Abstract
Mn-doped CdS nanoparticles were successfully prepared by the chemical precipitation method. The effect of Mn doping on the structure, optical, and magnetic properties of the prepared samples was studied. Cubic CdS is indicated by XRD investigation and no change in CdS crystal structure was noted by Mn doping due to the low concentration of Mn doping. The morphology of the pure and 0.07% doped samples was investigated by a high-resolution transmission electron microscope (TEM). TEM images revealed mosaic-shaped grains with a size range of 7–8 nm. The thermal behavior and stability of the pure sample were investigated by differential scanning calorimetry (DSC) and thermogravimetric analysis (TGA) in the argon atmosphere. The whole weight loss over the temperature range of 25–700 °C was about 17.4% which reveals the thermal stability of the prepared compound. The optical properties of the prepared samples were studied through diffuse reflection (DR). The transition energy increases only by the initial addition of Mn and then did not get affected by more Mn. The impact of doping on the energy bandgap, refractive index, and dielectric constant was discussed. Magnetic properties of Mn-doped CdS were investigated by a vibrating-sample magnetometer at room temperature. The results indicated an anti-ferromagnetic property with weak ferromagnetic behavior with no saturation.
Similar content being viewed by others
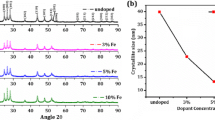
Avoid common mistakes on your manuscript.
1 Introduction
Diluted magnetic semiconductors (DMS) have received attracting interest and have been investigated for potential applications in spin-based information-processing technology exhibiting both semiconductor and magnetic properties [1,2,3]. The charge degree and the spin can be controlled by the doping elements in DMS materials [4]. DMS are formed when a part of cations of semiconducting material is replaced by ions of a transition metal that have filled d states (Cr, Ni, Co, Fe, and Mn) [5].
The transition metal ions are incorporated in II–VI semiconductors that are particularly interesting in the DMS field [6]. Ferromagnetic at room temperature and diluted magnetic semiconductors are intense candidates for spintronic applications [7]. Diluted magnetic semiconductors (DMS) display enhanced magnetic and optical properties owing to the exchange interaction of electron–hole bands with the magnetic atoms [8]. By changing the concentration of magnetic ions, the energy gap, lattice constant, optical, and magnetic properties in DMS materials can be modified [9].
Nanomaterials are described as materials with at least one exterior dimension of 1 to 100 nm [10]. Nanomaterials can exist naturally, be manufactured as by-products of combustion reactions, or be engineered specifically to serve a certain function. Physical and chemical aspects of these materials may be varied than those of respective bulk form [11]. Nanomaterials are included in a multitude of industrial sectors, including healthcare, cosmetics, ecological sustainability, and air purification, due to their capacity to create materials in a specific way to perform a specified function [12]. Nanomaterials are used in a multitude of strategies in the healthcare industry, one of which is drug delivery. An instance of this technique is the creation of nanoparticles for the delivery of chemotherapy treatments effectively to cancers and also to drug delivery to damaged regions of arteries to combat cardiovascular disease [13].
The nanostructured properties of materials were strongly affected by the synthesis method. Different approaches were employed in nanomaterials preparation such as co-precipitation [14,15,], electrochemical reductions [15], impregnation [16], microwave-assisted [17], thermal decomposition [18], and hydrothermal [19]. Co-precipitation as a traditional method is employed in nanomaterials synthesis since it is easy, cheap, and performed with low temperature or pressure. This method is affected by the pH of the medium, concentration of the starting materials, and precipitating reagents [20].
DMS in nanometer structure are showing large magneto-optical properties and low-dimensional exciton properties represented by the interaction between electrons and magnetic ions in quantum-confined DMS nanomaterials [21].
Nanoparticles DMS doped with Mn have been marked to display magneto-optical properties that appear different from the corresponding bulk material. (II–VI) group (CdS, ZnS, and ZnO) semiconductors have a wide bandgap that is related to a wide range of applications in many fields like X-ray detectors, window material for solar cells, photocatalysis, light-emitting diodes, address decoders, biological sensors, and gas detectors [22, 23].
In nanoscale, there is nonferromagnetic ordering, while the size of the nanoparticles would be smaller than the length required for a long-range magnetic order [24]. Moreover, it is important to study the smaller size which is intense to occur magnetic interactions, as an interesting factor for designing spintronic devices and smaller memory [25]. There are a large number of reports on the CdS-based DMS with transition metal other than Mn. Girbabu et al. [5] showed the magnetic properties effect in CdS:Co synthesis by chemical co-precipitation method. Chauhan et al. [24] studied the photocatalytic activities of CdS:Fe in visible light by the synthesized chemical precipitation method. A few reports are known on Mn-doped thin films [26]. Salimian et al. studied structural, optical, and magnetic properties of CdS:Mn nanoparticles [27]. In the present work, Mn-doped CdS nanoparticles were prepared by the chemical precipitation method. The effect of Mn concentration on the crystal structure, magnetic, and optical properties of the prepared samples was studied.
2 Experimental
2.1 Materials
All chemicals used in this work, cadmium acetate [Cd(CH3COO)2], sodium sulfide [Na2S], and manganese chloride [MnCl3], are purchased from Sigma-Aldrich and were used as received without further purification.
2.2 Preparation
Cadmium acetate [Cd(CH3COO)2] 200 mM is dissolved in 0.1 l deionized H2O by stirring for 30 min at temperature 80 °C. 200 mM sodium sulfide (Na2S) dissolved in 0.1 l deionized H2O is added dropwise to the former solution. By stirring the mixture for 180 min at temperature 80 °C, a precipitate is produced. This precipitate is collected by centrifugation and then rinsed by deionized H2O, followed by drying at 100 °C temperature for 8 h where the CdS powder is obtained.
Similarly, the manganese-doped CdS nanoparticles were prepared. A 2 mM, 6 mM, 10 mM, and 20 mM of manganese chloride (MnCl3) (equivalent to 0.00%, 0.03%, 0.05%, and 0.07%) dissolved in 0.1 l deionized H2O are added separately to the primary solution of Cd(CH3COO)2. The same steps are then followed, where 200 mM sodium sulfide (Na2S) dissolved in 0.1 l deionized H2O is added dropwise to the former solution. By stirring the mixture for 180 min at temperature 80 °C, a precipitate is produced. This precipitate is then collected by centrifugation and then rinsed by deionized H2O, followed by drying at 100 °C temperature for 8 h where the Mn-doped CdS powder is obtained.
2.3 Characterization
The XRD analysis of prepared powders was obtained by Philips model X’pert diffractometer (Philips Co., Germany) with CuKα source radiation and operated at voltage 40 kV and current 25 mA.
The morphology of the sample powders was carried out by a high-resolution transmission electron microscope (HRTEM) JEOL, Model: JEM 2100 (JEOL Co., USA).
Bruker vertex 80 (Bruker Co., Germany) Fourier-transform infrared spectroscopy (FTIR) was used to record the sample powder's output spectra.
In an argon atmosphere and 10 °C/min heating rate, the differential scanning calorimetry (DSC) and thermogravimetric analysis (TGA) of pure sample powder were done by employing Q600 SDT thermal analyzer system (TA Instruments, USA) in the range of 25–700 °C.
UV–Vis spectra are obtained in the wavelength range of 200–2500 nm by UV–Vis spectrophotometer Model: UV- 2450 (SHIMADZU Co., Japan).
Magnetic measurements are carried out by vibrating-sample magnetometer (VSM) model: 7410 series (Lake Shore Cryotronics, USA).
3 Results and discussion
3.1 XRD studies
Figure 1a shows the XRD spectra of the Cd1−xMnxS doped with x = 0.0., 0.03, 0.05, and 0.07%. The XRD resultant peaks are matched with Cubic CdS in agreement with JCPDS no. 075-1546. The peaks broadening are an indicator of the nano-CdS:Mn formation [28]. By Mn doping, no significant changes were observed in the resultant spectra which means that there is no change in CdS crystal structure by Mn doping; this is due to the low concentration of Mn doping. This result was observed before by Ganguly et al. [29]. Weichang et al. [30] found that the positions of the resultant peaks of CdS and Mn-doped CdS are almost the same. Angshuman et al. also observed that the CdS XRD profiles did not change by Mn2+ ion addition. In Mn ion-implanted CdS films, Chandramohan et al. [31] noticed that the XRD peak positions are hardly changed by Mn doping. CdS doped with 0.7 Mn sample XRD data were obtained by the FullProf software, using the pseudo-Voigt profile for the peaks profile simulation [32]. The background fitting was done by linear interpolation [33].
The Scherrer’s method was employed in the mean crystallite size estimating through the following equation [14, 34]:
where D, K, β, and θ signs to the mean crystallite size, the shape factor (≈ 0.9), the XR wavelength, the full width at half of the peak maximum intensity in radians, and Bragg diffraction angle, respectively. The mean value of crystallite size of Mn-doped CdS is 3.69, 3.79, 3.81, and 3.83 nm for 0.00%, 0.03%, 0.05%, and 0.07% Mn, respectively.
The number of dislocation lines per unit area (dislocation density δ) is estimated by [35, 36]
The corresponding dislocation density was found as 73 × 10–3, 70 × 10–3, 68.8 × 10–3, and 68.1 × 10–3 lines/nm2 for 0.00%, 0.03%, 0.05%, and 0.07% Mn, respectively.
For the pure sample (0.00% Mn), the refinement was done with the parameters U, V, W, unit cell parameters' mixing coefficient η, and the symmetry parameters converged to χ2 = 0.00345. Figure 1b shows the refined result of the sample XRD. The structure was found to be cubic [space group: F -4 3 m (#216-1)] with the plane indices as mentioned in the figure. The lattice parameters were obtained as a = 5.81799 Å, b = 5.81799 Å, c = 5.81799 Å, α = 90°, β = 90°, γ = 90°, and V = 196.9332 Å3. Using the Rietveld data, the geometrical structure of CdS, Fig. 1c, was drawn using the VESTA software [37].
3.2 Morphology studies
Figure 2 shows the transmission electron microscope (TEM) images of the CdS NPs doped with 0.00, 0.03, 0.05, and 0.07% Mn. Figure 2a and c shows the TEM of 0.00 and 0.07% Mn-doped CdS samples. Both sample images revealed mosaic-shaped grains with a size range of 7–8 nm as obtained in distribution histograms, Fig. 2b and d. The inset of Fig. 2a and c demonstrates the equivalent selected area electron diffraction (SAED) pattern of 0.00 and 0.07% Mn-doped CdS samples, respectively. The SAED presenting bright rings of random orientations indicate a polycrystalline structure of the prepared samples.
3.3 Thermal studies
The thermal analysis includes many approaches where some physical variable is noted against temperature. Thermal patterns provide a good evaluation of quality, constituents, and material applications [38]. Thermogravimetric analysis (TGA) is a form of thermo-analytical test which is carried out to check the weight variations with temperature variations [38]. TGA is frequently utilized for figuring out degradation, moisture content, chemical changes, and decomposition processes. The TGA curves are characteristics of a substance due to a unique series of physiochemical responses that are related to molecular composition and structure. Weight variations are attributable to the break and/or creation of physical and chemical bonds that may generate a volatile product or form a heavier product. Via such acquired data, the different chemical processes thermodynamics and kinetics can be estimated [39,40,41,42,43]. A simultaneous TGA–DSC measures both weight loss and heat flow in material against temperature or time in an adjusted condition [38]. TGA–DSC coexisting measurement not only enhances output results but also simplifies the interpretation of the results. The data acquired from DSC enable differentiation among endothermic and exothermic events lacking weight loss such as melting and crystallization processes and also that have a weight loss such as degradation process [38].
The resultant curve of weight loss alongside the DSC curve of pure CdS from 25 to 700 °C is presented in Fig. 3. There is a sharp weight loss from 25 to 90 °C (corresponds and coincides to an intense exothermic peak 79.3 °C in DSC trace) followed by a weight loss with a lower rate from 90 °C to about 214 °C (corresponds to 120–210 °C change section in DSC trace). These two weight losses or events observed in both TGA and DSC curves are related to the evaporation of surface- and crystalline-absorbed water of materials [44]. From about 215 °C to the end of the heating range, there is a very low rate of weight loss which is attributed to decomposition or degradation of the compound [45]. The whole weight loss over the temperature range of 25–700 was about 17.4%.
3.4 Optical studies
Diffuse reflection (DR) is the light reflected by an irregular or granular surface in a way that a falling beam is reflected at several perspectives. Diffusely reflected rays do not follow Snell’s law as a common reflection. Diffuse reflection comes from the rays penetrating the sample interior and returned to the surface just after several scatterings. So, the DR spectra will demonstrate absorbance and reflectance caused by efforts via transmission, internal and specular reflectance factors, and also scattering.
The Kubelka–Munk (KM) model, widely used and accepted in DR analysis, is described by the next function [46]:
where α, (R∞), R∞, B, hυ, Eg, and r are absorption coefficients, the Kubelka–Munk formula, the diffuse reflectance, constant, photon energy, optical bandgap, and an index, respectively. r index is used to determine the type of energy band transition where it is ½, 2, 3, and 3/2 for direct allowed, indirect allowed, direct forbidden, and indirect forbidden [47]. Therefore, through the Tauc model [48], the type and the value of the transition are estimated [48]:
where C is the proportionality constant.
In the wavelength range of 200–2500 nm, the diffuse reflectance was recorded for Mn-doped CdS samples and are presented in Fig. 4. All samples' DR rises as the wavelength rises to 700 nm and then suffers from interference. The intensity of DR is nearly the same for all samples. The addition of Mn did not affect the intensity or the absorption edge of the samples.
The absorption coefficient which is relative to the Kubelka–Munk function, as in Eq. 3, was computed and drawn with the beam wavelength as in Fig. 5. The figure shows that an absorption shoulder appears for all samples at 253 nm. A sharp absorption peak is observed at 446 nm for pure CdS samples and shifted to 440 nm for all doped samples.
The absorption shoulder was found at 253 nm which indicates a blue shift in the absorption edge. The characteristic excitonic peak centered around 440 nm reveals the quantum confinement effect arising due to the small size of as-formed CdS nanoparticles [49].
The energy bandgap value and type are obtained from the Tauc relation, Eq. 4. The calculations presented in Fig. 6a and b show that both direct and indirect directions can be operated in these samples. Figure 7 shows that the indirect transitions have a higher value than the direct transitions and so the direct type is the more probable transition process. Also, in both direct and indirect transitions, the transition energy increase by adding 0.3 Mn and then did not affect by additional Mn.
The Mn-doped CdS nanocrystals' absorption spectrum is typical for quantum-sized nanoparticles with an absorption edge blue shift corresponding to the bulk form with bandgap (2.38 eV) [50]. There is no influence of Mn2+ ions on the bandgap values of CdS due to its low concentration [51]. Upon doping the Mn ions, the wavelength is shifted slightly and the change in the bandgap proposes an energy transfer between the excited states and the Mn2+ ions 3rd levels which are coupled by energy transfer processes [52].
The material refractive index is calculated by mathematical models such as Herve–Vandamme, Moss, Reddy, Anani, Ravindra, and Kumar-Singh [53, 54]. The obtained data are listed in Tables 1 and 2.
Figure 8a and b represents the behavior of the calculated refractive indices with Mn content for both direct and indirect transition cases, respectively. The refractive index decreases by adding Mn and takes a constant behavior by adding more Mn.
The dielectric constants [53] of the current samples are calculated and listed in Tables 3 and 4 for obtained transitions (direct and indirect), respectively. The change of dielectric with Mn content is similar to the refractive index change as presented in Fig. 9.
Duffy [55] and Reddy [56] models were employed to get the optical electronegativity for the obtained transitions (direct and indirect). Table 5 shows the output calculations of optical electronegativity. Figure 10 presents the optical electronegativity as a function of Mn content where it is slightly increased by Mn addition and tack a constant shape by more adding. From the obtained results and with considering Pauling's [57] suggestion, the prepared Mn-doped CdS are considered as covalent materials. This result also is in agreement with the obtained XRD data.
3.5 Magnetic studies
Figure 11 depicts the plot of magnetization against the magnetic field (M–H hysteresis loops) of Mn-doped CdS nanoparticles at room temperature. Previous research has shown that pure CdS nanoparticles are diamagnetic by nature [58]. The M–H curves of Mn-doped CdS nanoparticles (x = 0.03, 0.05, and 0.07) show anti-ferromagnetic characteristics with weak ferromagnetic behavior and no saturation. By a close look at M–H hysteresis loops in Fig. 11, we can note that the behavior of sample x = 0.03 is diamagnetic up to H = 1235 Oe and then reverse its direction in the positive direction and the same behavior in a negative value of applied magnetic field up to − 930 Oe is repeated. This behavior is attenuated with increasing Mn content for x = 0.05 and 0.07. The competition between diamagnetic and ferromagnetic content in samples can explain this behavior. In small value of applied magnetic field, the diamagnetic content has value larger than the ferromagnetic content; with increasing the applied magnetic field, the ferromagnetism overcomes the diamagnetism. The magnetization at maximum magnetic field (Mmax) for samples x = 0.03, 0.05, and 0.07 was 0.0328, 0.0335, and 0.0215, respectively. The Mmax increases by a small value from x = 0.03 to x = 0.05 but decreases with increasing Mn content at x = 0.07 as depicted in Fig. 11. Pradyumna Elavarthi et al. [59] hypothesized that ferromagnetism in Cr-doped CdS nanoparticles at room temperature results from the substitution of Cr in the CdS lattice for lower concentrations of Cr, whereas the enhanced antiferromagnetic interaction suppresses the ferromagnetism for higher concentrations of Cr. Also, Saikia [60] investigated ferromagnetic ordering in Fe-doped CdS. It was found that ferromagnetic ordering decreased with increasing Fe more than 0.03%. The origin of the room-temperature ferromagnetism in the dilute magnetic semiconductor materials may be due to (i) the existence of different types of defects or vacancies that increase in number as the dopant concentration raises, (ii) different kinds of exchange mechanisms have emerged in host materials between transition elements, and (iii) secondary phases that can occur throughout the synthesis process [61, 62]. Magnetic dipoles are positioned on the surface of the nanoparticles in the case of a small concentration of Mn, which interacts with surrounding dipoles to make them to be orientated in the same direction, as a result of the ferromagnetic ordering [63,64,65]. As the dopant concentration rises, the short-range superexchange interaction takes over, suppressing the ferromagnetic ordering and enhancing antiferromagnetic interactions between the magnetic dipoles [64, 66].
4 Conclusion
The cubic CdS and Mn-doped CdS prepared by the chemical precipitation method were investigated structurally, optically, and magnetically. The mosaic-shaped grains with a size range of 7–8 nm were observed by TEM. Over the temperature range of 25–700, CdS lost about 17.4% which indicates its thermal stability. Both direct and indirect optical gap transitions can be operated in these samples, but the direct type is the more probable type. The transition energy increase from 2.02 to 2.08 eV by adding 0.03 Mn and then did not get affected by more Mn addition. The refractive index and dielectric constant decrease by adding Mn and take a constant behavior by more addition of Mn. Mn-doped CdS nanoparticles demonstrated anti-ferromagnetic with weak ferromagnetic behavior with no saturation, where there is competition between the diamagnetic and ferromagnetic content in samples. The short-range superexchange interaction suppresses the ferromagnetism of the system and enhanced antiferromagnetic interactions between the magnetic dipoles.
References
A.A. Azab, A.M. Mansour, G.M. Turky, Sci. Rep. (2020)
E.H. El-Khawas, A.A. Azab, A.M. Mansour, Mater. Chem. Phys. (2020)
K.R. Kittilstved, W.K. Liu, D.R. Gamelin, Nat. Mater. 5, 291 (2006)
T. Dietl, Nat. Mater. 9, 965 (2010)
J.K. Furdyna, J. Appl. Phys. 64, R29 (1988)
R. Sathyamoorthy, P. Sudhagar, A. Balerna, C. Balasubramanian, S. Bellucci, A.I. Popov, K. Asokan, J. Alloys Compd. 493, 240 (2010)
S.A. Wolf, D.D. Awschalom, R.A. Buhrman, J.M. Daughton, S. Von Molnár, M.L. Roukes, A.Y. Chtchelkanova, D.M. Treger, Science 294, 1488 (2001)
M.A. Islam, M.S. Hossain, M.M. Aliyu, P. Chelvanathan, Q. Huda, M.R. Karim, K. Sopian, N. Amin, Energy Procedia (Elsevier Ltd, Amsterdam, 2013), pp. 203–213
H. Murai, T. Abe, J. Matsuda, H. Sato, S. Chiba, Y. Kashiwaba, Appl. Surf. Sci. 244, 351–354 (2005)
M. Rizwan, A. Shoukat, A. Ayub, B. Razzaq, M.B. Tahir, Nanomaterials: Synthesis, Characterization, Hazards and Safety (Elsevier, Amsterdam, 2021), pp. 31–54
S.S. Ray, R. Salehiyan, Nanostructured Immiscible Polymer Blends (Elsevier, Amsterdam, 2020), pp. 15–28
R. Bissessur, Polymer Science Nanotechnology (Elsevier, Amsterdam, 2020), pp. 435–453
J. Chen, X. Zhang, R. Millican, J. Sherwood, S. Martin, H. Jo, Y. Sup Yoon, B.C. Brott, H.W. Jun, Adv. Drug Deliv. Rev. 170, 142 (2021)
S. Zinatloo-Ajabshir, M. Salavati-Niasari, J. Mater. Sci. Mater. Electron. 26, 5812 (2015)
D.C. Clifford, C.E. Castano, J.V. Rojas, Radiat. Phys. Chem. 132, 52 (2017)
H.R. Mahmoud, Adv. Powder Technol. 27, 1446 (2016)
L. Gu, L. Qian, Y. Lei, Y. Wang, J. Li, H. Yuan, D. Xiao, J. Power Sources 261, 317 (2014)
X. Zhao, F. Zhang, S. Xu, D.G. Evans, X. Duan, Chem. Mater. 22, 3933 (2010)
J.R. Kim, K.Y. Lee, M.J. Suh, S.K. Ihm, Catal. Today 185, 25–34 (2012)
H.R. Mahmoud, S.A. El-Molla, M.A. Naghmash, Ultrasonics 95, 95 (2019)
M. Tanaka, Y. Masumoto, Solid State Commun. 120, 7 (2001)
Z. Huang, F. Sun, Y. Zhang, K. Gu, X. Zou, Y. Huang, Q. Wu, Z. Zhang, J. Colloid Interface Sci. 356, 783 (2011)
S. Salimian, S. FarjamiShayesteh, S. Salimian, J. Supercond. Nov. Magn. 25, 2009 (2012)
R. Chauhan, A. Kumar, R.P. Chaudhary, Appl. Surf. Sci. 270, 655 (2013)
K.K. Nanda, S.N. Sahu, Solid State Commun. 111, 671 (1999)
M. Tanaka, J. Lumin. 100, 163 (2002)
J. Zhuang, X. Zhang, G. Wang, D. Li, W. Yang, T. Li, J. Mater. Chem. 13, 1853 (2003)
A. Dandia, V. Parewa, K.S. Rathore, Catal. Commun. 28, 90 (2012)
A. Ganguly, S.S. Nath, Mater. Sci. Eng. B 255, 114532 (2020)
W. Zhou, D. Tang, B. Zou, Phys. E. 47, 162 (2013)
S. Chandramohan, A. Kanjilal, J.K. Tripathi, S.N. Sarangi, R. Sathyamoorthy, T. Som, J. Appl. Phys 105, 123507 (2009)
R.A. Young, D.B. Wiles, J. Appl. Crystallogr. 15, 430 (1982)
H. Belgaroui, M. Loukil, R. Karray, A. Ben Salah, A. Kabadou, J. Alloys Compd. (2010)
N. Hassan, A.M. Mansour, N. Roushdy, A.A.M. Farag, W.G. Osiris, Optik (Stuttg). 158, 1255 (2018)
E.M. El-Menyawy, I.T. Zedan, A.M. Mansour, J. Electron. Mater. 46, 4353 (2017)
A.A.M. Farag, F.S. Terra, A. Ashery, A.M. Mansour, Optoelectron. Adv. Mater. Rapid Commun. 11, 82 (2017)
A.M. El Nahrawy, A.M. Mansour, A.M. Bakr, A.B. Abou Hammad, ECS J. Solid State Sci. Technol. 10, 063007 (2021)
A.M. Mansour, Int. J. Microstruct. Mater. Prop. 15, 215 (2020)
A.M. Mansour, M. Nasr, H.A. Saleh, G.M. Mahmoud, Appl. Phys. A Mater. Sci. Process 125, 625 (2019). https://doi.org/10.1007/s00339-019-2920-2
A.M. Mansour, I.M. El Radaf, G.M. Mahmoud, Int. J. Microstruct. Mater. Prop. 14, 462 (2019)
A.M. El Nahrawy, A.B. Abouhammad, A.M. Bakr, T.I. Shaheen, A.M. Mansour, Appl. Phys. A 126, 654 (2020)
A.M. Mansour, I.M.E. Radaf, T.A. Hameed, G.B. Sakr, U.P.B. Sci, Bull. Ser. B 81, 134 (2019)
A.M. El Nahrawy, A.B.A. Hammad, A.M. Youssef, A.M. Mansour, A.M. Othman, Appl. Phys. A 125, 1 (2019)
B. Rao, B. Kumar, V. Reddy, T. Rao, G. Chalapathi, Chalcogenide Lett. 8, 177 (2011)
S. Riaz, Z.A. Raza, M.I. Majeed, Polym. Bull. 77, 775 (2020)
B.A. Hemdan, A.M. El Nahrawy, A.F.M. Mansour, A.B.A. Hammad, A.B. AbouHammad, A.B.A. Hammad, Environ. Sci. Pollut. Res. 26, 9508 (2019)
A.M. El Nahrawy, A.M. Mansour, A.B. AbouHammad, A.R. Wassel, Mater. Res. Express 6, 016404 (2019)
A.M. Mansour, SILICON 11, 1989 (2019)
F. Gao, Appl. Phys. Lett. 98, 193105 (2011)
M. Ramrakhiani, P. Vishwakarma, Synth. React. Inorg. Met. Nano-Metal Chem. 36, 95–105 (2006)
M. Ikeda, K. Itoh, H. Sato, J. Phys. Soc. Jpn. 25, 455 (1968)
F.J. Brieler, M. Fröba, L. Chen, P.J. Klar, W. Heimbrodt, H.A.K. Von Nidda, A. Loidl, Chem. Eur. J. 8, 185 (2002)
S.K. Tripathy, Opt. Mater. (Amst). 46, 240 (2015)
S. Pal, R. Kumar Tiwari, D. Chandra Gupta, A. Singh Verma, J. Mater. Phys. Chem. 2, 20 (2014)
J.A. Duffy, Phys. Chem. Glas. 42, 151 (2001)
R.R. Reddy, K. Rama Gopal, Y. NazeerAhammed, K. Narasimhulu, L. Siva Sankar Reddy, C.V. Krishna Reddy, Solid State Ionics 176, 401 (2005)
L. Pauling, The Nature of the Chemical Bond, an Introduction to Modern Structural Chemistry (Cornell University Press, Ethaca, 1960)
G. Murali, D.A. Reddy, B. Poornaprakash, R.P. Vijayalakshmi, B.K. Reddy, IOP Conference Series Materials Science Engineering (Institute of Physics Publishing, Bristol, 2015)
P. Elavarthi, A.A. Kumar, G. Murali, D.A. Reddy, K.R. Gunasekhar, J. Alloys Compd. 656, 510 (2016)
D. Saikia, J. Jami, J.P. Borah, Phys. B 565, 25 (2019)
C. Madhu, A. Sundaresan, C.N.R. Rao, Phys. Rev. B 77, 201306 (2008)
W.Z. Xiao, L.L. Wang, Q.Y. Rong, G. Xiao, B. Meng, J. Appl. Phys. 115, 213905 (2014)
P.K. Sharma, R.K. Dutta, A.C. Pandey, S. Layek, H.C. Verma, J. Magn. Magn. Mater. 321, 2587 (2009)
S. Kumar, N.K. Verma, J. Supercond. Nov. Magn. 28, 137 (2015)
P.K. Sharma, R.K. Dutta, A.C. Pandey, J. Colloid Interface Sci. 345, 149 (2010)
A. Sundaresan, C.N.R. Rao, Nano Today 4, 96 (2009)
Author information
Authors and Affiliations
Corresponding author
Ethics declarations
Conflict of interest
The authors declare that they have no known competing financial interests or personal relationships that could have appeared to influence the work reported in this paper.
Additional information
Publisher's Note
Springer Nature remains neutral with regard to jurisdictional claims in published maps and institutional affiliations.
Rights and permissions
About this article
Cite this article
Ibrahim, R.S., Azab, A.A. & Mansour, A.M. Synthesis and structural, optical, and magnetic properties of Mn-doped CdS quantum dots prepared by chemical precipitation method. J Mater Sci: Mater Electron 32, 19980–19990 (2021). https://doi.org/10.1007/s10854-021-06522-0
Received:
Accepted:
Published:
Issue Date:
DOI: https://doi.org/10.1007/s10854-021-06522-0