Abstract
Wheat–tan spot interactions are known to have an inverse gene-for-gene relationship where pathogen-produced necrotrophic effectors are recognized by host sensitivity genes to cause susceptibility. However, broad-spectrum race-nonspecific resistance quantitative trait loci (QTL) that do not conform to the inverse gene-for-gene model have also been identified in this system. Here, we evaluated a population of wheat recombinant inbred lines derived from Salamouni (resistant) and Katepwa (susceptible) for reaction to two isolates of race 1 (Pti2 and Asc1) and one isolate of race 2 (86–124), which all produce the necrotrophic effector Ptr ToxA, and the isolate AR LonB2, which does not produce Ptr ToxA and does not conform to the current race classification system. As expected, the Tsn1 locus was significantly associated with disease caused by all three ToxA-producing isolates and was not associated with tan spot caused by AR LonB2. However, the amount of variation explained by Tsn1 varied considerably, with values of 5, 22, and 30 % for Asc1, Pti2, and 86–124, respectively, suggesting possible variability in ToxA gene regulation among these isolates. A locus on chromosome arm 7DS was specifically associated with isolate AR LonB2 but explained only 8 % of the variation. Additional QTL on 5DL and 7BS were race-nonspecific and associated with tan spot caused by multiple isolates. These results provide further evidence that race-nonspecific resistance QTL play important roles in governing reaction to tan spot, and they suggest that the wheat–tan spot pathosystem is more complicated than previously thought. The elimination of necrotrophic effector sensitivity genes and the addition of race-nonspecific resistance loci are needed to develop wheat cultivars with high levels of tan spot resistance.
Similar content being viewed by others
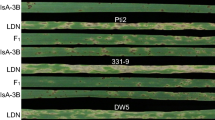
Avoid common mistakes on your manuscript.
Introduction
Tan spot is a foliar disease of wheat caused by the necrotrophic fungus Pyrenophora tritici-repentis (Died.) Drechs. [anamorph Drechslera tritici-repentis (Died.) Shoem.]. The pathogen infects both durum (Triticum turgidum) and bread wheat (T. aestivum) and occurs nearly everywhere these crops are grown. Conservation tillage practices have led to an increased incidence of tan spot due to the ability of the fungus to overwinter in stubble residue (Rees 1982). When conditions are favorable, tan spot has the potential to cause yield losses approaching 50 % (Hosford 1982; Rees and Platz 1983; Mehta and Gaudencio 1991). Typical symptoms of susceptible reactions include large, sometimes coalescing necrotic lesions surrounded by moderate to extensive chlorosis, whereas resistant reactions are limited to circular lesions usually dark in color that remain very small.
Isolates of P. tritici-repentis are currently classified into eight races based on their ability to produce one or more of three characterized necrotrophic effectors (host-selective toxins) (Lamari et al. 2003). The P. tritici-repentis effectors identified to date consist of Ptr ToxA, which causes necrosis, and Ptr ToxB and Ptr ToxC, which both induce chlorosis (Ciuffetti et al. 2010 for review). Race 1 isolates produce Ptr ToxA and Ptr ToxC, race 2 isolates produce Ptr ToxA, race 3 isolates produce Ptr ToxC, race 4 isolates are avirulent, and race 5 isolates produce Ptr ToxB. Races 6, 7, and 8 combine the effectors of races 3 and 5, 2 and 5, and 2, 3, and 5, respectively (Lamari et al. 2003). A set of four hexaploid wheat lines including Glenlea (necrosis caused by race 2), 6B365 (chlorosis caused by race 3), 6B662 (chlorosis caused by race 5), and Salamouni (resistant to all races) has been used to differentiate the races (Lamari et al. 1998).
Single genes control host sensitivity to each of the three effectors. The Tsn1 gene on the long arm of wheat chromosome 5B confers sensitivity to Ptr ToxA (Faris et al. 1996; Anderson et al. 1999). Extensive genomic analysis of Tsn1 (Haen et al. 2004; Lu and Faris 2006; Lu et al. 2006) led to the isolation of the gene, which was found to have similarity to the protein kinase and nucleotide binding site–leucine-rich repeat (NBS-LRR) classes of plant resistance genes (Faris et al. 2010). Sensitivity to Ptr ToxC and Ptr ToxB is governed by the Tsc1 gene on chromosome arm 1AS (Effertz et al. 2002) and the Tsc2 gene on chromosome arm 2BS (Friesen and Faris 2004; Abeysekara et al. 2010), respectively, neither of which has been cloned.
Although single genes have been shown to govern sensitivity to each of the three effectors, and all three effector–host gene interactions have been shown to play significant roles in disease development (Effertz et al. 2002; Cheong et al. 2004; Friesen and Faris 2004; Chu et al. 2008; Singh et al. 2008a, b, 2010; Sun et al. 2010), insensitivity to the effectors alone will not necessarily lead to complete disease resistance because, in many cases, other genetic factors in addition to the effector sensitivities are involved depending on the host genetic background (Faris et al. 1997, 1999; Friesen et al. 2003; Chu et al. 2008; Singh et al. 2008a, b; Li et al. 2011). In addition, more recent studies have led to the identification of single major genes on chromosomes 3A and 3D governing reaction to tan spot caused by race 1 isolates (Singh et al. 2006, 2008a, b; Tadesse et al. 2006b, 2008), and on chromosome 3B controlling reaction to races 3 and 5 (Tadesse et al. 2006a, 2010). Although effectors associated with the host genes on group 3 chromosomes have not yet been identified, in each case resistance was reported to be recessive, suggesting that host gene–effector interactions are likely responsible for governing susceptibility in these cases as well.
Other recent studies have reported the identification of race-nonspecific resistance quantitative trait loci (QTL) on several different chromosomes in populations where known effector sensitivity genes segregated but had no significant association with tan spot reaction (Faris and Friesen 2005; Chu et al. 2008, 2010). Together, these results strongly suggest the P. tritici-repentis race classification system is not sufficient to account for the complexity of the P. tritici-repentis–wheat interaction.
The objectives of this research were to analyze a population of recombinant inbred lines (RILs) derived from the wheat lines Salamouni and Katepwa for QTL associated with tan spot resistance caused by two different race 1 isolates, a race 2 isolate, and an isolate collected from Arkansas that does not fit the current race classification system because it caused necrosis on the Ptr ToxA differential cultivar Glenlea, but did not produce Ptr ToxA (Ali et al. 2010).
Materials and methods
Plant materials
A population derived from the hexaploid wheat landrace Salamouni and the Canadian bread wheat variety Katepwa, consisting of 121 RILs, was evaluated for reaction to tan spot. The population will hereafter be referred to as the SK population. Details regarding the development of the SK population were provided in Abeysekara et al. (2010).
Conidial inoculations and disease evaluation
The SK population and parents were screened with conidia produced by the race 1 isolates Asc1 and Pti-2, the race 2 isolate 86–124, and an isolate collected from Arkansas, USA, and designated AR LonB2 (Ali et al. 2010). Isolate AR LonB2 was shown by Ali et al. (2010) not to fit the current race classification system because it lacked the ToxA gene but still induced necrosis on the ToxA differential line Glenlea. Isolates were grown on plates containing V8-potato dextrose agar (Difco PDA; Becton, Dickinson and Company, Sparks, MD, USA) in the dark for 5–7 days. Inoculum was prepared as described by Lamari and Bernier (1989) and Ali et al. (2010). For each isolate, the parents and 121 SK RILs were planted in a completely randomized design consisting of three replications. Each replication consisted of one cone (Stuewe and Sons, Inc., Corvallis, OR, USA) per RIL and three plants per cone. Therefore, each experimental unit consisted of three plants, and a total of nine plants per line were evaluated for each isolate. Plants were grown in racks of 98 with the outside border of each rack planted with the susceptible variety Grandin to reduce any potential edge effects. Inoculum was adjusted to a concentration of 3,000 spores/ml and sprayed onto the plants at the 2- to 3-leaf stage until runoff as described in Abeysekara et al. (2010). After inoculation, plants were placed in a mist chamber with 100 % relative humidity at 21 °C for 24 h and then moved to growth chambers at the same temperature under a 12-h photoperiod for 6 days. Plants were then rated using a 1–5 lesion-type scale (Lamari and Bernier 1989) where 1 is the most resistant and 5 is the most susceptible.
To determine if RILs were of the Tsn1Tsn1 or tsn1tsn1 genotype, they were infiltrated with Ptr ToxA and scored as sensitive or insensitive based on the development of necrosis (Abeysekara et al. 2010). By definition, Ptr ToxA-sensitive RILs are Tsn1Tsn1 genotype and Ptr ToxA-insensitive RILs are tsn1tsn1 genotype.
Statistical and QTL analysis
Error variances among replicates for each isolate were evaluated for homogeneity using Bartlett’s Chi-squared test conducted with the PROC GLM command in SAS (SAS Institute 2008). Data from the replicates of each isolate that lacked significant heterogeneity for error variances were used to calculate reaction type means which were then used for QTL analysis. Fisher’s protected least significant difference (LSD) was calculated to identify significant differences among reaction-type means for each isolate at the 0.05 level of probability.
Linkage analysis and map construction for all 21 chromosomes in the SK population was described in Abeysekara et al. (2012). Here, a subset of 323 markers, used for map construction and giving the most complete coverage and spanning all 21 chromosomes, was used. The marker set consisted of 319 DNA markers and four morphological markers (Abeysekara et al. 2012) including Tsn1, Tsc2, Snn3 and Snn4, which confer sensitivity to the necrotrophic effector ToxA (produced by both P. tritici-repentis and the necrotrophic pathogen Stagonospora nodorum), Ptr ToxB (produced by P. tritici-repentis), and the S. nodorum effectors SnTox3 and SnTox4, respectively. The phenotypic data for each of the three replicates of each P. tritici-repentis was averaged for each of the RILs, and the mean lesion-type data was used for QTL analysis. The software programs QGene (Joehanes and Nelson 2008) and MapManager QTX (Manly et al. 2001) were used to conduct composite interval mapping (CIM) to identify markers and marker intervals significantly associated with tan spot resistance. A critical LOD threshold of about 3.15 was determined based on 1,000 permutations for each trait. Single factor regression was performed using markers associated with QTL based on CIM analysis to identify the markers most significantly (P < 0.01) associated with the QTL, which were then assembled into multiple regression models to determine the total amount of variation explained by the markers using the software program DataDesk (Data Description Inc., v4.1).
Results
Reaction of the SK population to Pyrenophora tritici-repentis inoculations
Salamouni was resistant to all four isolates with average disease reactions ranging from 1.25 for Asc1 to 1.83 for AR LonB2, whereas Katepwa was moderately susceptible to all four isolates with average reactions ranging from 3.13 for Asc1 to 3.67 for 86–124 (Table 1; Fig. 1). Average disease reaction types for the SK population were the least for isolate Asc1, with an average of 2.09 compared to isolates Pti-2, 86–124, and AR LonB2, which gave similar reaction-type averages of 2.64, 2.63, and 2.56, respectively. Some transgressive segregation was observed in the population with reaction types ranging from 1.0 to 4.17 for the four isolates overall (Table 1; Fig. 1).
Histograms of average tan spot reaction types in the Salamouni × Katepwa recombinant inbred population inoculated with four different isolates of Pyrenophora tritici-repentis. For isolates that produce Ptr ToxA (Asc1, Pti2, and 86–124), ToxA-sensitive and ToxA-insensitive lines are indicated by yellow and black bars, respectively. The blue bars used for the histogram of AR LonB2 represent the whole population
Ptr ToxA is produced by race 1 and race 2 isolates, but not by the AR LonB2 isolate. Further analysis of average lesion types indicated that ToxA-sensitive RILs had average reaction types of 2.21, 2.95, and 3.02 for isolates Asc1, Pti-2, and 86–124, respectively, whereas ToxA-insensitive genotypes had average reaction types of 1.99, 2.36, and 2.28 for the three isolates (Table 1; Fig. 1). In each of these cases, the ToxA average reaction types among the ToxA sensitive lines were significantly higher than among the ToxA insensitive lines, indicating that a compatible Tsn1–ToxA interaction contributed significantly to the development of tan spot caused by the three ToxA-producing isolates. However, the average disease reaction types for ToxA-sensitive and ToxA-insensitive RILs in response to AR LonB2 also did not differ, which was as expected due to the fact that AR LonB2 does not possess the ToxA gene (Ali et al. 2010).
Identification of QTL associated with tan spot resistance in the SK population
A total of four QTL associated with resistance to the four isolates were identified by CIM in the SK population, and resistance effects at all four loci were contributed by the resistant parent Salamouni. The four QTL were located on chromosome arms 5BL, 5DL, 7BS, and 7DS, and designated QTs.fcu-5B, QTs.fcu-5D, QTs.fcu-7B, and QTs.fcu-7D, respectively.
Not all QTL were significantly associated with resistance to all four isolates. QTs.fcu-5B and QTs.fcu-7B were significantly associated with resistance to Pti-2 and explained 25 and 8 % of the variation, respectively (Table 2; Fig. 2). The effects of QTs.fcu-5B were due to the Tsn1–ToxA interaction indicated by the defined peak of QTs.fcu-5B at the Tsn1 locus (Fig. 2). The marker Xbarc126.1 was the most significant marker associated with QTs.fcu-7B. In single factor regression, Tsn1 and Xbarc126.1 explained 22 and 7 % of the variation, respectively, and together they explained a total of 31 % (Table 3).
Composite interval regression maps of QTL associated with tan spot caused by isolates Asc1 (race 1), Pti2 (race1), 86–124 (race 2), and AR LonB2. In each case, resistance effects were contributed by Salamouni. The markers and their positions are indicated along the right side of the illustrated linkage maps and distances in centiMorgans (cM) are shown along the left. Composite interval regression lines are aligned with their corresponding linkage groups. The critical LOD threshold is indicated by the dotted lines. The line colors for each isolate are indicated in the legend
For the other race 1 isolate, Asc1, only QTs.fcu-5D was significant in CIM explaining 13 % of the variation (Table 2; Fig. 2). Although Asc1 is race 1 and known to produce Ptr ToxA, QTs.fcu-5B was not significant in CIM (Fig. 2). However, the Tsn1 locus was significant in single factor regression and explained 5 % of the variation (Table 3). Xwmc215 was the most significant marker associated with QTs.fcu-5D, and it explained 13 % of the variation in single factor regression. Together, Tsn1 and Xwmc215 explained 19 % of the variation for resistance to Asc1. QTs.fcu-7B, which was significantly associated with the other race 1 isolate Pti-2, was not significantly associated with resistance to Asc1 (Fig. 2).
The QTL QTs.fcu-5B and QTs.fcu-7B, which were associated with resistance to the race 1 isolate Pti-2, were also significantly associated with resistance to the race 2 isolate 86–124 in CIM explaining 32 and 5 % of the variation, respectively (Table 2; Fig. 2). However, the chromosome 7B markers including Xbarc126.1 were not significant in single factor regression, and the QTs.fcu-5D marker Xwmc215 was significant, explaining 5 % of the variation (Table 3). Together, Tsn1 and Xwmc215 explained 40 % of the variation for resistance to 86–124 in the multiple regression model.
For the isolate AR LonB2, which does not produce Ptr ToxA and does not belong to the current race classifications, the QTL QTs.fcu-7B and QTs.fcu-7D were significantly associated in CIM and explained 6 and 7 % of the variation, respectively (Table 2; Fig. 2). Xgwm44.2 was the most significant marker associated with QTs.fcu-7D and explained 8 % of the variation in single factor regression (Table 3). Markers associated with QTs.fcu-7B were not significant in single factor regression, but Xwmc215, which was associated with QTs.fcu-5D, was significant and explained 11 % of the variation. Together, Xgwm44.2 and Xwmc215 explained 18 % of the variation for resistance to AR LonB2 (Table 3).
Discussion
The hexaploid landrace Salamouni has been considered a durable tan spot resistance source and used in the differential set as the line that is universally resistant to all races (Lamari et al. 1998). Katepwa was known to be susceptible to race 1- and race 2-induced necrosis and chlorosis induced by race 5. Abeysekara et al. (2010) showed that in this population susceptibility to chlorosis caused by race 5 was due to the Tsc2–Ptr ToxB interaction, which accounted for 54 % of the variation in disease caused by the race 5 isolate DW5. The current research was conducted to evaluate the role of the Tsn1–Ptr ToxA interaction in the SK population and to identify additional factors (QTL) contributing to resistance or susceptibility caused by races 1 and 2, and the recently described isolate from Arkansas (Ali et al. 2010).
Of the four QTL identified, QTs.fcu-5B associated with Pti-2 and 86–124 had by far the largest effects (Fig. 2), which were due to the Tsn1–Ptr ToxA interaction. Therefore, QTs.fcu-5B is considered a susceptibility QTL due to Ptr ToxA sensitivity contributed by Katepwa. QTs.fcu-5B was not significant for isolate Asc1 in CIM. As a race 1 isolate, Asc1 is known to produce Ptr ToxA, and therefore one would expect the Tsn1 locus to be significantly associated with reaction to Asc1. However, the Tsn1 locus was significant in single factor regression, and average reaction types between the Tsn1Tsn1 and tsn1tsn1 genotypic classes were significantly different. In addition, the CIM regression curve for chromosome 5B generated using the Asc1 data also strongly suggested that the Tsn1–Ptr ToxA interaction played a role in governing disease caused by Asc1. Past research has shown that, depending on the host genetic background, Tsn1 can play a highly significant role (Cheong et al. 2004; Singh et al. 2008a, b, 2010), a relatively minor role (Friesen et al. 2003; Chu et al. 2008), or have no significant effects (Faris and Friesen 2005; Chu et al. 2010). Friesen et al. (2006) evaluated Ptr ToxA haplotypes of 54 P. tritici-repentis isolates, including Pti-2 and Asc1, and found no sequence variation in the ToxA gene. Therefore, allelic variation in Ptr ToxA is not likely responsible for the difference in Tsn1–Ptr ToxA effects between Pti-2 and Asc1. It is possible that other host resistance mechanisms, particularly broad spectrum or race-nonspecific resistance mechanisms, may preclude host–effector interactions such as Tsn1–Ptr ToxA, as suggested by Faris and Friesen (2005), or that the effects of a given host–effector interaction are reduced or masked due to epistatic effects with other host–effector interactions (Friesen et al. 2008). It is also possible that different isolates may express the same effectors at different levels, which was shown by Faris et al. (2011) to be the case with two S. nodorum ToxA-producing isolates. Faris et al. (2011) showed that the isolate that expressed ToxA at higher levels caused more Tsn1-specific disease than the isolate with lower ToxA expression. This could be the case with Asc1 and Pti-2.
In CIM analysis, QTs.fcu-5D was significantly associated with tan spot caused by Asc1 only, and QTs.fcu-7B was significant for Pti-2, 86–124, and AR LonB2, but not significant for Asc1. However, the regression curves generated in CIM analysis suggest that both QTL may be associated with response to all four isolates tested (Fig. 2), and therefore suggest that QTs.fcu-5D and QTs.fcu-7B may be race-nonspecific resistance QTL. QTs.fcu-7D was specific to AR LonB2 and only a slight indication of an association between this QTL and response to 86–124 was indicated by the chromosome 7D regression curves, whereas clearly no association between the 7D locus and either race 1 isolate existed.
No major tan spot resistance genes or effector sensitivity loci have been reported on chromosomes 5D, 7B, or 7D. Chu et al. (2010) reported a QTL with minor effects for resistance to Pti-2 and 86–124 in tetraploid wheat on chromosome 7B, but that QTL was on the long arm of 7B, whereas QTs.fcu-7B reported in this work was on the short arm. Therefore, these likely represent different QTL on chromosome 7B. Faris and Friesen (2005) reported a tan spot resistance QTL with minor effects for resistance to a race 3 isolate on chromosome arm 7DS. The marker most closely associated with that QTL was Xbarc126, the same marker associated with QTs.fcu-7D in this research, which is a strong indication that the same QTL, or alleles of the same QTL, were identified. This would suggest that, like QTs.fcu-5D and QTs.fcu-7B, QTs.fcu-7D is not race-specific.
By definition, P. tritici-repentis race 1 isolates produce effectors Ptr ToxA and Ptr ToxC, which are recognized by the sensitivity genes Tsn1 and Tsc1 on chromosome arms 5BL and 1AS and cause necrosis and chlorosis, respectively (Lamari et al. 1989), 2003; Faris et al. 1996; Ciuffetti and Tuori 1999; Effertz et al. 2002). As discussed above, the Tsn1 locus was associated with governing reaction to both isolates, but no QTL or marker on 1AS was found to be associated with either isolate indicating that sensitivity to Ptr ToxC does not segregate in this population, which agrees with previous research indicating that both Salamouni and Katepwa are insensitive to Ptr ToxC (Lamari et al. 1995). QTs.fcu-5D and QTs.fcu-7B appeared to have minor effects for both isolates, and multiple regression models for Pti-2 and Asc1 explained 31 and 19 % of the variation, respectively. While environmental and experimental inconsistencies may account for some of the variation, it is likely that other genetic factors went undetected, possibly due to the small population size or inadequate coverage of the genome with markers (Abeysekara et al. 2012). Therefore, it is possible that Pti-2 and/or Asc1 may harbor unique virulence factors.
Zhang et al. (2011) showed that two homeologous genes on the short arms of chromosomes 5B and 5D conferred sensitivity to the same necrotrophic effector produced by S. nodorum. Observation of the locations of the QTL identified in this research could suggest that the QTL on the long arms of 5B and 5D are homoeologous. However, through the cloning and characterization of the Tsn1 gene, Faris et al. (2010) showed that Tsn1 was specific to chromosome 5B, and that homoeoalleles do not exist on the homoeologous chromosomes 5A and 5D. Therefore, the gene responsible for the effects of QTs.fcu-5D is in a similar position relative to Tsn1, but most likely is not homoeologous with Tsn1.
In contrast, the microsatellite marker Xbarc126 detected loci associated with both of the QTL on the short arms of chromosomes 7B and 7D. Assuming that Xbarc126 detects homoeologous loci on these chromosomes, this would suggest that homoeologous genes possibly govern the effects of QTs.fcu-7B and QTs.fcu-7D.
In this research, we used the universal tan spot resistant line Salamouni as one parent of the SK mapping population. Tadesse et al. (2006b) also investigated the genetic basis of tan spot resistance conferred by Salamouni. Inoculating with a race 1 isolate (Asc1a), they conducted monosomic analysis using the monosomic lines of the hexaploid landrace Chinese Spring, which was susceptible to Asc1a. Their analysis revealed that chromosome 3A was the critical chromosome, and therefore the authors claimed that Salamouni harbored a recessive resistance gene on chromosome 3A that conferred resistance to tan spot caused by Asc1a. The results of our research gave no indication of a resistance gene/QTL on Salamouni 3A for any of the isolates, including the race 1 isolates Pti-2 and Asc1. There are two possible explanations for this. First, it is possible that Katepwa has the same recessive resistance gene on 3A as Salamouni, and therefore the population does not segregate for it. However, if this were the case, Katepwa would likely have higher levels of tan spot resistance than those observed. Alternatively, it is possible that Salamouni does not harbor a recessive resistance gene per se, but rather lacks a dominant gene for susceptibility, which is present in Chinese Spring. This is the most likely scenario given the known structure of the wheat–tan spot system, which is known to harbor several host–toxin interactions (Wolpert et al. 2002). This scenario would explain why we found no QTL associated with resistance derived from Salamouni on chromosome 3A, and it would suggest that the isolate Asc1a used by Tadesse et al. (2006b) produces a necrotrophic effector that is recognized by an effector sensitivity gene present on chromosome 3A of Chinese Spring. Further experimentation and analysis is needed to prove or disprove this hypothesis.
Tan spot has become a significant threat to wheat production worldwide over the past few decades, and as a necrotrophic pathogen, P. tritici-repentis is expected to thrive under a changing global climate (Manning and Tiedemann 1995). Molecular markers associated with resistance QTL can be used to expedite the introgression of resistance loci into adapted germplasm in a most efficient manner. The genomic analysis and cloning of the Tsn1 gene has led to the development of highly effective molecular markers that can be used to select against the Tsn1 allele that confers ToxA sensitivity (Lu et al. 2006; Oliver et al. 2008; Zhang et al. 2009; Faris et al. 2010). The effects of the QTL identified on 5D, 7B, and 7D were relatively minor. Therefore, their effects as well as marker associations should be validated in other genetic backgrounds and larger populations. However, the race-nonspecificity of these QTL makes them good candidates for use in developing wheat cultivars with broad spectrum and durable tan spot resistance.
References
Abeysekara NS, Friesen TL, Liu ZH, McClean PE, Faris JD (2010) Marker development and saturation mapping of the tan spot Ptr ToxB sensitivity locus Tsc2 in hexaploid wheat. Plant Gen 3:179–189
Abeysekara NS, Faris JD, Chao S, McClean PE, Friesen TL (2012) Whole genome QTL analysis of Stagonospora nodorum blotch resistance and validation of the SnTox4-Snn4 interaction in hexaploid wheat. Phytopathology 102:94–104
Ali S, Gurung S, Adhikari TB (2010) Identification and characterization of novel isolates of Pyrenophora tritici-repentis from Arkansas. Plant Dis 94:229–235
Anderson JA, Effertz RJ, Faris JD, Francl LJ, Meinhardt SW, Gill BS (1999) Genetic analysis of sensitivity to a Pyrenophora tritici-repentis necrosis-inducing toxin in durum and common wheat. Phytopathology 89:293–297
Cheong J, Wallwork H, Williams KJ (2004) Identification of a major QTL for yellow leaf spot resistance in the wheat varieties Brookton and Cranbrook. Aust J Agric Res 55:315–319
Chu CG, Friesen TL, Xu SS, Faris JD (2008) Identification of novel tan spot resistance loci beyond the known host-selective toxin insensitivity loci in wheat. Theor Appl Genet 117:873–881
Chu CG, Chao S, Friesen TL, Faris JD, Zhong S, Xu SS (2010) Identification of novel tan spot resistance QTL using an SSR-based linkage map of tetraploid wheat. Mol Breed 25:327–338
Ciuffetti LM, Tuori RP (1999) Advances in the characterization of the Pyrenophora tritici-repentis-wheat interaction. Phytopathology 89:444–449
Ciuffetti LM, Manning VA, Pandelova I, Betts MF, Martinez JP (2010) Host-selective toxins, Ptr ToxA and Ptr ToxB, as necrotrophic effectors in the Pyrenophora tritici-repentis-wheat interaction. New Phytol 187:911–919
Effertz RJ, Meinhardt SW, Anderson JA, Jordahl JG, Francl LJ (2002) Identification of a chlorosis-inducing toxin from Pyrenophora tritici-repentis and the chromosomal location of an insensitivity locus in wheat. Phytopathology 92:527–533
Faris JD, Friesen TL (2005) Identification of quantitative trait loci for race-nonspecific resistance to tan spot in wheat. Theor Appl Genet 111:386–392
Faris JD, Anderson JA, Francl LJ, Jordahl JG (1996) Chromosomal location of a gene conditioning insensitivity in wheat to a necrosis-inducing culture filtrate from Pyrenophora tritici-repentis. Phytopathology 86:459–463
Faris JD, Anderson JA, Francl LJ, Jordahl JG (1997) RFLP mapping of resistance to chlorosis induction by Pyrenophora tritici-repentis in wheat. Theor Appl Genet 94:98–103
Faris JD, Li WL, Liu DJ, Chen PD, Gill BS (1999) Candidate gene analysis of quantitative disease resistance in wheat. Theor Appl Genet 98:219–225
Faris JD, Zhang ZC, Lu HJ, Lu SW, Reddy L, Cloutier S, Fellers JP, Meinhardt SW, Rasmussen JB, Xu SS, Oliver RP, Simons KJ, Friesen TL (2010) A unique wheat disease resistance-like gene governs effector-triggered susceptibility to necrotrophic pathogens. Proc Natl Acad Sci USA 107:13544–13549
Faris JD, Zhang Z, Rasmussen JB, Friesen TL (2011) Variable expression of the Stagonospora nodorum effector SnToxA among isolates is correlated with levels of disease in wheat. Mol Plant Microbe Interact 24:1419–1426
Friesen TL, Faris JD (2004) Molecular mapping of resistance to Pyrenophora tritici-repentis race 5 and sensitivity to Ptr ToxB in wheat. Theor Appl Genet 109:464–471
Friesen TL, Ali S, Kianian S, Francl LJ, Rasmussen JB (2003) Role of host sensitivity to Ptr ToxA in development of tan spot of wheat. Phytopathology 93:397–401
Friesen TL, Stukenbrock EH, Liu ZH, Meinhardt S, Ling H, Faris JD, Rasmussen JB, Solomon PS, McDonald BA, Oliver RP (2006) Emergence of a new disease as a result of interspecific virulence gene transfer. Nat Genet 38:953–956
Friesen TL, Zhang Z, Solomon PS, Oliver RP, Faris JD (2008) Characterization of the interaction of a novel Stagonospora nodorum host-selective toxin with a wheat susceptibility gene. Plant Physiol 146:682–693
Haen KM, Lu HJ, Friesen TL, Faris JD (2004) Genomic targeting and high-resolution mapping of the Tsn1 gene in wheat. Crop Sci 44:951–962
Hosford RM Jr (1982) Tan spot. In: Hosford RM Jr (ed) Tan spot and related diseases workshop. North Dakota State University Agriculture Exp Stn, Fargo, pp 1–24
Joehanes R, Nelson JC (2008) QGene 4.0, an extensible Java QTL-analysis platform. Bioinformatics 24:2788–2789
Lamari L, Bernier CC (1989) Toxin of Pyrenophora tritici-repentis: host-specificity, significance in disease, and inheritance of host reaction. Phytopathology 79:740–744
Lamari L, Sayoud R, Boulif M, Bernier CC (1995) Identification of a new race of Pyrenophora tritici-repentis: Implications for the current pathotype classification system. Can J Plant Pathol 17:312–318
Lamari L, Gilbert J, Tekauz A (1998) Race differentiation in Pyrenophora tritici-repentis and survey of physiologic variation in western Canada. Can J Plant Pathol 20:396–400
Lamari L, Strelkov SE, Yahyaoui A, Orabi J, Smith RB (2003) The identification of two new races of Pyrenophora tritici-repentis from the host center of diversity confirms a one-to-one relationship in tan spot of wheat. Phytopathology 93:391–396
Li HB, Yan W, Liu GR, Wen SM, Liu CJ (2011) Identification and validation of quantitative trait loci conferring tan spot resistance in the bread wheat variety Ernie. Theor Appl Genet 122:395–403
Lu HJ, Faris JD (2006) Macro- and micro-colinearity between the genomic region of wheat chromosome 5B containing the Tsn1 gene and the rice genome. Funct Integr Gen 6:90–103
Lu HJ, Fellers JP, Friesen TL, Meinhardt SW, Faris JD (2006) Genomic analysis and marker development for the Tsn1 locus using bin-mapped ESTs and flanking BAC contigs. Theor Appl Genet 112:1132–1142
Manly KK Jr, Cudmore HH, Meer JM (2001) MAP MANAGER QTX, cross platform software for genetic mapping. Mamm Gen 12:930–932
Manning WJ, Tiedemann AV (1995) Climate change: potential effects of increased atmospheric carbon dioxide (CO2), ozone (O3), and ultraviolet-B (UV-B) radiation on plant diseases. E. Environ Pollut 88:219–245
Mehta YR, Gaudencio CA (1991) Effects of tillage practices and crop rotation on the epidemiology of some major wheat diseases. In: Saunders DA (ed) In: Proceedings of the international conference on wheat for non-traditional warmer areas. CIMMYT, Mexico, pp 226–283
Oliver RP, Lord M, Rybak K, Faris JD, Solomon PS (2008) Emergence of tan spot disease caused by toxigenic Pyrenophora tritici-repentis in Australia is not associated with increased deployment of toxin-sensitive cultivars. Phytopathology 98:488–491
Rees RG (1982) Yellow spot, an important problem in the northeastern wheat areas of Australia. In: Hosford RM Jr (ed) Tan spot of wheat and related diseases workshop. North Dakota State University Agriculture Exp Stn, Fargo, pp 68–70
Rees RG, Platz JG (1983) Effects of yellow spot on wheat: comparison of epidemics at different stages of crop development. Aust J Agric Res 34:39–46
SAS Institute (2008) SAS/STAT user’s guide, Release 9.2. SAS institute, Cary, NC
Singh PK, Gonzalez-Hernandez JL, Mergoum M, Ali S, Adhikari TB, Kianian SF, Elias E, Hughes GR (2006) Identification and molecular mapping of a gene conferring resistance to Pyrenophora tritici-repentis race 3 in tetraploid wheat. Phytopathology 96:885–889
Singh PK, Mergoum M, Ali S, Adhikari TB, Hughes GR (2008a) Genetic analysis of resistance to Pyrenophora tritici-repentis races 1 and 5 in tetraploid and hexaploid wheat. Phytopathology 98:702–708
Singh S, Bockus WW, Sharma I, Bowden RL (2008b) A novel source of resistance in wheat to Pyrenophora tritici-repentis race 1. Plant Dis 92:91–95
Singh PK, Mergoum M, Adhikari TB, Shah T, Ghavami F, Kianian SF (2010) Genetic and molecular analysis of wheat tan spot resistance effective against Pyrenophora tritici-repentis races 2 and 5. Mol Breed 25:369–379
Sun XC, Bockus WW, Bai GH (2010) Quantitative trait loci for resistance to Pyrenophora tritici-repentis race 1 in a Chinese wheat. Phytopathology 100:468–473
Tadesse W, Hsam SLK, Wenzel G, Zeller FJ (2006a) Identification and monosomic analysis of tan spot resistance genes in synthetic wheat lines (Triticum turgidum L. × Aegilops tauschii Coss.). Crop Sci 46:1212–1217
Tadesse W, Hsam SLK, Zeller FJ (2006b) Evaluation of common wheat cultivars for tan spot resistance and chromosomal location of a resistance gene in the cultivar ‘Salamouni’. Plant Breed 125:318–322
Tadesse W, Hsam SLK, Wenzel G, Zeller FJ (2008) Chromosome location of a gene conferring resistance to Pyrenophora tritici-repentis in Ethiopian wheat cultivars. Euphytica 162:423–430
Tadesse W, Schmolke M, Hsam SLK, Mohler V, Wenzel G, Zeller FJ (2010) Chromosomal location and molecular mapping of a tan spot resistance gene in the winter wheat cultivar Red Chief. J Appl Genet 51:235–242
Wolpert TJ, Dunkle LD, Ciuffetti LM (2002) Host-selective toxins and avirulence determinants: what’s in a name? Annu Rev Phytopathol 40:251–285
Zhang Z, Friesen TL, Simons KJ, Xu SS, Faris JD (2009) Identification, development and validation of markers for marker-assisted selection against the Stagonospora nodorum toxin sensitivity genes Tsn1 and Snn2 in wheat. Mol Breed 23:35–49
Zhang Z, Friesen TL, Xu SS, Shi GJ, Liu ZH, Rasmussen JB, Faris JD (2011) Two putatively homoeologous wheat genes mediate the recognition of SnTox3 to confer effector-triggered susceptibility to Stagonospora nodorum. Plant J 65:27–38
Acknowledgments
The authors thank Danielle Holmes and Jamie Hauff for technical assistance. This research was supported by USDA-ARS CRIS project 5442-22000-033-00D. Mention of trade names or commercial products in this publication is solely for the purpose of providing specific information and does not imply recommendation or endorsement by the U.S. Department of Agriculture. USDA is an equal opportunity provider and employer.
Author information
Authors and Affiliations
Corresponding author
Rights and permissions
About this article
Cite this article
Faris, J.D., Abeysekara, N.S., McClean, P.E. et al. Tan spot susceptibility governed by the Tsn1 locus and race-nonspecific resistance quantitative trait loci in a population derived from the wheat lines Salamouni and Katepwa. Mol Breeding 30, 1669–1678 (2012). https://doi.org/10.1007/s11032-012-9750-7
Received:
Accepted:
Published:
Issue Date:
DOI: https://doi.org/10.1007/s11032-012-9750-7