One possible mechanism by which plants could survive salt stress is to compartmentalize sodium ions away from the cytosol. In the present work, transgenic buckwheat plants overexpressing AtNHX1, a vacuolar Na+/H+ antiporter gene from Arabidopsis thaliana, were regenerated after transformation with Agrobacterium tumefaciens. These plants were able to grow, flower and accumulate more rutin in the presence of 200 mmol/l sodium chloride. Moreover, the content of important nutrients in buckwheat was not affected by the high salinity of the soil. These results demonstrated the potential value of these transgenic plants for agriculture use in saline soil.
Similar content being viewed by others
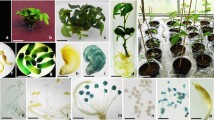
Avoid common mistakes on your manuscript.
Introduction
Buckwheat is a dicotyledonous crop of the Polygonaceae family. In general, only two species of buckwheat, common buckwheat (Fagopyrum esculentum) and tartary buckwheat (Fagopyrum tartaricum) are used as food around the world (Bonafaccia et al. 2003). Buckwheat seeds contain 10–12.5% protein and have high nutritional value (Greenway et al. 1980). Flavones, flavonoids, sterols, fagopyrin, and thiamin-binding proteins in buckwheat seeds possess potential effects in treating some chronic diseases (Kreft et al. 2006). Flavonoids are a group of polyphenolic plant secondary metabolites important for plant biology and human nutrition. In these compounds, flavonols are potent antioxidants, and their dietary intake is correlated with a reduced risk of cardiovascular diseases (Bovy et al. 2002 ). In buckwheat, the main component of flavonoids (80%) is rutin, a flavonol glycoside (quercetin 3-rhamnosylglucoside). Rutin can antagonize the increase of capillary fragility associated with haemorrhagic disease (Im et al. 2003; Kreft et al. 1999), reduce high blood pressure (Abeywardena et al. 2001), decrease the permeability of the blood vessels, reduce the risk of arteriosclerosis (Wojcicki et al. 1995), have an anti-oedema effect and antioxidant activity (Holasova et al. 2001; KrkosKova et al. 2005; Park et al. 2000; Watanabe 1998). However, buckwheat is a salt-sensitive glycophyte cereal crop and the growth and grain yields of buckwheat are significantly affected by soil salinity. Therefore, cultivation of buckwheat with higher salt tolerance would produce remarkable economic and medicinal benefits.
Soil salinity is one of the major environmental factors limiting agricultural productivity in many regions of the world. The reason is that most crop species are glycophytes, which are usually salt sensitive. High Na+ concentration in the cytosol is detrimental to plant growth and leaves are usually more susceptible to Na+ toxicity than roots. Many metabolic and physiological processes can be inhibited by high concentration of Na+ accumulated in the cytoplasm and possibly also in the nucleus, including the activities of enzymes and transcription factors (Xue 2002). Tolerance of some glycophytes to soil salinity is achieved by either extruding Na+ out of cells or sequestering Na+ into the vacuole, and Na+/H+ antiporters in plasma membrane and tonoplast play a vital role in these processes. The functions of maintaining Na+ out from the cytoplasm of plant cells and maintaining low cytosolic Na+ concentrations under salinity conditions (Blumwald et al. 2000) is accomplished by pumping Na+ either out of cells (plasma membrane antiporter) or into vacuole (vacuolar antiporter) in exchange of H+. Although studies on the activity of Na+/H+ antiporter in plant cells started as early as in 1976 (Ratner et al. 1976), only recently, plasma membrane and vacuolar Na+/H+ antiporter genes were identified in plants (Apse et al. 1999; Gaxiola et al. 1999; Shi et al. 2000; Tao et al. 2002). It has been proposed that compartmentalization of Na+ into the vacuole, through the action of vacuolar Na+/H+ antiporters, provides an efficient mechanism to avert the deleterious effects of Na+ in the cytoplasm. This compartmentalization process can also maintain intracellular osmotic potential by the use of Na+ as an osmoticum to drive water into the cells (Blumwald et al. 2000). Furthermore, the vacuolar volume of plant cells can increase during salt stress (Mimura et al. 2003), thus further enhancing the capacity of plant cells to sequester Na+ into the vacuole.
Improvement of the salt tolerance of crops has been tackled by traditional breeding (Shannon 1997; Schachtman et al. 1992). Until recently, with the development of genetic transformation technology of many plant species, a new engineering approach by introduction of a specific gene has been employed to achieve the potentially radical improvement of plant salt tolerance. A novel and direct strategy was carried out by the manipulation of Na+/H+ antiporter genes to reduce the Na+ level in the vital subcellular sites of plant cells. Apse et al. (1999) found that the overexpression of a vacuolar Na+/H+ antiporter gene from Arabidopsis thaliana, AtNHX1, significantly enhanced the salt tolerance of transgenic Arabidopsis. The effectiveness of overexpressing a vacuolar Na+/H+ antiporter gene in improving salt tolerance has also been shown in tomato (Zhang and Blumwald 2001), Brassica napus (Zhang et al. 2001), rice (Ohta et al. 2002), Perennial ryegrass (Wang et al. 2005) and wheat (Zhe et al. 2004) under greenhouse experimental conditions. These studies demonstrated the important role of Na+/H+ antiporters in the salt tolerance of plants and genetic manipulation of Na+/H+ antiporter gene can be adopted to increase agricultural productivity in saline soils.
In vitro regeneration via adventitious shoot organogenesis represents an ideal alternative, not only for clonal propagation, but also for genetic transformation of plants. Studies on plant regeneration of buckwheat have been conducted (Jin et al. 2002) and improved by modifying the tissue culture technique (Chen et al. 2006). However, there is still no report about genetic transformation in buckwheat. Further improvement of buckwheat regeneration system and establishment of a high-efficiency transformation system would make a significant contribution to buckwheat genetic engineering.
The purpose of this study was to optimize conditions for producing organogenic calli and regenerating plants from hypocotyls and cotyledon segments, and to develop an efficient transformation protocol that can be used for genetic transformation and other fundamental studies. Meanwhile, we have examined whether salt tolerance in buckwheat can be improved by enhancing the level of vacuolar Na+/H+ antiporters. A vacuolar Na+/H+ antiporter gene from Arabidopsis, AtNHX1, driven by a constitutive promoter was introduced into buckwheat via Agrobacterium tumefaciens and several parameters were measured for the evaluation of the salt tolerance of transgenic plants. Our results showed that the salt tolerances of the transgenic buckwheat plants over-expressing the Na+/H+ antiporter gene had dramatically been improved in comparison with the wild type plants.
Material and methods
Plant material
Seeds of buckwheat cultivar “PingLiang” were provided by Gan Su Academy of Agricultural Science. Plant expression vector pHZX1 harboring the Na+/H+ antiporter gene (AtNHX1) was kindly provided by Dr. HX Zhang of National Institute of Plant Physiology, in Shanghai. The AtNHX1 gene was under the control of the cauliflower mosaic virus (CaMV) 35S promoter, and the terminator region contained the polyadenylation signal of the nopaline synthetase gene (Nos). The plasmid could be identified by digestion with BamHI.
Callus induction and plant regeneration
Seeds were surface-sterilized with 70% ethanol for 30 s and then 0.1% HgCl2 for 12 min. After rinsing four to five times with sterile distilled water, seeds were placed on MS medium to germinate at moderate light intensity (1000 lux: 16 h day/8 h night). The cotyledons and hypocotyls were dissected aseptically from the sterile seedlings (10–12 days) and cultured in precuture medium containing 2 mg/l 2, 4-D, 1.5 mg/l 6-BA, 600 mg/l casein hydrolysate (CH) and 300 mg/l yeast extract (YE) to induce the calli in darkness for 7–12 days. The well-grown calli were transferred to regeneration medium supplemented with 2.0 mg/L 6-BA, 1.0 mg/L KT, 0.1 mg/L IAA, 600 mg/l CH and 300 mg/l YE and sub-cultured (1000 lux: 16 h day/8 h night) for 4–6 weeks at 2 week intervals. Green shoots were transferred onto root-inducing medium containing 1/2MS, 1.0 mg/L IBA and 0.5 mg/l NAA and illuminated at 5000 lux. All media were based on MS with 0.75% agar and 30 g/l sucrose. The pH was adjusted to 5.8–6.2 prior to autoclaving at 121°C and 1.2 bars for 25 min.
Effects of acetosyringone (AS)
Agrobacterium tumefaciens strain LBA4404 containing pHZX1 binary vector was used in transformation experiments. To study the effects of AS on transformation efficiency, AS was added at different transformation stages: A, without AS in transformation process; B, AS was added only in the incubation process with LBA4404; C, AS was added in callus preculture, incubation with LBA4404 and co-culture process. The AS concentration used in all the tests was 200 mmol/L.
Buckwheat transformation
A single colony of LBA4404 was picked into a test tube containing YEB liquid medium (5.0 g/L Difco Bacto beef extract, 5.0/L g tryptone, 5.0 g /L sucrose, 1.0 g/L YE, 0.5 g/L Mg2SO4·7H2O, 50 mg/L kanamycin), and incubated at 27 ∼ 29. 8°C with shaking (200 rpm) overnight. The bacterium suspension were transferred into 100 ml YEB liquid medium containing 200 mmol/L AS and cultured for 7 ∼ 8 h until the density of Agrobacterium (OD600) achieved 0.5 ∼ 0.8. Then the bacteria were pelleted by centrifugation at 12,000 rpm for 1 min and resuspended in AAM liquid medium (MS plus 3% sucrose and 200 mmol/l AS, pH 5.2), the OD600 was adjusted to 0.5 ∼ 1.0. Fresh calli precultured for 0 ∼ 4 days were immersed in Agrobacterium suspension for 8 ∼ 25 min in the dark. Excess bacteria on the calli surface were removed with sterilized filter paper and the calli were co-cultured (preculture medium containing 200 mmol/L AS) at 25°C in the dark for 1 ∼ 4 days. Then the infected calli were rinsed with sterile water for 5 to 6 times, and subcultured on selection medium (regeneration medium supplemented with 50 mg/L kanamycin, 200 mg/L cefotaxime) at 2 week intervals. Approximately 6 weeks later, survived shoots were transferred onto root-inducing medium to induce roots.
PCR and Southern blotting analysis
PCR was adopted to verify the insertion of AtNHX1 gene in the genomic DNA of putative transgenic plants, with a primer pair of 5′-ACTCACCTAAACCACGAAGC-3′ and 5′-CAGA CCACCAAATCACAACC-3′. Thirty cycles (95°C for 30 s, 53°C for 1 min and 72°C for 1 min) were performed using rTaq polymerase (TianWei, China). Integration of AtNHX1 gene was further confirmed by Southern blotting analysis. Ten milligram of genomic DNA of each putative transgenic line was digested with different restriction endonucleases and separated by electrophoresis in 1.0% agarose gel, then transferred to nylon membrane and hybridized with Dig-labeled AtNHX1 gene probe. The probe was a 1.6 Kb PCR product of AtNHX1 gene through PCR. The hybridization was carried out as previously described (Sambrook et al. 1989).
RT-PCR analysis of AtNHX1 expression in transgenic buckwheat
Reverse transcription PCR (RT-PCR) was performed using total RNA pre-treated with RNase-free DNase I as described by Li et al. (2004). One microgram of total RNA from each sample was used in a 10 μl RT reaction system with oligo dT as primer. One microlitre of the RT reaction mixture after the completion of cDNA synthesis was used for amplification of AtNHX1 in a 20 μl PCR system and the conditions were as mentioned above.
Northern blotting hybridization
Total RNA for gel-blot analysis was isolated from putative transgenic plant tissues according to the method of Li et al. (2004). Fifteen microgram of RNA was fractionated in 1% (v/v) formaldehyde agarose gel before transfer to nylon membrane and hybridization with Dig-labeled AtNHX1 fragment. Hybridization was performed at 65°C in 0.25 mmol/L sodium phosphate buffer containing 7% (w/v) SDS, 1% (w/v) BSA, 1 mmol/L EDTA and pH 8. Blot was washed at 65°C, twice with 2 × SSC and 0.1% (w/v) SDS, twice with 0.2 × SSC and 0.1% (w/v) SDS (1 × SSC = 0.15 mol/L NaCl, 0.015 mol/L sodium citrate, pH 7), and autoradiographed with an intensifying screen at 37°C for 30 min.
Determination of Na+, K+ and proline concentrations in leaf and root
Different transgenic lines (Tr) and wild-type lines (CK) of buckwheat were cultured on MS medium for 2 weeks and then treated with MS solution containing 0–200 mmol/l NaCl (0, 50, 100 and 200 mmol/l) for 3 days. Roots were rinsed with distilled water, then the leaves and roots of each treatment were collected and dried at 70°C. Na+ and K+ concentrations were measured by atomic absorption spectrum (SOLAR M6, IRIS Advantage ICP, Instrument, America). The proline concentrations were determined as described by Bates et al. (1973). Leaf and root segments were homogenized with 3% sulfosalicylic acid and the homogenates were centrifuged at 3,000g for 20 min. The supernatant was treated with acetic acid and acid ninhydrin, after boiling for 1 h, the absorbance at 520 nm was determined. Contents of proline are expressed as μmol g−1 FW.
Determination of rutin concentrations in leaf and root
Samples were quickly ground to a fine powder using a mortar that had been dried at 200°C and rutin was then extracted with 1 ml of methanol per 0.05 mg dry sample at 50°C for 3 h. The centrifuged supernatant was filtered through a 0.2 mm PTEF membrane (Iwaki Co. Ltd., Japan) and the filtrate was used for HPLC (Suzuki et al. 2002).
Analysis of rutin contents in buckwheat roots, stems and leaves were performed on Waters 2690 separation module (Waters Milford, USA) equipped with a photodiode array detection system Waters 996. The column used was a symmetry C18, 5 μm (150*3.9 mm) from Waters, and the operation temperature was 25°C. The mobile phase consisted of 0.5% acetic acid in water (eluent A) and of 100% methanol (eluent B). The gradient program was 6–50% A and 40–50% B. The injection volume for all samples was 10 μl. Spectra were recorded from 200 to 450 nm at a flow-rate of 0.2 ml/min (Kim et al. 2004).
Results
Effects of preculture time and explant type on transformation frequency of buckwheat
As shown in Fig. 1, the transformation frequencies changed with the preculture time and explant type were not the same. Explants became more sensitive for the integration of T-DNA with increased periods of preculture. The results demonstrated that the transformation frequencies of cotyledon and hypocotyl explants could be improved by preculture for 1–2 days in comparison with the explants without undergoing preculture. The highest transformation frequencies of cotyledon and hypocotyls were observed after 2 days preculture. However, along with prolonged preculture time, the transformation frequencies decreased. It is possible that the injury to explants could become serious with long preculture time decreasing explants’ viability. Besides, the explants would wither easily in the absence of a preculture process. Therefore, only after suitable preculture, the transformation frequency of buckwheat by Agrobacterium could amount to the best. However, these results were not fit for all the plants. When Kim et al. (2004) used Agrobacterium to infect explants of Perilla frutescens, they found that the transformation frequency without preculture was much higher than that of the explants which were precultured for a period of time. Figure 1A also showed that the transformation frequencies of hypocotyl explants were much higher compared with the cotyledon explants, suggesting that the sensibility of explants to Agrobacterium was changeable due to the difference of cell growth age and physiological state from different tissue in the same plant.
Effects of the Agrobacterium infection and co-culture time on transformation frequency of buckwheat
Two other important factors affecting the transformation of buckwheat were the time of infection and co-culture. When the cotyledon segments were infected with Agrobacterium for 20 min and hypocotyl segments for 25 min respectively, the transformation frequency of these two explants came up to the highest (Fig. 1B). The co-culture time was also very critical to the transformation of buckwheat. When the explants without undergoing a co-culture process were directly transferred to the selection medium, the transformation frequency was very low since Agrobacterium did not proliferate enough and would be killed by cefotaxime (Cef) quickly. In contrast, if the co-culture time was too long, the transformation frequency would also decrease due to the serious cell damage caused by Agrobacterium. In this study, we found that when the time of co-culture exceeded 2 days, the following inhibition of bacterium would be very difficult and the consequences included increase of Cef expenditure, pollution of plant materials and descent of transformation frequency. As shown in Fig. 2A, co-culture for 1 ∼ 2 days with Agrobacterium was suitable for the transformation of buckwheat explants.
Effects of AS on transformation of buckwheat hypocotyl callus
Acetosyringone is thought to be the external signal molecule which can induce expression of vir genes. It is the key factor in Agrobacterium-mediated transformation of cereal crops. To increase the transformation frequency of buckwheat, AS was added at different stages during the process of experiments (Fig. 2B). High regeneration frequency of resistant green shoots could be obtained when AS was supplemented during the incubation of explants with bacteria and the co-culture processes. But the transformation frequency did not increase significantly compared with that without AS in the whole transformation process. Moreover, AS is a very expensive chemical compound, so it was unnecessary to add AS in the process of buckwheat transformation.
Production of transgenic buckwheat
Mature seeds were sterilized and then cultured on MS medium for 10 ∼ 12 days. The cotyledons and hypocotyls were dissected into 1–5 mm and were used as the explants. Explants precultured for 2 days were infected and co-cultured for 1–2 days with Agrobacerium on the same medium. Agrobacterium was eliminated with Cef in subsequent culture process. The yellowish and non-friable calli formed after 7–10 days of culture (Fig. 3A). 73.6% of the calli could turn into yellowish, nodular, and friable embryonic calli (Fig. 3B) which were capable to regenerate plants. Calli were then transferred onto the regeneration medium. Regenerated healthy green shoots were transferred into rooting medium and almost all of them generated strong roots in 1–2 weeks (Fig. 3C). Rooted shoots were adapted for 2 ∼ 3 days by opening the lids of the containers and then transplanted to soil.
Plant regeneration of transgenic buckwheat. (A) The yellowish or slight red loosen calli induced after 15 days from hypocotyl explants on MS medium supplemented with 2.0 mg/L 2,4-D and 1.0 mg/L 6-BA; (B) Green adventitious shoots regenerated after 30 days on differentiation MS medium supplemented with 2 mg/L 6-BA, 0.1 mg/L IAA and 1 mg/L KT; (C) The roots formed after 10 days from adventitious shoots on 1/2 MS with 1.0 mg/L IBA and 0.5 mg/L NAA; (D), The transgenic plants grew vigorously in the presence of 200 mmol/L NaCl afer 21 days; (E) The wild-type plants withered in the presence of 200 mmol/L NaCl after 21 days
Molecular identification of the transgenic plants
A total of 5 transgenic lines carrying the AtNHX1 gene were identified by PCR amplification of a 559 bp fragment of AtNHX1 (B1–B5, Fig. 4A). Four independent transgenic lines (B1–B4) were selected for Southern blotting analysis. Total genomic DNA was extracted from the leaves of the transformed and untransformed plants by SDS. The genomic DNA was digested respectively with EcoRI and HindIII which cut at a unique site within the T-DNA, and the pHZX1 plasmid was digested with EcoRI. As shown in Fig. 4B, the transgenic plants B1–B4 showed single integration event of the AtNHX1 gene thereby confirming their transgenic nature. No hybridization signal was observed in the untransformed sample (Fig. 4B, lane 2).
Identification of transgenic buckwheat. (A) PCR analysis of AtNHX1: Ck, wild type buckwheat were used as negative control; P, plasmid control; B1–B5, independent transgenic buckwheat lines. (B) Southern blotting analysis of transgenic buckwheat lines: P, plasmid pHZX1 digested with EcoRI as positive control; Ck, wild type buckwheat digested with EcoRI; B1 and B3, independent transgenic buckwheat lines digested with EcoRI; B2 and B4, independent transgenic buckwheat lines digested with HindIII. (C) RT-PCR analysis of transgenic buckwheat lines: Ck, wild type buckwheat, B1–B5, independent transgenic buckwheat lines. (D) Northern blotting analysis of transgenic buckwheat lines: Ck, wild type buckwheat; B1–B5, independent transgenic buckwheat lines
The transgenic lines (B1–B5) positive in PCR identification were selected for expression analysis of the CaMV 35S promoter-driven AtNHX1 using RT-PCR and primers specific for AtNHX1 cDNA based on their relatively high salt tolerance. An AtNHX1-specific band with the expected size (559 bp) was amplified from DNase-treated RNA prepared from 1-week-old seedlings of transgenic lines B1–B5 (Fig. 4C), but not from wild-type line. To analyze mRNA accumulation of the introduced AtNHX1 gene, five independent transgenic lines (B1–B5) were detected by RNA gel blot analysis. Northern analysis showed that the mRNAs of AtNHX1 could be accumulated effectively in the transgenic buckwheat plants (Fig. 4D), and mRNA levels varied among independent transformants.
Determination of Na+, K+ and proline concentrations in leaf and root of transgenic buckwheat
One transgenic line (B1) and wild-type plants of buckwheat were treated with different concentrations of NaCl. The concentrations of Na+, K+ and proline in leaves and roots were measured. The Na+ concentration in the leaves and roots of both transgenic and wild-type plants increased as NaCl concentration increased. However, at the range of 50–200 mmol/l NaCl, the transgenic line preserved more Na+ in leaves (Fig. 5B) and roots (Fig. 5A) than wild-type plants except in roots treated with 50 mmol/l NaCl. For example, at 200 mmol/l NaCl, the Na+ concentrations in leaves and roots of transgenic line were 7.12 and 4.54 mg/g dry weight (DW), respectively, while they were only 4.62 and 2.04 mg/g DW respectively in the wild-type plants. It indicated that the transgenic line absorbed more Na+ than wild-type in the same condition. Despite showing high Na+ content in leaves when the transgenic plants were stressed with 200 mmol/l NaCl, these plants were able to grow, flower, and set seeds. These results clearly demonstrated overexpression of the vacuolar Na+/H+ antiporter could enhance the accumulation of Na+. It should be noted that although our experiments were carried out in the greenhouse, our results were obtained with a relatively low humidity and high light intensity. The K+ content in roots and leaves of transgenic line and wild-type plants decreased as the NaCl concentration increased. Although there were significant differences between leaves of wild type and transgenic plants at each NaCl level tested (Fig. 5D), there was no significant difference between the transgenic lines and wild-type plants at each NaCl level (Fig. 5C) in roots. Under the same NaCl concentration, the leaves and roots of the wild-type plants contained more K+ than that of transgenic line. Adaptation of plants to saline environments not only depends on their ability to avert the toxic effects of Na+ per se, but also on their ability to overcome salt-induced injury and it closely related with K+ uptake and K+ homeostasis. K+ concentrations in plant cells are kept under homeostatic control with cytosolic K+ concentrations in the order of 100–200 mmol/l. When exposed to relatively low NaCl concentrations, Na+ can promote growth of many plants at low K+ concentrations in the growth medium. Under high-salinity conditions, Na+ may displace K+ from its carrier binding sites and this competition results in impaired K+ uptake and lower K+ cytosolic concentrations. Nevertheless, the growth of the transgenic plants was not significantly affected by high salinity, suggesting that K+ nutrition was not compromised in the transgenic plants. Table 1 showed that the proline content in transgenic line were higher than wild-type at each NaCl level both in roots and leaves. Transgenic plants were stressed with 100 mmol/l NaCl displayed 1.60 (root) and 1.73 (leaf) fold increase in proline content compared with wild-type plant. The accumulation of proline in response to high salinity has been well-documented. Proline contributes to osmotic adjustment, protection of macromolecules during dehydration, and as hydroxyl radical scavenger.
Salt-tolerance of the transgenic buckwheat plants
One transgenic line (B1) and wild-type buckwheat plants were transplanted to plastic pots and watered with a nutrient solution. When the buckwheat plants grew vigorously, they were irrigated with a nutrient solution containing 0–200 mmol/L NaCl 21 days later, the growth of the wild-type and transgenic buckwheat plants were affected by salt stress, and the inhibitory effect correlated with NaCl concentration (Table 2). When the concentration of NaCl amounted to 200 mmol/L, the wild-type plants gradually withered and died after 21 days (Fig. 3D–E) whereas the transgenic lines survived and continued growing. These results demonstrated that the transgenic line was less-inhibited by salt stress compared to wild-type plants, showing that overexpression of the Na+/H+ antiporter cDNA can indeed improve salt-tolerance.
Determination of rutin concentrations in leaf, stem and root
Buckwheat is a plant that produces rutin, a compound known for its pharmacological effects. The following actions of rutin make it a potential anti-cancer agent. They include cell cycle regulation, interaction with type II estrogen binding sites, and tyrosine kinase inhibition. In addition, rutin appears to be non-toxic (Davis and Matthew 2000). To investigate the changes of rutin contents after overexpressing the vacuolar Na+/H+ antiporter gene of Arabidopsis in buckwheat, the rutin concentrations of leaves, stems and roots in both transgenic line and wild-type plant were measured. The results were showed in Table 3. The rutin concentrations of leaves, stems and roots in transgenic line were 6.4, 2.4 and 4.3-fold of the wild-type plant respectively. Thus, it suggested that the synthesis of rutin was enhanced in transgenic plants overexpressing AtNHX1.
Discussion
The improvement of plant salt-tolerance by the overexpression of a vacuolar Na+/H+ antiporter gene AtNHX1 from Arabidopsis has been carried out in dicot species, such as A. thaliana, tomato and B. napus, cotton and others (Yamaguchi and Blumwald 2005). The effects of preculture, addition of AS, infection, and extent of co-culture time were evaluated by the transformation frequency of explants infected with A. tumefaciens strain LBA4404 harboring the plasmid pHZX1. The results showed that AtNHX1-positive transgenic plants could be regenerated effectively from hypocotyl co-cultured for 1∼2 days with Agrobacterium after preculture for 2 days and infection for 20∼25 min. However, another factor affecting transformation efficiency, AS, did not increase the transformation frequency.
The salt tolerance trait in natural wheat genotypes and many other monocot species is often closely associated with a low level of Na+ accumulation in leaves and a high ratio of leaf K+/Na+ content (Schachtman et al. 1992; Gorham 1990; Gorham et al. 1990). This is contrary with dicot species, where there is no apparent correlation between salt tolerance and the leaf Na+ content (Tester et al. 2003). In this report, we monitored the Na+ content of buckwheat treated with NaCl (0–200 mmol/l) and found that the Na+ content in leaves and roots of the transgenic line were higher than that of wild-type plant (Fig. 5). And Na+ mainly accumulated in leaves in comparison with roots, and the transgenic plants showed enhanced salt-tolerance compared with the wild-type. Similar results have also been obtained in Arabidopsis thaliana, tomato, Brassica and other plants overexpressing vacuolar Na+/H+ antiporter gene AtNHX1 (Yamaguchi and Blumwald 2005). Ohta et al. (2002) and Fukuda et al. (2004) found that the salt-tolerance of transgenic rice overexpressing halophyte (A. gmelini) gene AgNHX1 and rice gene OsNHX1 was improved in comparison with wild-type plant under NaCl stress. However, the Na+ content of leaves increased both in the transgenic and wild-type plants similarly. These results confirmed that the Na+/H+ antiporter gene could partially sequester Na+ into the vacuoles and avert the toxic effects of excessive Na+ on the cells.
A markedly lower level of K+ accumulation and K+/Na+ ratio in the leaves of the transgenic lines under severe saline conditions (200 mmol/l NaCl) was also observed, compared with the non-transgenic control. This is likely to be the result of increased Na+ import, because the rate of K+ transport can be affected by Na+ levels through its competition for K+ binding sites of transport proteins which function in acquisition of K+ (Blumwald et al. 2000). For example, wheat transport proteins, HKT1 and LCT1, capable of transporting K+, can also mediate the uptake Na+ (Schachtman et al. 1997; Schachtman et al. 1994) and the competitive interactions of K+ and Na+ for binding to HKT1 has been shown by Gassmann et al. (1996).
When grown under the same conditons, rutin concentrations of transgenic buckwheat line were higher than that of wild-type plant, which indicated that overexpression of Na+/H+ antiporter gene could influence rutin metabolism and its possible accumulation in the vacuole. Vacuolar Na+/H+ antiporters have been shown to have significant effects in intravesicular trafficking and protein targeting (Sottosanto et al. 2004), and it is possible that the increase in rutin content was due to the altered homeostasis brought about by the expression of AtNHX1. Therefore, when AtNHX1 gene was introduced into buckwheat by Agrobacterium, the medical value of buckwheat would not be affected.
Genetic transformation of many higher plants has been achieved using several methods, such as silicon carbide fiber-mediated transformation (Dalton et al. 1998), direct gene transfer to protoplast (Wang et al. 1997), biolistic transformation and others (Cho et al. 2000; Altpeter et al. 2000; Dalton et al. 1999). However, buckwheat transformation was not achieved unitl now. In this study, the salt tolerant gene AtNHX1 was introduced into buckwheat with Agrobacterum-mediated method, demonstrating the suitability of buckwheat for genetic transformation. The salt tolerance of the resultant transgenic buckwheat has been improved, suggesting that these plants could be important for agricultural production in saline soils or could be irrigated with non-potable saline water.
References
Abeywardena MY, Head RJ (2001) Dietary polyunsaturated fatty acid and antioxidant modulation of vascular dysfunction in the spontaneously hypertensive rat. Prostaglandins Leukot Essent Fatty Acids 65:91–97
Altpeter F, Xu JP (2000) Rapid production of transgenic turfgrass (Festuca rubra L.) plants. J Plant Physiol 157:441–448
Apse MP, Aharon GS, Snedden WA, Blumwald E (1999) Salt tolerance conferred by overexpression of a vacuolar Na+/H+ antiporter in Arabidopsis. Science 285:1256–1258
Bates LS, Waldren RP, Teare ID (1973) Rapid determination of proline for water-stress studies. Plant Soil 39:205–207
Blumwald E, Aharon GS, Apse MP (2000) Sodium transport in plant cells. Biochim Biophys Acta 1465:140–151
Bonafaccia G, Gambelli L, Fabjan N, Kreft I (2003) Trace elements in flour and bran from common and tartary buckwheat. Food Chem 83:1–5
Bovy A, Vos RD, Kempera M, Schijlena E, Pertejoa MA, Muirb S, Collinsb G, Robinsonb S, Verhoeyenb M, Hughesc S, Buelgad CS, Tunena A (2002) High-flavonol tomatoes resulting from the heterologous expression of the maize transcription factor genes Lc and C1. Plant Cell 14:2509–2526
Cho MJ, Ha CD, Lemaux PG (2000) Production of transgenic tall fescue and red fescue plants by particle bombardment of mature seed-derived highly regenerative tissues. Plant Cell Rep 19:1184–1189
Chen LH, Xu ZQ (2006) Tissue culture and high-frequency plant regeneration of buckwheat (Fagopyrum esculentum Moench). J Mol Cell Biol 39(5):445–452
Dalton SJ, Bettany AJE, Morris P (1999) Co-transformed, diploid Lolium perenne (perennial ryegrass), Lolium multiflorum (Italian ryegrass) plants produced by microprojectile bombardment. Plant Cell Rep 18:721–726
Dalton SJ, Bettany AJE, Timms E, Morris P (1998) Transgenic plants of Lolium multiflorum, Lolium perenne, Festuca arundinacea and Agrostis stolonifera by silicon carbide fibre-mediated transformation of cell suspension cultures. Plant Sci 312:31–43
Davis WL, Matthew SB (2000) Antioxidants and cancer III: quercetin. Altern Med Rev 5:196–208
Fukuda A, Nakamura A, Tagiri A, Tanaka H, Miyao A, Hirochika H, Tanaka Y (2004) Function, intracellular localization and the importance in salt tolerance of a vacuolar Na+/H+ antiporter from rice. Plant Cell Physiol 45(2):146–159
Gassmann W, Rubio F, Schroeder JI (1996) Alkali cation selectivity of the wheat root high-affinity potassium transporter HKT1. Plant J 10:869–882
Gaxiola RA, Rao R, Sherman A, Grisafi P, Alper SL, Fink GR (1999) The Arabidopsis thaliana proton transporters, AtNhx1 and Avp1, can function in cation detoxification in yeast. Proc Natl Acad Sci USA 96:1480–1485
Gorham J (1990) Salt tolerance in the Triticeae: K+/Na+ discrimination in synthetic hexaploid wheat. J Exp Bot 41:623–627
Gorham J, Wyn Jones RGA (1990) Bristol, partial characterization of the trait for enhanced K+/Na+ discrimination in the D genome of wheat. Planta 180:590–597
Greenway H, Munns R (1980) Mechanisms of salt tolerance in nonhalophytes. Annu Rev Plant Physiology 31:149–190
Holasova M, Fidlerova V, Smrcinova H, Orsak M, Lachman J, Vavreinova S (2001) Buckwheat––the source of antioxidant activity in functional foods. Food Res Intern 35:207–211
Im J, Huff HE, Hsieh F (2003) Effects of processing conditions on the physical and chemical properties of buckwheat grit cakes. J Agric Food Chem 51:659–666
Jin H, Hao JG, Jia JF (2002) Efficient plant regeneration in vitro in buckwheat. Plant Cell Tissue Organ Cult 69:293–295
Kim KH, Lee YH, Kim D, Park YH, Lee JY, Wang YS, Kim YH (2004) Agrobacterium-mediated genetic transformation of Perilla frutescens. Plant Cell Rep 23:386–390
Kim SL, Kim SK, Park CH (2004) Introduction and nutritional evaluation of buckwheat sprouts as a new vegetable. Food Res Intern 37:319–327
Kreft S, Knapp M, Kreft I (1999) Extraction of rutin from buckwheat (Fagopyrum esculentum Moench) seeds and determination by capillary electrophoresis. J Agric Food Chem 47:4649–4652
Kreft I, Fabjan N, Yasumoto K (2006) Rutin content in buckwheat (Fagopyrum esculentum Moench) food materials and products. Food Chem 98:508–512
KrkosKova B, Mrazova Z (2005) Prophylactic components of buckwheat. Food Res Intern 38:561–568
Li YY, Wang ZH, Zhang Z (2004) An optimum method of extracting buckwheat genomic RNA. Biotechnology 14:23–24 (in Chinese)
Mimura T, Kura-Hotta M, Tsujimura T, Ohnishi M, Miura M, Okazaki Y, Mimura M, Maeshima M, Washitani-Nemoto S (2003) Rapid increase of vacuolar volume in response to salt stress. Planta 216:397–402
Ohta M, Hayashi Y, Nakashima A, Hamada A, Tanaka A, Nakamura T, Hayakawa T (2002) Introduction of a Na+/H+ antiporter gene from Atriplex gmelini confers salt tolerance to rice. FEBS Lett 532:279–282
Park JW, Kang DB, Kim CW, Ko SH, Yum HY, Kim KE (2000) Identification and characterization of the major allergens of buckwheat. Allergy 55:1035–1041
Ratner A, Jacoby B (1976) Effect of K+, its counter anion, and pH on sodium efflux from barley root tips. J Exp Bot 27:843–852
Sambrook J, Fritsch EF, Maniatis T (1989) Molecular cloning, a lab manual, 2 edn. Cold Spring Harbour Laboratory Press, NY, pp 31–58
Schachtman DP, Kumar R, Schroeder JI, Marsh EL (1997) Molecular and functional characterization of a novel low-affinity cation transporter (LCT1) in higher plants. Proc Natl Acad Sci USA 94:11079–11084
Schachtman DP, Lagudah ES, Munns R (1992) The expression of salt tolerance from Triticum tauschii in hexaploid wheat. Theor Appl Genet 84:714–719
Schachtman DP, Munns R (1992) Sodium accumulation in leaves of Triticum species that differ in salt tolerance. Aust J Plant Physiol 19:331–340
Schachtman DP, Schroeder JI (1994) Structure and transport mechanism of a high-affinity potassium uptake transporter from higher plants. Nature 370:655–658
Shannon MC (1997) Adaptation of plants to salinity. Adv Agron 60:75–120
Shi HZ, Ishitani M, Kim CS, Zhu JK (2000) The Arabidopsis thaliana salt tolerance gene SOS1 encodes a putative Na+/H+ antiporter. Proc Natl Acad Sci USA 97:6896–6901
Sottosanto JB, Gelli A, Blumwald E (2004) DNA array analysis of Arabidopsis thaliana lacking a vacuolar Na+/H+ antiporter: impact of AtNHX1 on gene expression. Plant J 40:752–771
Suzuki T, Honda Y, Funatsuki W, Nakatsuka K (2002) Purification and characterization of flavonol 3-glucosidase, and its activity during the ripening in tartary buckwheat seeds. Plant Sci 163:417–423
Tao X, Apse MP, Aharon GS, Blumwald E (2002) Identification and characterization of a NaCl-inducible vacuolar Na+/H+ antiporter in Beta vulgaris. Physiol Plant 116:206–212
Tester M, Davenport R (2003) Na+ tolerance and Na+ transport in higher plants. Ann Bot 91:503–527
Wang GR, Binding H, Posselt UK (1997) Fertile transgenic plants from direct gene transfer to protoplasts of Lolium perenne L. and Lolium multiflorum Lam. J Plant Physiol 151:83–90
Wang YY, Chen QJ, Chen M, Chen J, Wang XC (2005) Salt-tolerant transgenic perennial ryegrass (Lolium perenne L.) obtained by Agrobacterium tumefaciens-mediated transformation of the vacuolar Na+/H+ antiporter gene. Plant Sci 169:65–73
Watanabe M (1998) Catechins as antioxidants from buckwheat (Fagopyrum esculentum Moench) groats. J Agric Food Chem 46:839–845
Wojcicki J, Barcew-Wiszniewska B, Samochowiec L, Rozewicka L (1995) Extractum Fagopyri reduces atherosclerosis in high-fat diet fed rabbits. Die Pharmazie 50:560–562
Xue GP (2002) Characterization of the DNA-binding profile of barley HvCBF1 using an enzymatic method for rapid, quantitative and high-throughput analysis of the DNA-binding activity. Nucl Acids Res 30:77–79
Yamaguchi T, Blumwald E (2005) Developing salt tolerant crop plants: challenges and opportunities. Trend Plant Sci 12:615–620
Zhang HX, Blumwald E (2001) Transgenic salt-tolerant tomato plants accumulate salt in foliage but not in fruit. Nat Biotechnol 19:765–768
Zhang HX, Hodson JN, Williams JP, Blumwald E (2001) Engineering salt-tolerant Brassica plants: characterization of yield and seed oil quality in transgenic plants with increased vacuolar sodium accumulation. Proc Natl Acad Sci USA 98:12832–12836
Zhe YX, Da YZ, Gang PX, Zhang H, Zhao YX, Xia GM (2004) Enhanced salt tolerance of transgenic wheat (Triticum aestivum L.) expressing a vacuolar Na+/H+ antiporter gene with improved grain yields in saline soils in the field and a reduced level of leaf Na+. Plant Sci 167:849–859
Acknowledgement
The authors thank Dr. Hong-Xia Zhang who provided us the plasmid pHZX1. This work was supported by Major Research Project of Natural Science Foundation of Shaanxi Province (2001 SM24), Research Project of Provincial Key Laboratory of Shaanxi (04JS07) and Scientific Research Project of the Education Department of Shaanxi Province (05JK304).
Author information
Authors and Affiliations
Corresponding author
Rights and permissions
About this article
Cite this article
Chen, LH., Zhang, B. & Xu, ZQ. Salt tolerance conferred by overexpression of Arabidopsis vacuolar Na+/H+ antiporter gene AtNHX1 in common buckwheat (Fagopyrum esculentum). Transgenic Res 17, 121–132 (2008). https://doi.org/10.1007/s11248-007-9085-z
Received:
Accepted:
Published:
Issue Date:
DOI: https://doi.org/10.1007/s11248-007-9085-z