Abstract
The production of cellulosic man made fibres by the viscose process has been known for more than 120 years now, but still some aspects are not sufficiently understood in detail. The carbohydrates in the pulp are exposed to varying conditions during the manufacturing process. In the first production step of steeping, the strong alkaline treatment leads to undesirable loss reactions of the cellulose. In this study, a comprehensive kinetic model was developed for process simulation of cellulose degradation for the fist time comprising primary and secondary peeling, stopping and alkaline hydrolysis. A total chlorine free bleached beech sulfite pulp was treated with 18 % sodium hydroxide at 40, 50 and 60 °C for time periods up to 80 h. The corresponding reaction rates, activation energies and frequency factors for all reaction steps were calculated. The peeling-off reaction was of great significance for the cellulose yield loss, due to a contribution of the secondary peeling after random chain scission. The moderate decrease of the intrinsic viscosity and the changes in molar mass distribution indicated the validity of the assumption. Further, a reduction of the carbonyl and an increase of the carboxyl groups in the cellulose were observed due to the formation of the stable metasaccharinic acid at the reducing ends of the molecules. The fibre morphology was investigated by SEM measurements. Already short alkaline treatment times favored the dissolution of fibril fragments from the fibre surface leading to a smooth fibre surface.
Similar content being viewed by others
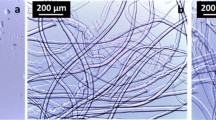
Explore related subjects
Discover the latest articles, news and stories from top researchers in related subjects.Avoid common mistakes on your manuscript.
Introduction
The production of regenerated cellulosic fibres is gaining importance due to an increasing demand in the global textile market. The inherent physiological properties of cellulose fibres cannot be sufficiently achieved by synthetic fibres (Eichinger 2012). Since these properties are requested in many fibre products, a minimum constant share of cellulosic fibres, including both natural and man-made fibres, of 33–37 % on the global fibre consumption has been predicted (Hämmerle 2011; Eichinger 2012). Further, a replacement of cotton by man-made cellulosic fibres will lead to a sustainability enhancement. A recent life cycle analysis confirmed that man-made cellulosic fibres have a much smaller carbon footprint as compared to cotton. (Shen and Patel 2010). On this account viscose staple production experienced a steady growth at an annual rate of 6.7 % during the last 10 years, slightly higher than that of polyester staple (6.6 %) (Bywater 2011).
Although the Lyocell process, using a direct cellulose solvent, has been successfully commercialized during the last 20 years, the viscose staple fibers remain the dominant man-made cellulose fiber due to their broad field of application. Particular needs concerning fiber properties can be easily accomplished as the process itself is long established and well engineered, but there is still potential for improvements.
In the first step of the viscose process, bleached dissolving wood pulp is treated with steeping lye which contains a sodium hydroxide concentration of about 18 wt%. In this step and during the subsequent pre-aging, the cellulose is activated for xanthation and its degree of polymerisation is adjusted to a level required for further processing.
Due to the high alkali content native cellulose I is entirely converted to cellulose II ensuring an increased reactivity towards derivatization (Kolpak et al. 1978; Krässig 1993). At the same time alkali-soluble hemicelluloses and undesired short-chain cellulose present in the pulp are removed through dissolution as well as through alkaline-induced degradation reactions. The predominant reaction of cellulose under steeping conditions is the peeling-off reaction due to beta-elimination.
Generally, the pathway of successive shortening of the molecule chains was investigated in connection with swelling and dissolution in cold alkali (Davidson 1934a). Later Richtzenhain suggested the degradation taking place at accessible reducing end groups (Richtzenhain et al. 1954) and isosaccharinic acids were identified as the main soluble products of alkaline peeling (Richards and Sephton 1957). The yield loss reaction was found to be terminated by the stopping reaction. The deprotonation of the 1,2-enol tautomer to the aldehyde leads to the formation of an anomeric enolate. The accompanying successive elimination of the hydroxide anion from the C-3 position is the key step in the stopping reaction. After a benzilic acid rearrangement, an alkali-stable metasaccharinic acid end group is formed (Machell and Richards 1957). Additionally to the benzilic rearrangement, the glycosidic bond could be cleaved in a further alkaline-induced peeling reaction.
The extent of undesired cellulose yield loss rises with an increasing reaction rate for peeling compared to stopping. Therefore, the ratio of both reaction rates is a crucial parameter.
In addition to temperature and alkali concentration, the type of applied base used is decisive for the extent of stabilisation. Alkaline earth metal hydroxides seem to accelerate the stable acid formation at the molecule end group, while divalent earth alkali hydroxides, in particular strontium hydroxide, lead to an increased formation of metasaccharinic acid with concomitant low yield losses (Colbran and Davidson 1961 ).
Naturally, the peeling reaction rate is proportional to the concentration of reducing end groups of the cellulose. Therefore, the yield loss shows a strong correlation with the degree of polymerization (DP) of the polysaccharide. This was verified by Johansson and Samuelson (1975).
Although many investigations on the kinetics of alkali-induced cellulose degradation were performed, no suitable data are available for the temperature and alkali concentration ranges occurring during steeping of dissolving pulp (Davidson 1934b; Entwistle et al. 1949; Haas et al. 1967; Glaus et al. 1999; Van Loon and Glaus 1997; Pavasars et al. 2003; Glaus and Van Loon 2008).
Therefore, the objective of this study was to develop a comprehensive kinetic model on the basis of extensive set of experiments describing the yield loss of cellulose under the conditions of pulp steeping, as a first step of viscose fiber manufacture.
Experimental
Materials and methods
A beech sulfite pulp was used for all the experiments. A TCF bleached mill sample was taken after final drying. The characteristics of the pulp are shown in Table 1.
The sodium hydroxide solution for steeping was prepared using pellets (p.a. grade, Merck) and adjusted to 18 ± 0.02 wt%. The pulp was placed in a round-bottom flask equipped with a magnetic stirrer and a thermometer. The sodium hydroxide solution was flushed with argon and adjusted to the required temperature levels of 40, 50 or 60 °C. The whole apparatus including the lye was then evacuated and flushed with argon several times before starting the experiment to eliminate the oxygen to minimize undesired side reactions. After the addition of the steeping lye the mixture was stirred vigorously for 10 min to ensure a complete mixing. A uniform impregnation of the fibres with sodium hydroxide was achieved. Xylan present in the pulp was dissolved to a great extent during this initial step. Therefore the starting point for the modelling of cellulose degradation kinetics was chosen after the initial steeping. The pulp suspension remained in the reactor for up to 80 h. All experiments were performed at least twice for each treatment time.
Subsequently, the suspension was filtered on a glass filter funnel (Porosity 4) and the solid residue was neutralized with 20 % acetic acid, washed until neutral pH and quantitatively transferred into a crystallizing dish, where it was air dried. The yield of the insoluble cellulose fraction was determined after the dry content analysis. To obtain the yield loss fraction, the amount of the insoluble material after the definite treatment time was related to the cellulose yield after the initial steeping time.
Intrinsic viscosity measurements of the insoluble cellulose fraction were performed after dissolution in cupriethylenediamine according to the SCAN-CM 15:99 method.
The determination of neutral sugar monomers was performed by anion exchange chromatography (AEC) with pulsed amperometric detection (PAD) after a two step total hydrolysis with H2SO4 according to Sixta et al. (2001).
The amount of carboxylic groups in the insoluble fraction was determined by the methylene blue adsorption method (Philipp et al. 1965; Whistler 1963). The uronic acid content of the initial pulp was determined by the FDAM-method (Bohrn et al. 2006).
The carbonyl group content was obtained after labelling with fluorescence marker and comparing with the SEC values (Röhrling et al. 2002).
Molecular weight distribution was determined by size exclusion chromatography (SEC) with multi-angle laser light scattering (MALLS) detection in DMAc-LiCl solution according to Schelosky et al. (1999).
For the SEM analysis the pulp samples were disintegrated in deionised water and freeze dried. Single fibres were fixed on a sample holder with an adhesive tape and subsequently sputtered with Au/Pd (Okamoto and Meshitsuka 2010). The prepared samples have been examined by high-resolution scanning electron microscopy at a 500× and 10,000× magnification with a Hitachi S4000 SEM (FE-SEM) applying an acceleration voltage of 8 kV.
Results and discussion
Development of a model for the kinetics of cellulose degradation under alkaline steeping conditions
Several studies concerning alkaline pulping describe carbohydrate degradation and the resulting low molecular weight products under the influence of temperatures around 150 °C and higher. Various carbohydrate containing materials formed similar compositions of the carboxylic acids under alkaline pulping conditions. Independent on alkali concentration and the characteristics of the substrate, mainly α- and β- glucoisosaccharinic acids were formed due to the peeling reaction. Formic, acetic, lactic and glycolic acids were identified as the most common further fragmentation products of the cellulose. In xylan containing sources, xyloisosaccharinic acid was also formed in a considerable amount. (Niemelä and Sjöström 1986; Sjöström 1977, 1991).
It has been shown that under the conditions of alkaline pulping polysaccharides, e.g. cellulose and hemicelluloses, are subjected to alkaline hydrolysis. Lai and Ontto revealed that the extent of the base-catalyzed cleavage of glycosidic linkages is generally negligible at 120 °C (Lai and Ontto 1979 ). Alkaline hydrolysis is mainly responsible for the depolymerisation of polysaccharides, but also for yield losses due to secondary peeling reactions which are triggered by the newly created reducing end groups (Franzon and Samuelson 1957; Nevell and Zeronian 1985).
Different assumptions have to be made for temperatures below pulping conditions. In this study, the behaviour of the main component cellulose under the particular conditions of alkaline steeping was of prevailing interest. Dissolving pulp used for the fibre production generally contains cellulose in amounts of >95 % odp. Especially, xylan in a hardwood dissolving pulp is removed to a content of below 1 % during alkaline steeping. Inevitable degradation reactions lead to an undesirable yield loss of the fibre forming material during industrial processing.
In alkaline media, three possible reaction types contributing to cellulose degradation can be distinguished.
1. The peeling off reaction (beta-elimination) of the reducing end group and their conversion to isosaccharinic acids is the most important contribution to yield loss during the steeping reaction (Fig. 1). The accessible reducing end group of the cellulose is transformed to an enediol via keto-enol tautomerism, which in the case of ketoses and aldoses is known as Lobry de Bruin-Alberda van Ekenstein transformation. Under alkaline conditions, the 2,3-enediol is deprotonated to an enolate, which promotes β-alkoxycarbonyl elimination at the C-4 carbon atom, followed by benzilic acid rearrangement to form α- or β-isosaccharinic (3-deoxy-2-C-(hydroxymethyl)-pentonic acid) acid. Retroaldol reactions of the enediol may lead to additional short chain degradation products (Lai 1996).
2. The second mechanism is the stopping reaction. The deprotonation of the 1,2-enediol causes β-hydroxycarbonyl elimination. After benzilic acid rearrangement, the formation of an alkali-stable glucometasaccharinic acid end group is observed (Machell and Richards 1957). The stopping or termination reaction was shown to occur only with alkali concentrations sufficiently high to produce the dianionic intermediate, whereas the peeling-off reaction is effective through both the mono- and the dianions (Young et al. 1972). Thus, the stopping reaction rate is more increased with rising alkali concentrations than the peeling reaction (Lai and Sarkanen 1969). The pathway of the reaction is illustrated in Fig. 1. Besides chemical stopping reactions, physical stopping occurs, when the end-wise degradation reaction reaches an inaccessible, crystalline region of the cellulose fibrils. Both, the chemical and physical stopping reactions account for the total chain termination reaction.
3. Alkaline hydrolysis is another type of cellulose degradation reaction. It usually does not occur at temperatures below 140 °C in a detectable scale. In contrast to the endwise peeling, this cellulose chain cleavage leads to a rapid decrease in DP. The mechanism of the hydrolysis was found to follow an internal nucleophilic substitution. After a deprotonation of the glucoside, the ionic intermediate is converted to degradation products. (Lai 1981). The random chain scission affords the formation of new reducing end groups accessible to secondary peeling, which further leads to an undesired yield loss.
For modelling cellulose degradation reactions in alkaline media, several assumptions were made concerning the significance of the mentioned reaction types. First attempts to describe the degradation kinetics were reported by Haas et al. (1967). A range of studies on the kinetics of degradation of cellulosic materials have recently been performed with regard to its behaviour under radioactive waste repository conditions (Van Loon and Glaus 1997; Van Loon et al. 1999). The kinetics of cellulose degradation in cement pore water at ambient temperature was calculated for time periods up to 12 years (Glaus and Van Loon 2008) using the kinetic model presented in a previous publication (Haas et al. 1967). Under the conditions of the pore water of a cementitious radioactive waste repository (pH of 13.3, room temperature) 80 % of the formed dissolved organic carbon were identified as α- and β-isosaccharinic acids (Glaus et al. 1999). In later studies a term representing the yield loss due to alkaline hydrolysis was added to the original equation (Pavasars et al. 2003).
In the early studies, the reaction rate constants for the peeling and stopping reactions of hydrocellulose were determined in 5 % aqueous sodium hydroxide solution at different temperatures. The overall yield loss was expressed by a simple equation denoted as Model I (Haas et al. 1967; Young et al. 1972):
where P is the mole fraction of peeled-off material, R 0 the mole fraction of the REGs at time 0, k p the first-order rate constant for the peeling reaction and k s is the first-order rate constant for the stopping reaction, including both chemical and physical stopping. This model neglects alkaline hydrolysis and secondary peeling reactions. Nevertheless, it was used in more recent publications to describe cellulose degradation in alkaline media, mainly in the context of radioactive waste repositories (Van Loon et al. 1999). In our study, a significant impact of alkaline hydrolysis was not expected, since the investigated temperature was in the range typical for the viscose processing of 40–60 °C.
Therefore, Eq. 1 was first used to obtain the rate constants. The initial end group mole fraction (R 0) of 2.6 × 10−3 (DPn = 407) was obtained from SEC measurements where a fluorescence marker (CCOA) was used to determine the REG concentration (Röhrling et al. 2002). The results of the application of this model on the obtained experimental data are shown in Fig. 2. The calculated reaction rate constants are listed in Table 2. A slight decrease of the determination coefficient reveals a decreasing agreement between the model and the experimental results with increasing temperature (40 °C: R² = 0.9952; 50 °C: R² = 0.9952; 60 °C: R² = 0.9890), suggesting that alkaline hydrolysis starts contributing to cellulose degradation. Thus, an additional calculation (Model II) was carried out using the equation proposed by (Van Loon and Glaus 1997; Pavasars et al. 2003):
where k h represents the rate constant of alkaline hydrolysis and D the overall carbohydrate yield loss. This equation, however, does not consider secondary peeling reactions initiated by the newly formed reducing end groups. Figure 3 compares the calculated cellulose yield losses, as a function of time at different temperatures using Eq. 2 (solid lines). The prediction based on Model II using the same experimental data from Fig. 2 (R² = 0.9942) is clearly superior to that from Model I (R² = 0.9908), in particular at higher temperature. The calculated rate constants for Eq. 2 are listed in Table 3.
Model II results in a suitable fitting for the experimental data. Nevertheless, a considerably lower impact of the alkaline hydrolysis on cellulose degradation was assumed in a later publication by Glaus and Van Loon (2004). The temperature range of the performed experiments seemed too low for a determination of hydrolysis reaction rate constant. A negligible occurrence of that reaction type at temperatures below 90 °C may be a possible explanation for the behavior.
None of the models described in the literature involved the amount of cellulose degraded due to secondary peeling after the formation of new reducing end groups initiated by alkaline hydrolysis.
An extended model (Model III) was developed to predict the degradation of cellulose during alkaline steeping in the viscose process. Therefore, a revision and adaptation of the existing models was required to allow exact predictions of the undesired cellulose yield loss. Model III was developed following the suggestions by Testova et al. including secondary peeling revealing that the yield loss caused by cellulose chain cleavage by hydrolysis is of minor importance (Testova et al. 2013).
The major contribution to the amount of degraded cellulose is caused by the endwise peeling reaction. The extent is dependent on the reducing end group concentration in the pulp and is expressed by the following equation:
where P stands for the material loss due to peeling-off and R represents the amount of reducing end groups. The amount of the latter is reduced due to the stopping reaction and, at the same time, increased due to alkaline hydrolysis.
In this equation Γ0 stands for the initial amount of the carbohydrates, while H represents the material degraded by hydrolysis, whose rate is proportional to the amount of non-degraded material.
The system of the last three differential equations was solved with the help of the DSolve function of the Wolfram Mathematica software setting the following initial conditions:
The determination of total degraded material D = P + H leads to a solution displayed in Eqs. (6)–(9).
with
For the fraction of degraded cellulose units expressed by \( P = \frac{D}{{\Upgamma_{0} }} \), the equation simplifies to:
To solve the system manually, a transformation of the system into one higher order differential equation is necessary. After deriving both sites of Eq. 4, the following expression is obtained:
Inserting Eqs. 4 and 5 into Eq. 11 leads to:
And after multiplying Eq. 3 with k h , addition to Eq. 12 and rearranging the terms gives:
leading to a second order homogeneous differential equation with constant coefficients with the characteristic solutions: −β ± a, with the notation of Eqs. 7, 8 and 9. Assuming that α is real and positive the solution of Eq. 13 is:
where C 1 and C 2 are integration constants. The received equation inserted into Eq. 3 and solved gives:
After inserting 14 and 15 into Eq. 4, H can be calculated:
Using the early mentioned initial conditions, the integration constants can be determined and after further calculations Eq. 16 is reached for the degraded material.
Evaluation of kinetic models for cellulose degradation during alkaline steeping
For comparison, all the described models were considered to verify their applicability for the data obtained in the experiments. All models consistently showed that alkaline peeling is responsible for the significant yield loss, resulting in the formation of soluble degradation products. In general, Models II and III describe the cellulose behavior under alkaline steeping conditions more precisely than Model I, especially at temperatures exceeding 50 °C (Figs. 2, 3).
The proportion of peeling versus stopping reactions characterizes the extent of the undesired cellulose degradation. Therefore, the ratio of the reaction constants kp/ks is a measure of the average degradable chain length and the stability of the carbohydrate substrate (Tables 2, 3, 4). The kp/ks ratios obtained by Model I are much higher than those of the other models, which points out the clear emphasis on the peeling reaction (Haas et al. 1967).
The amount of remaining cellulose was expressed as a function of time and temperature depending also on the activation energy and the frequency factor, the values of which were estimated by means of nonlinear regression to provide the best fit of the function to experimental data (Tables 5 and 6). For Model I the calculation resulted in values of the activation energy for both the peeling (98.7 kJ/mol), and stopping (93.9 kJ/mol) shown in Table 5. However, they compared well with the values of 101 and 97 kJ/mol for peeling and termination reactions of hydrocellulose reported in the literature for a significantly higher temperature range between 78 and 132 °C (Van Loon and Glaus 1997; Haas et al. 1967). As mentioned, Haas et al. did not consider alkaline hydrolysis in their model calculations. The kinetics of alkaline hydrolysis has been studied separately by Lai and Sarkanen (1967), as well as (Lai 1981) and Franzon and Samuelson (1957). Van Loon and Glaus (1997) and later Pavasars et al. (2003) were the first who modeled the full cellulose degradation under alkaline conditions combining peeling-off and base-catalyzed cleavage of glycosidic bonds. The application of their model denoted here as Model II on our experimental data resulted in significantly lower kp/ks ratios than obtained form Model I (Table 3). This can be explained by a substantial increase of the rate constants of termination compared to Model I.
Lai and Sarkanen (1969) and later Young et al. (1972) have shown that the stopping reaction occurs only with alkali concentrations sufficiently high to produce dianionic intermediate. Consequently, the stopping reaction rates are higher at high alkali concentration, as used in our study resulting in lower kp/ks ratios (Table 3). Although secondary peeling at the newly formed REGs is not considered by Model II, it provides a better prediction of the experimental data than Model I. As shown in Table 3, the reaction rates for the alkaline hydrolysis calculated by Model II are more than two orders of magnitude higher than those obtained by the first-order chain scission kinetics corresponding to alkaline hydrolysis (Eq. 17).
where k cs is the rate constant of the hydrolytic chain scission, DPv t is DPv at time t of alkaline degradation, DPv 0 is the starting DPv. The DP values were calculated based on the intrinsic viscosity of the pulp samples (SCAN-CM 15:88). Based on the DPv values of the regenerated, steeped pulp samples determined at 40 °C, a k cs value of 4.1 × 10−6 h−1 was calculated, which is much closer to the k h value of 46.3 × 10−6 h−1 obtained by Model III (Table 4) rather than a value of 1,190 × 10−6 h−1 received by Model II (Table 3). The rather low chain scission rate of the steeped pulp (Fig. 4) is accompanied by a significant narrowing of the molar mass distribution (MMD). As shown in Fig. 5 and Table 7 the PDI decreased from 5.7 after 0 h to about 3 after 80 h treatment time at 40 °C or 40 h at 60 °C. This can be attributed predominantly to the degradation of the long cellulose chains as a continuous decrease from 18 to 11 % was observed for the weight fraction of DP > 2,000, while the weight fraction of DP < 100 first decreased owing to dissolution and then stayed constant (Table 7).
The fraction of low molecular mass (DP < 100) in the starting pulp initially contained 3.1 % xylan and low molar mass cellulose. After preparation of the alkali cellulose and immediate stopping of the reactions (sample after 0 h) most of the hemicelluloses were already removed. Only 0.6 % xylan was attributing to the DP < 100 fraction and after prolonged reaction time only a minimum hemicellulose loss was observed. This means that any further loss of the low molar mass fragments due to longer reaction times can almost completely be attributed to cellulose degradation (Table 7). Even after long treatment times this fraction showed a negligible gain or remained unchanged (Fig. 5).
Furthermore, a rather slow decrease in intrinsic viscosity (Fig. 4) and Mw (Table 7) is an evidence for a slow degradation of the molecule chain length. This is a clear contrast to the contribution of the hydrolysis to cellulose degradation in Model II. From these observations, it can be summarized that a fitting of the experimental data by Model II leads to an overestimation of the alkaline hydrolysis reaction during steeping of dissolving pulp in the viscose process since secondary peeling at the newly formed REGs is neglected.
The formation of alkali-soluble degradation products accounts for the yield loss. In our study, they largely consisted of hydroxyacids, the major chemical compounds resulting form peeling. Especially, a high concentration of isosaccharinic acids was identified in the lye. This acid is well known as the main product of the peeling-off reaction. Additionally, the insoluble fraction after precipitation with sulphuric acid (β-cellulose) was very low. The soluble low Mw compounds (γ-cellulose) accounted for up to 92–95 % of the total organic fraction in the lye after an alkali treatment time of 63 h, which is in good accordance with the end-wise peeling mechanism. An increase of the β-cellulose fraction, corresponding to the frequent chain scission due to alkaline hydrolysis, was not observed. At no moment, xylo-oligomers resulting from alkaline hydrolysis had been identified in the liquor. It clearly confirms that both primary and secondary peeling reactions account for the majority of the cellulose yield loss. The composition and formation of the alkali soluble products will be discussed in detail in Part II.
The experimental observations of this study were fitted with the newly developed Model III. The reaction rates are listed in Table 4. The overall precision of both Models II and III are very similar (Fig. 3). Nevertheless, the rates of the individual reactions occurring under alkaline conditions are consistently explained by Model III. The overall peeling rates (kp) include the contribution of primary and secondary peeling and are thus higher than those obtained by Model I or II. The resulting ratios kp/ks are in the expected range for high alkali treatments (Paananen et al. 2010). This also applies to the very low alkaline hydrolysis reaction rates (Table 4) confirming the negligible contribution of alkali-induced random chain scission at low temperatures. However, the high alkali concentration during steeping accounts for higher alkaline hydrolysis reaction rates compared to those obtained at low concentrations (Lai 1981). The validity of Model III is also supported by the gradual decrease of the weight average molar mass (Mw) and the concomitant decrease in the weight fraction DP > 2,000. The activation energies for the peeling and stopping reactions obtained by Model III were in the expected range (Table 5). The Ea of 101.2 kJ/mol for the peeling reaction was exactly the same as reported by Van Loon and Glaus (1997) and Haas et al. (1967).
Simultaneously, the activation energy for the stopping reaction was slightly lower. However, the results for the Ea for the alkaline hydrolysis showed much lower values than reported in the literature. Lai (1981) reported activation energies for the base-catalyzed cleavage of glycosidic linkages at 6.3 M [OH−] using cellulose as a substrate of 84.5 and 76.7 kJ/mol, respectively. The value derived from this study of 24.7 kJ/mol was clearly too low. The typical temperature range for steeping between 40 and 60 °C was obviously too low and too narrow to obtain reliable results for the rate coefficients of alkaline hydrolysis. Nevertheless, the obtained values for the reaction rates were in a reasonable order of magnitude to account for the observed chain scission of the cellulose and the yield loss triggered by secondary peeling reactions.
Carbohydrate composition
The xylan content of 3.1 % in the starting pulp was reduced to 0.6–0.7 % through the treatment with steeping lye and the production of the alkali cellulose. After prolonged alkaline treatment, the value decreased to 0.5 % for all investigated samples (Table 8). Parallel to progressing cellulose degradation through peeling-off reaction, accessible xylan was solubilised.
The residual xylan seems to be resistant towards further alkaline degradation. Wollboldt et al. (2010) and Schild and Sixta (2011) correlated these alkali stable hemicelluloses with the impaired reactivity of dissolving pulps. One explanation for this could be the generation of xylan incorporated in cellulose chains during biosynthesis of the polymers in the growing tree. This could hinder the accessibility of the entrapped hemicelluloses during steeping, because crystalline regions do not take part in the degradation of polymers.
The relative composition of the insoluble cellulosic fraction remained unchanged with extended treatment time. The composition of alkali soluble components and degradation products will be discussed in a subsequent publication.
Carboxyl and carbonyl groups
The cellulose residue recovered after initial alkaline steeping contains only about 50 % of the carboxyl group content (COOHMB) in the initial dissolving pulp (Table 1 vs. Table 8). This decrease may be largely attributed to the removal of the hemicelluloses. The amount of carboxyl groups determined by the FDAM-method (COOHFDAM) is adequate to the uronic acid amount at the backbone of the molecule (Potthast et al. 2006). Metasaccharinic and other onic acids are not detected by this method. The COOHFDAM value of 13.5 μmol/g (Table 1) may for the most part be attributed to the alkali soluble xylan. Therefore, the results for the carboxyl groups of around 13 μmol/g substrate after the initial steeping are reasonable.
The entire further gain in acid end groups can be explained by the formation of metasaccharinic acid (Table 8). Figure 6 illustrates a substantial rise of the COOH content of cellulose with increasing temperature owing to an increase in the stopping reaction rate. A strong correlation of the metasaccharinic acid formation rate with the temperature is apparent.
An opposite tendency was displayed by the course of the carbonyl group (CO) content of the cellulose molecules as a function of time and temperature (Fig. 7). The CO concentration decreased with longer treatment time and increasing temperature due to rising rates of the termination reaction. The availability of the reducing end groups for the endwise elimination was decreasing with proceeding metasaccharinic acid formation. Due to the negligible reaction rate of alkaline hydrolysis, a minimal gain in concentration of the reducing end groups is accomplished. Hence the stopping reaction rate surpasses by far the chain cleavage velocity leading to a successive reduction of the carbonyl groups.
Fibre morphology
SEM images were performed to visualize changes in the fibre texture during alkaline steeping of the pulp. In the untreated sample fibril bundles liberated from the cell wall layer can be observed as a consequence of the previous bleaching and drying (Fig. 8a). In the course of the alkaline treatment the off-standing bundles are dissolved and the fibre surface becomes smooth (Fig. 8b, d). At the same time the lumen of the initially flat fibre cells swells due to the exposure to the sodium hydroxide solution. After extended reaction time again short fibre bundles have accumulated on the surface (Fig. 8c, e).
Application of the model to industrial steeping conditions
Alkaline steeping of the pulp is generally performed at 30–55 °C (Götze 1967). To achieve optimal alkali cellulose quality, a temperature of around 50 °C was reported by Mais and Sixta (2004) and Tatevosyan et al. (1965). The treatment time in industrial scale reaches up to 30 min for lower temperatures (Barthel and Philipp 1967; Tatevosyan et al. 1965). Assuming a residence time of 20 min at 50 °C, the cellulose yield loss after steeping calculated by the new model comes to 0.34 %. Considering the pulp used in this study (R 18 = 93.26 % odp) an undesired cellulose loss of 3.12 kg/t pulp occurs due to the peeling reactions. This adds to the intended purification loss of the pulp during steeping. A temperature increase of 5 °C leads to a loss of 0.59 %, whereas a steeping temperature of 45 °C decreases the degradation to 0.19 %. The reduction of the treatment time has a similar effect. After 10 min at 50 °C a minus of 0.17 % is expected, while after 30 min already 0.50 % of the cellulose is degraded.
Beside temperature and treatment time, the amount of the material yield loss is dependent on the initial concentration of the reducing end groups in the pulp. With lower DP, a higher amount of the REGs is present in the substrate leading to an accelerated degradation. A decrease of the DP by 20 % results in a 0.10 % higher cellulose loss.
Conclusions
A kinetic study was performed to describe the pulp yield loss during alkaline steeping in the viscose process. So far, models in the literature, only take primary peeling, stopping and alkaline hydrolysis into account. The newly introduced Model III explains all reaction mechanisms more comprehensibly than the previous models describing the kinetics of cellulose degradation in strong alkaline media. The contribution of secondary peeling is considered additionally. The rate constants reflect the extent of every single reaction type resulting in a good correlation with the experimental data.
The MMD and viscosity measurements confirmed that the peeling reaction is the predominant reaction type causing carbohydrate degradation and, thus, yield loss during alkaline treatment of cellulosic substrates. The activation energies for primary and secondary peeling as well as for the stopping reaction calculated with the new model are in the range of data earlier reported in the literature. Contrary to treatments of carbohydrates at low alkali concentrations as occurring under hot caustic extraction, the alkaline hydrolysis shows measureable reaction rates under steeping conditions, especially at temperatures above 50 °C. Due to the low temperature range during steeping, the determined Ea value for alkaline hydrolysis was not reliable. Therefore, the applicability of the model should be investigated for alkaline reactions at higher temperatures as used during alkaline pulping.
The observed increase of the carboxyl group content in the residual cellulose may be attributed to the formation of metasaccharinic acid caused by the stopping reaction, while at the same time a decrease of reducing end groups was confirmed.
The short chain material and loose fibrils were degraded more readily leaving a smooth fibre surface. After prolonged exposure to the alkali, short fibre bundles were again liberated from the surface.
Abbreviations
- BAR:
-
Benzilic acid rearrangement
- CCOA:
-
Carbazole-9-carbonyloxyamine
- D :
-
Overall carbohydrate yield loss
- DMAc:
-
N,N-Dimethylacetamide
- DP:
-
Degree of polymerisation
- FDAM:
-
9H-fluoren-2-yl-diazomethane
- FE-SEM:
-
Scanning electron microscope
- H :
-
Material degraded by hydrolysis
- HPLC:
-
High performance liquid chromatography
- ISA:
-
Isosaccharinic acid
- k h :
-
Rate constant of the alkaline hydrolysis
- k p :
-
Rate constant of the peeling reaction
- k s :
-
Rate constant of the stopping reaction
- MALLS:
-
Multi-angle laser light scattering
- MSA:
-
Metasaccharinic acid
- MB:
-
Methylene blue
- MMD:
-
Molar mass distribution
- Mn:
-
Number-average molecular weight
- Mw:
-
Weight-average molecular weight
- Odp:
-
Oven dried pulp
- P :
-
Mole fraction of peeled-off material
- PAD:
-
Pulsed amperometric detection
- PDI:
-
Polydispersity index
- R :
-
Amount of reducing end groups
- R 0 :
-
Initial reducing end group mole fraction
- REG:
-
Reducing end group
- SEC:
-
Size exclusion chromatography
- TCF:
-
Total chlorine free
- WRV:
-
Water retention value
- Γ0 :
-
Initial amount of the carbohydrates
- η:
-
Intrinsic viscosity
References
Barthel P, Philipp B (1967) Untersuchungen zum Abbauverlauf bei der Alkalicellulose-Vorreife verschiedenartiger Zellstoffe. Faserforschung und Textiltechnik 18(6):266–273 (537–538)
Bohrn R, Potthast A, Schiehser S, Rosenau T, Sixta H, Kosma P (2006) The FDAM method: determination of carboxyl profiles in dellulosic materials by combining group-selective fluorescence labeling with GPC. Biomacromolecules 7(6):1743–1750
Bywater N (2011) The global viscose fibre industry in the 21st century—the first 10 years. Lenzinger Berichte 89:22–29
Colbran RL, Davidson GF (1961) The degradative action of hot dilute alkalis on hydrocellulose. J Text Inst 52:T73–T87
Davidson GF (1934a) The dissolution of chemically modified cotton cellulose in alkaline solutions. Part I—in solutions of sodium hydroxide particularly at temperatures below the mormal. J Text Inst 25:T174–T196
Davidson GF (1934b) The dissolution of chemically modified cotton cellulose in alkaline solutions. Part I—in solutions of sodium hydroxide particularly at temperatures below the mormal. J Text Inst 25:T174–T196
Eichinger D (2012) A vision of the world of cellulosic fibers in 2020. Lenzinger Berichte 90:1–7
Entwistle D, Cole EH, Wooding NS (1949) The autoxidation of alkali cellulose. Part I: an experimental study of the kinetics of the reaction. Text Res J 19(9):527–546
Franzon O, Samuelson O (1957) Degradation of cellulose by alkali cooking. Sven Papperstidn 60(23):872–877
Glaus MA, Van Loon LR (2004) Technical report 03–08: cellulose degradation at alkaline conditions: long-term experiments at elevated temperatures, Hannover
Glaus MA, Van Loon LR (2008) Degradation of cellulose under alkaline conditions: new insights from a 12 years degradation study. Environ Sci Technol 42:2906–2911
Glaus MA, Van Loon LR, Achatz S, Chodura A, Fischer K (1999) Degradation of cellulosic materials under the alkaline conditions of a cementitious repository for low and intermediate level radioactive waste Part I: identification of degradation products. Anal Chim Acta 398:111–122
Götze K (1967) Chemiefasern nach dem Viskoseverfahren. Springer, Berlin
Haas DW, Hrutfiord BF, Sarkanen KV (1967) Kinetic study on the alkaline degradation of cotton hydrocellulose. J Appl Polym Sci 11:587
Hämmerle FM (2011) The cellulose gap (the future of cellulose fibres). Lenzinger Berichte 89:12–21
Johansson MH, Samuelson O (1975) End-wise degradation of hydrocellulose during hot alkali treatment. J Appl Polym Sci 19:3007–3013
Kolpak FJ, Weih M, Blackwell J (1978) Mercerization of cellulose: 1. Determination of the structure of mercerized cotton. Polymer 19(2):123–131
Krässig HA (1993) Cellulose: structure, accessibility and reactivity; methods of activation. Polymer monographs, vol 11. Gordon and Breach Science Publishers, Yverdon, Switzerland
Lai YZ (1981) In: International symposium on wood and pulping chemistry, Stockholm, The Ekman Days, vol 2. pp 26–33
Lai YZ (1996) Reactivity and accessibility of cellulose, hemicelluloses, and lignins. In: Hon DN-S (ed) Chemical modification of lignocellulosic materials. Marcel Dekker, New York, pp 35–95
Lai YZ, Ontto DE (1979) Effects of alkalinity on endwise depolymerisation of hydrocellulose. J Appl Polym Sci 23:3219–3225
Lai Y-Z, Sarkanen KV (1967) Kinetics of alkaline hydrolysis of glycosidic bonds in cotton cellulose. Cellul Chem Technol 1:517–527
Lai Y-Z, Sarkanen KV (1969) Kinetic study on the alkaline degradation of amylose. J Polym Sci C 28:15–26
Machell G, Richards GN (1957) The alkaline degradation of polysaccharides. Part II. The alkali-stable residue from the action of sodium hydroxide on cellulose. J Chem Soc 4500–4506
Mais U, Sixta H (2004) Characterization of alkali-soluble hemicelluloses of hardwood dissolving pulps. In: ACS Symposium Series, vol 864 (Hemicelluloses), pp 94–107
Nevell TP, Zeronian SH (eds) (1985) Cellulose chemistry and its applications. Ellis Horwood, Chichester
Niemelä K, Sjöström E (1986) The conversion of cellulose into carboxylic acids by a drastic alkali treatment. Biomass 11:215–221
Okamoto T, Meshitsuka G (2010) The nanostructure of kraft pulp 1: evaluation of various mild drying methods using field emission scanning electron microscopy. Cellulose 17:1171–1182
Paananen M, Tamminen T, Nieminen K, Sixta H (2010) Galactoglucomannan stabilization during the initial kraft cooking of scots pine. Holzforschung 64:683–692
Pavasars I, Hagberg J, Borén H, Allard B (2003) Alkaline degradation of cellulose: mechanisms and kinetics. J Polym Environ 11(2):1015–2636
Philipp B, Rehder W, Lang H (1965) Zur Carboxylbestimmung in Chemiezellstoffen. Das Papier 19(1):1–9
Potthast A, Rosenau T, Kosma P (2006) Analysis of oxidized functionalities in cellulose. Adv Polym Sci 205:1–48
Richards GN, Sephton HH (1957) The alkaline degradation of polysaccharides. Part I. Soluble products of the action of sodium hydroxide on cellulose. J Chem Soc 4492–4499
Richtzenhain H, Lindgren BO, Abrahamsson B, Holmberg K (1954) Über den alkalischen Abbau von Polysacchariden. I. Mitteil. Abbau von Baumwollhydrocellulose. Svensk Papperstidning 57(10):363–366
Röhrling J, Potthast A, Rosenau T, Sixta H, Kosma P (2002) Determination of carbonyl functions in cellulosic substrates. Lenzinger Berichte 81:89–97
Schelosky N, Röder T, Baldinger T, Milacher W, Morgenstern B, Sixta H (1999) Molecular mass distribution of cellulosic products by size exclusion chromatography in DMAc/LiCl. Das Papier 12(12):728–738
Schild G, Sixta H (2011) Sulfur-free dissolving pulps and their application for viscose and lyocell. Cellulose 18:1113–1128
Shen L, Patel MK (2010) Life cycle assessment of man-made cellulose fibres. Lenzinger Berichte 88:1–59
Sixta H, Schelosky N, Milacher W, Baldinger T, Röder T (2001) Characterization of alkali-soluble pulp fractions by chromatography. 11th ISWPC, vol 3. Nice, France
Sjöström E (1977) The behavior of wood polysaccharides during alkaline pulping processes. Tappi 60(9):151–157
Sjöström E (1991) Carbohydrate degradation products from alkaline treatment of biomass. Biomass Bioenergy 1(1):61–64
Tatevosyan EL, Makarova TP, Meos AI (1965) Characteristics of alkali cellulose obtained by a continuous process. Khim Volokna 4:26–29
Testova L, Nieminen K, Penttilä PA, Serimaa R, Potthast A, Sixta H (2013) Cellulose degradation in alkaline media upon acidic pretreatment and stabilisation. Carbohydr Polym. doi:10.1016/j.carbpol.2013.01.093
Van Loon LR, Glaus MA (1997) Review of the kinetics of alkaline degradation of cellulose in view of its relevance for safety assessment of radioactive waste repositories. J Environ Polym Degrad 5(2):97–109
Van Loon LR, Glaus MA, Laube A, Stallone S (1999) Degradation of cellulosic materials under the alkaline conditions of a cementitious repository for low- and intermediate-level radioactive waste. II. Degradation kinetics. J Environ Polym Degrad 7(1):41–51
Whistler RL (1963) Methods in carbohydrate chemistry, vol 3. Academic Press, New York
Wollboldt RP, Zuckerstätter G, Weber HK, Larsson PT, Sixta H (2010) Accessibility, reactivity and supramolecular structure of E. globulus pulps with reduced xylan content. Wood Sci Technol 44:533–546
Young RA, Sarkanen KV, Johnson G (1972) Marine plant polymers Part III. A kinetic analysis of the alkaline degradation of the polysaccharides with specific reference to (1→3)-beta-D-glucans. Carbohyd Res 21:111–122
Acknowledgments
Financial support was provided by the Austrian government, the provinces of lower Austria, upper Austria, and Carinthia as well as by Lenzing AG. We also express our gratitude to the Johannes Kepler University, Linz, the University of Natural Resources and Applied Life Sciences, Vienna, and Lenzing AG for their in-kind contributions
Author information
Authors and Affiliations
Corresponding author
Rights and permissions
About this article
Cite this article
Mozdyniewicz, D.J., Nieminen, K. & Sixta, H. Alkaline steeping of dissolving pulp. Part I: cellulose degradation kinetics. Cellulose 20, 1437–1451 (2013). https://doi.org/10.1007/s10570-013-9926-2
Received:
Accepted:
Published:
Issue Date:
DOI: https://doi.org/10.1007/s10570-013-9926-2