Abstract
Thermogravimetry has been widely applied to the study of wood and cellulose materials. There is a general agreement that decomposition of hemicellulose, cellulose, and ligning take place in a relatively narrow range of temperature, partially overlapping. There is no a definitive demonstration of which thermal feature corresponds to each component. In this study, three hardwood and two softwood species were considered: Castannea sativa, Eucaliptus globulus, Quercus robur, Pinus pinaster, and Pinus sylvestris. Thermogravimetric analysis of wood powder, ethanol-extracted wood, holocellulose, and lignin, obtained from those species revealed some important differences between hardwood and softwood holocelluloses and an important role of the ethanol-extractives, which explain the different behavior observed in both kinds of wood. FTIR spectra obtained from the evolved gases helped to clarify some degradation steps.
Similar content being viewed by others
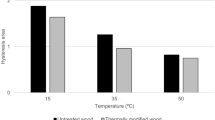
Explore related subjects
Discover the latest articles, news and stories from top researchers in related subjects.Avoid common mistakes on your manuscript.
Introduction
The major chemical components of wood are cellulose, hemicelluloses, lignin, and extractives [1]. Thermal stability of wood is usually studied by thermogravimetry (TG) [2–11]. Wood materials are known to present different degradation profiles depending on the wood composition. Cellulose presents higher thermal stability than hemicelluloses and lignin. Holocellulose is a combination of cellulose (a glucan polymer) and hemicellulose (mixtures of polysaccharides) [12]. This behavior was related to the fact that cellulose is highly crystalline and hemicelluloses and lignin are amorphous [13]. Hemicelluloses are the least thermally stable of the wood components, due to the presence of acetyl groups [14]. It was also reported that lignin degradation starts at relatively low temperatures, proceeding over a wide range of temperature [15]. Several extractives were obtained from wood with different solvents and by different methods. The extractives fraction usually includes lipids, phenolic compounds, terpenoids, fatty acids, resin acids, steryl esters, sterol, and waxes [16–19]. The amount of extractives is always small, usually in the 2–5% range [20]. Nevertheless, they significantly contribute to wood properties, such as mechanical strength and color [21]. Thus, the amount and type of these extractives generally affects the wood quality. The amount of extractives differs between wood species and is also influenced by several factors, including the growing conditions and the amount of heartwood [22]. The influence of some wood extractives on the thermal stability of wood has been studied in some cases. Mészáros et al. [23] found some differences between extracted and un-extracted wood samples. They also studied thermal degradation of the wood extractives from Robinia pseudoacacia. It was also reported that the removal of extractives produces a decrease in the fixed carbon content, a decrease in char yield and a displacement of the entire thermogravimetric curve toward higher temperatures [24]. The role of ethanol/cyclohexane extractives on the thermal degradation of wood from four species was investigated [2]. The aim of this study is to clarify the role of some of the main constituents of wood through TG analysis of wood powder, ethanol-extracted powder, holocellulose, and lignin. The effect of ethanol-extractives is investigated in softwood and hardwood species. In this context, Fourier transform infrared (FTIR) and mass spectroscopy (MS) analysis of the evolved gases may help to understand some degradation steps [9, 25–28].
Experimental
Wood samples from five species, namely Castannea sativa and Pinus pinaster, Pinus sylvestris, Eucaliptus globulus and Quercus robur, were investigated. The following samples were prepared from the raw material: wood powder, ethanol-extracted wood, holocellulose, and ligning obtained from these species. Chunks of 2-year-old timber were converted into powder by a Siebtechnik rotary disc mill. Part of the powder was extracted for 6 h with ethanol in soxhlet and then, holocellulose was obtained by the Browning method. A fraction of the extracted powder was destined to obtain lignin by the TAPPI222 standard.
Thermal stability studies were performed by TG in a TA Instruments 2960 SDT with a 100 mL/min flow of Nitrogen. Sample sizes of about 10 mg were used in all case. The samples were placed in open Alumina crucibles. The thermal program consisted of a 10 min isothermal step at 115 °C, followed by a 20 °C/min ramp from 20 to 900 °C. The isothermal step was aimed to remove hygroscopic water, which could interfere with the low temperature degradation steps. The SDT instrument was attached to a Bruker Vector 22 spectrometer and FTIR spectra were continuously obtained from the gases evolved in the holocellulose experiments.
Results and discussion
Figure 1 shows the time derivative of TG curves (DTG) corresponding to powder, extracted powder, and holocellulose of both species. It is remarkable that the non-extracted powder curves present a similar shape but with two important differences: the main degradation appears as an important peak with a shoulder on the low-temperature side in both cases. This peak-shoulder may be in principle assigned to the cellulose–hemicellulose, partially overlapping with lignin. But this peak appears at about 50 °C below in the case of Castannea than in the case of Pinus. It indicates the presence of other components, different in each species, which affect the thermal stability of cellulose and hemicellulose. Another difference between both curves is a shoulder at 250 °C on the Pinus curve. That shoulder cannot be observed on the Castannea curve, but it may be hidden by the main degradation process. On the other hand, it can be observed that the ethanol-extracted samples present approximately the same stability in both cases, which is similar to that observed in the case of non-extracted Pinus, with a peak at 375 °C and a shoulder at 315 °C. The different behavior observed before and after the extraction in the case of Castannea indicates a degradation promoting effect of the Castannea ethanol-extractives. In the case of Pinus the overall stability was not improved by the extraction, but the shoulder at 250 °C disappeared. Thus, that shoulder represents some ethanol-extractives, different from those observed in Castannea, which do not affect the thermal stability of cellulose and hemicellulose. In the case of Castannea, the extractive degradation takes place simultaneously to the degradation of other compounds, which suggests that the extractives are more intimately mixed with the other components of the wood in the case of Castannea than in the case of Pinus. Thus, in the case of Castannea, the extractives degradation would promote the degradation of the other wood components. A shoulder is observed at about 420 °C in all the ethanol-extracted and non-extracted powder samples. This high-temperature shoulder does not appear to be affected by the extraction process and can be easily assigned to the end of an overlapping degradation process of ligning. DTG plots of holocellulose are also shown in Fig. 1. The curves obtained from both species are very alike, with a peak at 350 °C and a shoulder at 280 °C. Since holocellulose is composed of cellulose and hemicellulose [12], the peak at 350 °C can be easily assigned to cellulose and the shoulder at 280 °C to holocellulose. The main difference between both species is that the peak is slightly narrower in the case of Pinus and thus the shoulder is more evident in that case. Figure 2 shows how the holocellulose DTG plots can be grouped into two groups: softwoods (P. pinaster and P. sylvestris) and hardwoods (C. sativa, E. globulus, and Q. robur). The fact that the hardwood peaks are broader and more asymmetric than the softwood ones indicates a more complex degradation process, involving other compounds whose degradation takes place in the middle of hemicellulose and cellulose. A comparison of the holocellulose and ethanol-extracted powder curves, in Fig. 1, shows a much lower thermal stability of holocellulose (about 50 °C). This is an indication of an important stabilizing effect of lignin, which is structurally mixed with cellulose and holocellulose in the ethanol-extracted samples. It can be also observed in Fig. 1 a small peak in the range from room temperature to 120 °C. That peak is present in all the curves, but it is higher in the case of holocelluloses. Since all the samples were initially preheated at 115 °C, the possibility of absorbed water is excluded. That peak can be explained as desorption of absorbed nitrogen, which was absorbed while cooling from 115 °C to room temperature under nitrogen atmosphere. Since holocellulose was obtained by removal of the cementing lignin, its structure is much less compact, with a higher surface area for gas interaction.
Figure 3 shows the DTG plots obtained from the non-extracted and ethanol-extracted powder samples. Considering the non-extracted samples, all of them differ among themselves, especially the hardwood ones. On the other hand, the ethanol-extracted samples can be classified into two groups: hardwoods and softwoods. The main difference between the two groups is a shoulder at about 290–300 °C. That difference is a consequence of the different nature of deciduous and conifers lignins [29], which are chemically bonded to hemicellulose. Figure 4 shows an overlay of the lignin DTG plots obtained according to the TAPPI222 standard. It is important to note that it is not possible to obtain lignin without altering its structure and it is difficult to obtain identical samples even using the same method [30, 31].
Nevertheless, although the extracted lignins are altered with respect to their native conditions, it can be observed in Fig. 4 that hardwood lignins experience a faster degradation in the 290–300 °C. This fact explains the different behavior of softwood and hardwood ethanol-extracted samples. The ranges of degradation are approximately the same for all the samples, although a long-degradation process was observed at high temperature in Q. robur. Nevertheless, these results do not represent the behavior of lignin in wood since the separation process modifies the structure of lignin and removes the interactions between fractions [32].
Since the aforementioned DTG results of holocelluloses in Fig. 2 indicate the presence of a compound different from hemicellulose and cellulose, FTIR spectra of the evolved gases were analyzed. Guaiacol was identified along some degradation steps in all cases. This aromatic compound cannot be originated from cellulose or hemicellulose and is a typical degradation product of lignin. It means that a part of the lignins remained with holocellulose after extraction by the Browning method. A typical absorption band of guaiacol is 1,263 cm−1. That band does not interfere with CO, CO2, or any other compound of the evolved gases. Figure 5 shows the P. pinaster and C. sativa absorbance traces at 1,263 cm−1, which represent the degradation of the remaining lignins. The shape relation of these curves is similar to that observed in the DTG curves of Fig. 2. Thus, the different behavior of both kinds of holocelluloses does not come from the holocelluloses themselves, but from the remaining lignins, which are different and behave differently. It is important to notice that these remaining fractions of the lignins are intimately bonded to hemicellulose so that their behavior is different than that observed in Fig. 4 for extracted lignins. On the other hand, Fig. 6 shows the absorbance traces at 2,104 and 2,351 cm−1, which correspond to CO and CO2, respectively. Both traces show a maximum at the same temperature, which can be easily related to cellulose. Nevertheless, the CO2 trace shows a shoulder at lower temperature, which is not present in the CO trace. This means that CO2 is produced by the degradation of both hemicellulose and cellulose, but CO comes mainly from cellulose. A quick comparison of the CO2 shoulders indicates that hemicellulose degrades earlier in the case of P. pinaster than in the C. sativa one.
Conclusions
Comparison of powder, ethanol-extracted powder, and holocellulose DTG traces obtained in Nitrogen atmosphere allowed to identify features corresponding to cellulose, hemicellulose, and extractives.
A different effect of ethanol-extractives was observed in thermal stability of softwoods and hardwoods. The softwood extractives degrade at lower temperature than the other wood components and do not affect their thermal stability. In the case of hardwood, the extractives clearly promote the degradation of the other components.
While the unextracted samples of hardwood powder behave very differently, the ethanol extracted ones behave similarly. The unextracted samples of softwood powder also behave differently, but after ethanol extraction their behaviors become much alike. Nevertheless, even after extraction, the hardwood and softwood samples behave differently. A higher stability of softwood with respect to softwood was observed in ethanol-extracted samples. That difference was explained as a consequence of the different nature of deciduous and conifers lignins.
The study of holocelluloses revealed a more complex degradation process in the case of hardwoods, indicating the presence of other compounds whose degradation takes place simultaneously to that of hemicellulose and cellulose.
FTIR analysis of the gases evolved from holocelluloses tests evidenced the presence of some remaining lignin, which behaves differently in the case of softwoods and hardwoods, and which is responsible of the different behavior of these holocelluloses. It was also found that Pinus hemicellulose degrades at lower temperature than the Castannea one.
References
Fengel D, Wegener G. In: Wood: chemistry, ultrastructure, reactions. Berlin: W. de Gruyter; 1984.
Shebani AN, van Reenen AJ, Meincken M. The effect of wood extractives on the thermal stability of different wood species. Thermochim Acta. 2008;471:43–50.
Carrier M, Loppinet-Serani A, Denux D, Lasnier J-M, Ham-Pichavant F, Cansell F, Aymonier C. Thermogravimetric analysis as a new method to determine the lignocellulosic composition of biomass. Biomass Bioenergy. 2011;35:298–307.
Wu C-H, Chang C-Y, Lin J-P. Pyrolysis kinetics of paper mixtures in municipal solid waste. J Chem Tech Biotechnol. 1997;68:65–74.
Raveendran K, Ganesh A. Heating value of biomass and biomass pyrolysis products. Fuel. 1996;75:1715–20.
Gašparovič L, Koreňová Z, Jelemenský Ľ. Kinetic study of wood chips decomposition by TGA. Chem Pap. 2009;64:174–81.
Yorulmaz SY, Atimtay AT. Investigation of combustion kinetics of treated and untreated waste wood samples with thermogravimetric analysis. Fuel Proc Tech. 2009;90:939–46.
Severiano L, Lahr F, Bardi M, Machado L. Evaluation of the effects of gamma radiation on thermal properties of wood species used in Brazilian artistic and cultural heritage. J Therm Anal Calorim. 2011;106:783–6.
Qu H, Wu W, Jiao Y, Xie J, Xu J. Investigation on the thermal decomposition and flame retardancy of wood treated with a series of molybdates by TG–MS. J Therm Anal Calorim. 2011;105:269–77.
Budrugeac P, Emandi A. The use of thermal analysis methods for conservation state determination of historical and/or cultural objects manufactured from lime tree wood. J Therm Anal Calorim. 2010;101:881–6.
Tarrio-Saavedra J, Naya S, Francisco-Fernandez M, Lopez-Beceiro J, Artiaga R. Functional nonparametric classification of wood species from thermal data. J Therm Anal Calorim. 2011;104:87–100.
Pettersen RC. The chemical composition of wood. In: Rowell R, editor. The chemistry of solid wood, vol. 207. Washington: American Chemical Society; 1984. p. 57–126.
Hill CAS. Wood modification: chemical thermal and other processes. Chichester: Wiley; 2006.
Bourgois J, Bartholin MC, Guyonnet R. Thermal treatment of wood: analysis of the obtained product. Wood Sci Technol. 1989;23:303–10.
Nassar M, MacKay G. Mechanism of thermal decomposition of lignin. Wood Fiber Sci. 1984;16:441–53.
Dorado J, Claassen FW, van Beek TA, Lenon G, Wijnberg JBPA, Sierra-Alvarez R. Elimination and detoxification of softwood extractives by white-rot fungi. J Biotechnol. 2000;80:231–40.
Fernandez MP, Watson PA, Breuil C. Gas chromatography-mass spectrometry method for the simultaneous determination of wood extractive compounds in quaking aspen. J Chrom A. 2001;922:225–33.
Kallioinen A, Vaari A, Rättö M, Konn J, Siika-aho M, Viikari L. Effects of bacterial treatments on wood extractives. J Biotechnol. 2003;103:67–76.
Ishida Y, Goto K, Yokoi H, Tsuge S, Ohtani H, Sonoda T, Ona T. Direct analysis of phenolic extractives in wood by thermochemolysis-gas chromatography in the presence of tetrabutylammonium hydroxide. J Anal Appl Pyrol. 2007;78:200–6.
Zhang X, Nguyen D, Paice MG, Tsang A, Renaud S. Degradation of wood extractives in thermo-mechanical pulp by soybean lipoxygenase. Enzym Microb Tech. 2007;40:866–73.
Pandey K. A note on the influence of extractives on the photo-discoloration and photo-degradation of wood. Polymer Degrad Stabil. 2005;87:375–9.
Hillis W. Formation and properties of some wood extractives. Phytochemistry. 1972;11:1207–18.
Mészáros E, Jakab E, Várhegyi G. TG/MS, Py-GC/MS and THM-GC/MS study of the composition and thermal behavior of extractive components of Robinia pseudoacacia. J Anal Appl Pyrol. 2007;79:61–70.
Várhegyi G, Grønli MG, Di Blasi C. Effects of sample origin, extraction, and hot-water washing on the devolatilization kinetics of chestnut wood. Ind Eng Chem Res. 2004;43:2356–67.
Artiaga R, Cao R, Naya S, González-Martín B, Mier J, García A. Separation of overlapping processes from TGA data and verification by EGA. J ASTM Int. 2005;2:12795.
Pan W, Whitely N, Xu W, Li S. Characterization of polymeric materials by thermal analysis spectroscopy and microscopic techniques. In: Artiaga R, editor. Thermal analysis. Fundamentals and applications to material characterization. A Coruña: Universidade da Coruña, Servicio de Publicaciones; 2005. p. 141–154.
Boerjan W, Ralph J, Baucher M. Lignin biosynthesis. Ann Rev Plant Biol. 2003;54:519–46.
Zhao H, Cao Y, Sit S, Lineberry Q, Pan W. Thermal characteristics of bitumen pyrolysis. J Thermal Anal Calorim. 2011. doi:10.1007/s10973-011-1590-x.
Hu S, Hu Y, Song L, Lu H. The potential of ferric pyrophosphate for influencing the thermal degradation of cotton fabrics. J Thermal Anal Calorim. 2011. doi:10.1007/s10973-011-1732-1.
Evans RJ, Milne TA, Soltys MN. Direct mass-spectrometric studies of the pyrolysis of carbonaceous fuels: III primary pyrolysis of lignin. J Anal Appl Pyrol. 1986;9:207–36.
Garcia F, Rodriguez JJ, Martin F. Posibilidades de aprovechamiento de la lignina en la industria química. Ingen Quim 1984;10:249–254.
Caballero J, Font R, Marcilla A. Comparative study of the pyrolysis of almond shells and their fractions, holocellulose and lignin. Product yields and kinetics. Thermochim Acta. 1996;276:57–77.
Acknowledgements
This study was partially funded by the Spanish Ministerio de Educacion y Ciencia MTM2008-00166 and MAT2010-21342-C02-01. The first author acknowledges Consellería de Educación e Ordenación Universitaria of Xunta de Galicia (Spain) for supporting this study.
Author information
Authors and Affiliations
Corresponding author
Rights and permissions
About this article
Cite this article
Sebio-Puñal, T., Naya, S., López-Beceiro, J. et al. Thermogravimetric analysis of wood, holocellulose, and lignin from five wood species. J Therm Anal Calorim 109, 1163–1167 (2012). https://doi.org/10.1007/s10973-011-2133-1
Published:
Issue Date:
DOI: https://doi.org/10.1007/s10973-011-2133-1