Abstract
An antifouling electrochemical aptasensor for ATP is described that has a zwitterionic self-assembled sensing interface on a glassy carbon electrode modified with a reduced graphene oxide carbon nanofiber (GO-CNF). The GO-CNF was first modified by self-polymerization of dopamine which provided a platform for simultaneously self-assembly of the ATP aptamer and cysteine. By using hexacyanoferrate as the electrochemical probe, in the presence of ATP, the aptamer strands fold around ATP molecules, thus leading to the variation of the electrochemical signal. The aptasensor has a linear response in the 0.1 pM to 5 nM ATP concentration range, and a 13 fM lower detection limit. The electrode is strongly resistant to nonspecific adsorption and biofouling. This enabled the detection of ATP even in spiked human plasma.

An antifouling electrochemical aptasensor employing reduced graphene oxide carbon nanofiber as conductive substrate and zwitterionic cysteine as antifouling material for adenosine triphosphate detection.
Similar content being viewed by others
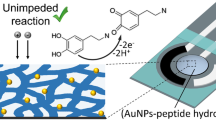
Explore related subjects
Discover the latest articles, news and stories from top researchers in related subjects.Avoid common mistakes on your manuscript.
Introduction
The progress in biomarkers identification offers opportunities for early disease diagnosis, especially for cancer, since the early diagnosis of cancer is dominating for its successful therapy. Therefore, it is urgent to explore high-performance means for biomarkers determination. To date, some new technologies consisting of fluorescence methods [1,2,3,4,5] enzyme-linked immunosorbent assay [6], surface enhanced Raman spectroscopy [7], surface plasmon resonance [8], etc. have been carried out for biomarkers analysis. Distinct from the above techniques, electrochemical means has attracted much attention due to its advantages of straightforward operation, high sensitivity, good selectivity, low cost, and rapid response. Electrochemical biosensors that based on aptamers, also known as electrochemical aptasensors, have provided wide applications in the disease biomarkers tracing. Thus, electrochemical aptasensors have become a hot research topic. Aptamers are artificially synthesized single-stranded oligonucleotides [9], with the ability to particularly bind with targets chosen in advance including small-sized nucleic acids, large-sized proteins, and even entire cells tightly [10]. At present, aptamers have been extensively applied as biorecognition segments in the fields of fabricating new type of electrochemical biosensors in consideration of their tremendous superiority such as cost-efficient, rapid production, easy labeling, high stability, and inherent selectivity. [11,12,13] Zhang and co-workers constructed an easily regenerated electrochemical aptasensor for sensitively detecting Adenosine Triphosphate (ATP) with satisfactory results [14]. On account of the selected specific aptamers, electrochemical aptasensors are inferred to play a vital role in early diagnosis of disease especially cancer and appraisal of treatment efficacy.
Although great progress has been made in the researches of electrochemical aptasensors, the practical clinical application of aptasensors still remains a monumental challenge due to serious nonspecific adsorptions and biofouling from complex biological media. Thus, the fabrication of antifouling electrochemical assay interface that can both lessen background interference and preserve sufficient target binding ability has been proved to be the most optimal goal in practical clinical application. To overcome this problem, many attempts have been conducted to devise antifouling assay interfaces based on antifouling materials [15]. Researches demonstrate that zwitterionic materials are ideal for antifouling applications [16]. Zwitterionic molecules are electroneutral materials which embody equivalent mole rate of positive and negative charges in close proximity [17]. The charged zwitterionic molecules possess enhanced hydration effect, which would accordingly promote its antifouling properties. Among various zwitterionic materials, cysteine (Cys) stands out as a prospective candidate. Cys is a natural zwitterionic compound in biosystem and owns intrinsically exceptional biocompatibility. With both carboxyl (–COOH) and amine (–NH2) groups, Cys has been confirmed to be capable of enhancing the antifouling performance and reducing protein adsorption [18, 19]. Shevate and others [20] have demonstrated that polystyrene-b-poly(4-vinylpyridine) isoporous membrane achieves intense water flux and antifouling ability after being coated with polydopamine (PDA) and grafted with Cys. Research carried out by Li et al. [21] points out that Cys grafted onto PDA coated poly(ethylene terephthalate) membranes improve the antifouling ability of the membranes and prevent HeLa cell adhesion. Besides its outstanding biocompatibility and comparable antifouling property, Cys is a low-cost, readily available material. However, few researches have been done taking advantage of Cys to prepare electrochemical biosensors. Hence, in this paper, Cys was adopted to construct antifouling assay interface of electrochemical aptasensor.
One current practice of preparing antifouling electrochemical aptasensor is taking advantage of bare gold electrode or glassy carbon electrode (GCE) as bioassay platforms. In view of previous report that nanomaterial-based electrochemical signal amplifications can profoundly improve the sensitivity and selectivity of electrochemical biosensors [22], we exploited reduced graphene oxide-carbon nanofiber (GO-CNF) modified GCE as bioassay platform for antifouling interface construction. GO-CNF, with its inherent merit, deserves adequate and wide attentions. Firstly, GO-CNF with uniform diameters and highly reticulate structures can be synthesized readily via scalable electrospinning technique and carbonizing at elevated temperatures. Secondly, GO-CNF with high specific surface area provides tremendous binding sites for the subsequent modification. Significantly, GO-CNF holds good electrical conductivity and possesses advantageous charge mobility, which is a significant qualification as the most desirable electrode substrates.
Another key issue for antifouling electrochemical aptasensor fabrication is the integration of antifouling materials and recognition aptamers on electrode surface. A feasible approach for antifouling materials and aptamers coating which has drawn considerable attention over the past few years is the utilizing of PDA. Inspired by the component of adhesive proteins in mussels, PDA which formed by dopamine (DA) self-polymerization under alkaline condition (pH 8.5) has been widely explored to manufacture multifunctional biocompatible inorganic and organic interfaces for varieties of prospective applications without complicated treatment process [23]. Another advantageous trait of PDA lies in the existence of numerous functional groups comprising catechol, quinone, amine, and imine, all these functional groups serve as the starting points for further modification with selected molecules. For example, materials contain thiol- and amino- functional groups can react with oxidized quinone form of catechol groups existed in PDA via Michael addition and Michael addition/Schiff base reaction, respectively [23]. (Supporting Information, Scheme S1) What’s more, PDA, synthetized by the natural neurotransmitter DA, retains superb biocompatibility and insignificant cytotoxicity [23]. With these virtues, PDA, inevitably, has been promptly merged into a broad range of applications comprising biological, chemical, materials, and medical sciences, as well as applied science engineering .
On account of the superiority of Cys, GO-CNF, and PDA that discussed above, in this research, we fabricated a uniquely aptasensor based upon mixed self-assembled aptamer and Cys on PDA coated GO-CNF modified GCE (GO-CNF/GCE). NH2-terminated ATP-specific aptamers and thiol- functional Cys employed in this research can covalent bond with PDA through the Michael addition reaction and Schiff base reaction. To the best of our knowledge, GO-CNF nanomaterials and Cys for the construction of antifouling electrochemical aptasensors have been rarely exploited. Adenosine triphosphate (ATP) was chose as the assay biomarker in this report since it is one of the most significant biomolecules in living creatures controlling diverse cellulate functions and processes. The ATP levels distinctly elevated in many malignant tumor cells because of the increased glycolysis [24]. GO-CNF was synthesized by a cost-efficient electrospinning and carbonizing process and applied as electrode substrates for aptasensor manufacture. After PDA coating, the PDA coated GO-CNF/GCE (PDA/GO-CNF/GCE) with high specific surface area provides tremendous binding sites for antifouling material Cys and ATP aptamer mixed self-assembly. Furthermore, through the mixed self-assembly manner, the antifouling interface not only maintains enough selective target binding ability due to the long-chained ATP-specific aptamers, but also holds the ability to resist nonspecific protein adsorption owing to the small molecules Cys coating. The high amounts of Cys and ATP aptamers immobilization can further decrease detection limits and enhance antifouling ability of the aptasensor. Additionally, Cys not only acts as antifouling material for aptasensor construction, it can also block the residual active sites of the PDA film. Thus, the aptasensor was able to detect human plasma with desirable results.
Experimental section
Reagents
Natural graphite (Qingdao Hengrui Industrial, www.hengruitai.com) was used in the GO synthesis procedure. Polyacrylonitrile (PAN, MW = 150,000) powder, N, N′-Dimethylformamide (DMF, AR) (Sigma-Aldrich, www.sigmaaldrich.com) were employed in the synthesis of GO-CNF. ATP, cytosine triphosphate (CTP), uridine triphosphate (UTP), guanosine triphosphate (GTP), adenosine monophosphate (AMP), adenosine diphosphate (ADP) (Yuanye Bio-Technology Co. www.shyuanye.com), DA, cysteine (Cys), lysozyme (Lys), bovine serum albumin (BSA) (Sigma-Aldrich, www.sigmaaldrich.com) were used in the electrochemistry study. The ATP binding aptamer was [5’-NH2-(CH2)6-ACC TGG GGG AGT ATT GCG GAG GAA GGT] (Sangon Biotech Co., Ltd. www.sangon.com) with the affinity binding interactions (dissociation constants, Kd = 31 ± 3 μM) [25]. All chemicals were of analytical grade and doubly distilled water was used throughout the research. The human plasma was provided by The First Hospital of Jilin University (www.jdyy.cn). Experiments were conducted at room temperature.
Apparatus
Scanning electron microscopy (SEM) measurements were obtained by adopting of JEOL JSM 6700F (www.jeol.co.jp) scanning electron microscope operated at 5 kV acceleration voltage. Fourier transform infrared spectroscopy (FTIR) images were received with a Nicolet Impact 410 FTIR spectrometer (www.thermofisher.com). X-ray photoelectron spectroscopy (XPS) measurements were carried out on an ESCALAB-MKII 250 X-ray photoelectron spectrometer (www.thermofisher.com) adopting Al Kα radiation. The water contact angle was surveyed on a JC2000 contact angle goniometer (Zhongchen Digital Technical Co., www.meterstar.com) via sessile drop method at ambient temperature and humidity. Electrochemical measurements were executed on a CHI 920C electrochemical workstation (www.chinstr.com) with a bare glassy carbon electrode (GCE, 3 mm in diameter) as working electrode, a saturated calomel electrode (SCE) as reference electrode, and a platinum wire as counter electrode. All potentials were referenced to SCE.
Synthesis of GO and GO-CNF
GO was synthesized using the natural graphite with reference to modified Hummer’s method [26]. The successful synthesis of GO was verified in the Supporting Information. GO-CNF was produced via a typical procedure listed below: first, 1.00 g PAN and 0.10 g GO were dispersed uniformly in 10 mL DMF with rapid stirring for 12 h. Then, the mixture was loaded into a glass capillary with the tip immobilized about 20 cm away from an aluminum foil collector, which was connected to a high-voltage power supply that was maintained at 18 kV. The electrospinning process lasted for about 12 h. Next, the electrospun grey textiles (GO–PAN) were placed into a vacuum desiccator and dried overnight. Finally, the fabrics GO–PAN was stabilized in a tube furnace at 240 °C for 2 h under air with a heating rate of 1.0 °C min−1, and then the textiles was heated to 500 °C under N2 atmosphere at 2.0 °C min−1, subsequently carbonized at 900 °C for 1 h with a heating rate of 3.0 °C min−1. The grey textiles GO–PAN was transformed into the black GO-CNF.
Preparation of the electrochemical aptasensor
The aptasensor was produced as illustrated in Scheme 1. Prior to using, GCE was polished with 0.3 and 0.05 μm alumina powder, and then sonicated with nitric acid/water (1/1, v/v), absolute ethanol, and water for 3 min in sequence. Later, the cleaned GCE was dried by nitrogen steam and 10 μL of GO-CNF suspension (1.0 mg mL−1) was uniformly cast onto the polished GCE surface and dried in the air at ambient temperature. The GO-CNF/GCE was then submerged in the DA solution (2 mg mL−1) which was dissolved by Tris buffer (10 mM, pH 8.5) for 80 min for PDA anchoring, afterwards, the electrode was sufficiently rinsed with water to remove unpolymerized DA and dried in the air. Then, 10 μL of 2 μM aptamer (10 mM Tris buffer, pH 7.4) and 5 μL of 5 mg mL−1 Cys (10 mM Tris buffer, pH 7.4) were simultaneously dropped onto PDA/GO-CNF/GCE surface and incubated overnight at room temperature. (Optimization of the Cys concentrations was depicted in the Supporting Information) The electrode was sealed in a beaker with a little water at bottom to avoid solution evaporating. After incubation, the Apt-Cys/PDA/GO-CNF/GCE was completely rinsed using PBS (10 mM, pH 7.4) to remove weakly bound aptamers and Cys.
Electrochemical impedance spectroscopy (EIS) measurements
EIS tests was carried out to observe the process of aptasensor fabrication since it was able to transfer intricate bio-recognition activities occurred at electrode surface into intuitionistic electrical signals. EIS measurements were conducted in PBS (10 mM, pH 7.4) containing 0.1 M KCl and 5 mM K3[Fe(CN)6]/K4[Fe(CN)6]. The Nyquist plot of EIS was used, which consists of a semicircle portion (its semicircle diameter represents the charge transfer resistance) and a linear portion (which associated with the diffusion limited process at the electrode interface) separately at higher and lower frequencies. The direct current potential was set at 0.20 V and frequency range was from 105 to 0.01 Hz with the amplitude of 5 mV.
Results discussion
Characterization of the electrochemical Aptasensor
To confirm the feasibility of the electrospinning method, SEM tests were carried out to investigate the morphology of the GO–PAN and GO–CNF. Figure 1a shows SEM image of electrospun nanofibers GO–PAN, as we can see, the fabricated GO–PAN nanofibers are comparatively uniform with smooth surface. After carbonized at 900 °C, displayed as Fig. 1b, c and d, the diameter of the nanofibers dramatically decreases from 300 ± 50 nm to 70 ± 20 nm. And the surface of GO–CNF is relatively rough with some nanoscale lamellar structure, which is ascribed to the loss of PAN at elevated temperature and the introduction of GO into the nanofibers. After PDA anchoring (Fig. 1e), GO–CNF still maintains the primary nanofibrous structure. Thus, the GO–CNF with uniform diameters, freestanding structure, and high specific surface area make it a desirable electrode modified material for aptasensor construction.
XPS was employed to characterize the composition of different electrode materials after each modification steps. As exhibited in Fig. 1f, the XPS survey spectrum of GO–PAN exhibits three predominant peaks associated with C 1 s (285 eV), N 1 s (400 eV), and O 1 s (533 eV) electrons [27]. After calcination, the peak intensity of N 1 s evidently decreases in GO–CNF due to the release of N2 at high temperature, which is in accord with previous report [28]. In addition, the notable decrease of the O 1 s peak of GO–CNF in contrast with GO–PAN proves the successful reduction of GO during calcination. After being submerged in the DA solution, the N 1 s and O 1 s peak intensities enhance distinctly compared with that of GO–CNF, providing an attestation that GO–CNF modified electrode surface is favorably coated with a PDA film. With the simultaneous immobilization of aptamer and Cys, two relatively minor characteristic peaks appear including P 2p at 134 eV and S 2p at 164 eV [29]. As discussed above, NH2-terminated ATP-specific aptamers and Cys can covalent bond with PDA through the Michael addition reaction and Schiff base reaction. The P 2p and S 2p signal peaks separately originate in the surface-immobilized aptamer and Cys, indicating the successful construction of the electrochemical aptasensor.
The surface hydrophilic property of the different materials was evaluated by static water contact angle method assessment. As presented in Fig. 2, the contact angle value of GO–CNF coated silicon wafer is 38.2°, indicating the hydrophilic property of synthetized GO–CNF. After PDA modification, the water contact angle value decreases slightly to 35.1°, which is attributed to the introduction of the hydrophilic groups including carboxyl, amino, hydroxyl etc. As expected, aptamer and Cys modified interface displays advanced hydrophilic property with a much lower contact angle value of 22.3°. The superhydrophilicity of Apt-Cys/PDA/GO-CNF coated interface is due to the uniquely zwitterionic structure of Cys with alternate amino and carboxyl groups. Consequently, Apt-Cys/PDA/GO-CNF with high hydrophilicity is capable of repealing nonspecific protein adsorption in bioanalysis and biomedical detection.
Electrochemical characterization of the fabrication process of Aptasensor
The construction process of the aptasensor was observed via EIS and cyclic voltammograms (CV). The charge transfer resistance (Rct) of different modified electrodes were listed in Table S1. GO-CNF/GCE shows the smallest Rct, which means the GO-CNF has prominent electron transfer performance. This phenomenon is in accordance with what we have described above that GO-CNF holds good electrical conductivity. After PDA coating, the Rct of PDA/GO-CNF/GCE dramatically increases attributing to the poor electrical conductivity of PDA. When the Cys and aptamer are self-assembled on electrode surface, the Apt-Cys/PDA/GO-CNF/GCE demonstrates much higher Rct. The possible causes are that aptamer embodies negatively charged phosphate skeletons which can repulse negative [Fe(CN)6]3−/4− probe, leading to higher Rct than PDA/GO-CNF/GCE. It is noteworthy that the Rct of Apt-Cys/PDA/GO-CNF/GCE after ATP incubation (aptamer specifically bound to the ATP) increases remarkably, which can just be utilized as the signal for ATP detection. The sound reasons lie in the fact that ATP carries negative phosphate groups, and the binding of ATP to the electrode surface can further repel negatively charged [Fe(CN)6]3−/4− probe and raise the Rct of the aptasensor. The results of CV (Fig. 3b) which are consistent with EIS results further confirm the successful manufacture of aptasensor.
Impedance Nyquist plots of the EIS (a) and CV curves (b) obtained at different modified electrodes: a. GO-CNF/GCE; b. GCE; c. PDA/GO-CNF/GCE; d. Apt-Cys/PDA/GO-CNF/GCE; and e. ATP/Apt-Cys/PDA/GO-CNF/GCE. Measurements were performed in PBS (10 mM, pH 7.4) containing 5.0 mM [Fe(CN)6]3−/4− and 0.1 M KCl. The concentration of ATP was 0.01 μM (10 mM PBS, pH 7.4). Inset in (a): EIS obtained at the a. GO-CNF/GCE and b. GCE, respectively
Optimization of the ATP incubation time
Incubation time is a significant experiment condition parameter for ATP aptasensor to realize the highest sensitivity and the most efficient determination. Thus the incubation time is optimized via EIS tests and showed in Fig. 4. It can be obviously noticed that Rct changes [ΔRct/Rct0 (%)] increase gradually with time varying from 0.5 h to 1.5 h, and then hardly grow from 1 .5h to 2.5 h. Consequently, 1.5 h is chose as the optimal incubation time for ATP detection. Here, Rct is the impedance value of the aptasensor measured after incubated with ATP or other objects, Rct0 indicates the impedance value of the aptasensor without incubation, and ΔRct = Rct - Rct0, which represents the impedance value changes.
Determination of ATP via EIS measurements
EIS measurements were utilized to evaluate the electrochemical assay performance of the fabricated aptasensor for label-free ATP tracing. As displayed in Fig. 5, the [ΔRct/Rct0 (%)] value of the aptasensor increases along with the increasing ATP concentrations, and the [ΔRct/Rct0 (%)] is in good linear relationship with the logarithm of ATP concentrations ranging from 0.1 to 5000 pM with a low detection limit of 0.013 pM (S/N = 3). The linear regression equation is ΔRct/Rct0 (%) = 25.17 logC (pM) + 51.92 (R2 = 0.9958). The detection limit is much lower than that of most previous researches (Table S2). This probably because the high specific surface area structure of the PDA coated GO-CNF which supplies tremendous binding sites for antifouling material Cys and ATP aptamer mixed self-assembly. Besides, through the mixed self-assembly manner, the antifouling interface not only maintains enough selective target binding ability due to the long-chained ATP-specific aptamers, but also holds the ability to resist nonspecific protein adsorption owing to the small molecules Cys. Furthermore, PDA and Cys can create a favorable environment for aptamer to maintain high bioactivity and binding affinity, which leads to low detection limit and strong anti-pollution traits of the aptasensor. Since the clinically meaningful concentration of ATP in serum ranges from 0.1 to 3 mM [30, 31], the sensitivity of the proposed method is satisfied for practical application.
(a) Typical EIS related to the aptasensor incubated with varying ATP concentrations (PBS, 10 mM, pH 7.4): plots from inner to outer represent 0, 0.1, 1, 10, 100, 1000 and 5000 pM ATP, respectively. Measurements were performed in PBS (10 mM, pH 7.4) containing 5.0 mM [Fe(CN)6]3−/4− and 0.1 M KCl. (b) Rct changes [ΔRct/Rct0 (%)] of the ATP aptasensor upon different ATP concentrations. Inset: the corresponding calibration plot of Rct changes versus the logarithm of ATP concentrations
Selectivity, reproducibility, stability and antifouling property of the Aptasensor
To explore selectivity of the aptasensor for the electrochemical detection of ATP, Rct changes were examined in different solutions containing CTP (1.0 nM), UTP (1.0 nM), GTP (1.0 nM), AMP (100 pM), ADP (100 pM), and ATP (100 pM). As shown in Fig. 6, in contrast with Rct change caused by ATP, Rct changes of the aptasensor observed for CTP, UTP, GTP, AMP, and ADP detecting is negligible, demonstrating the fabricated aptasensor has beneficial specificity for ATP determination. This result is in accordance with previous reports concerning ATP detection [32, 33]. The superb selectivity of aptasensor is ascribed to the inherent characteristics of aptamers. They can bind with their objective molecules with high specificity and affinity via folding into different secondary and tertiary structures [34]. Our research results further testify the inherent selectivity of aptamers, which make it a research focus in electrochemical biosensors construction.
The reproducibility of the ATP aptasensor was evaluated by EIS measurements. The results were displayed in Fig. S3. The relative standard derivation (RSD) of Rct changes conducted by ten different electrodes prepared under the same experimental conditions for detecting 1.0 pM ATP is 6.11%. It means that the reproducibility of the ATP aptasensor is acceptable.
In order to evaluate whether the aptasensor can satisfy the requirement of long term stability, the same modified GCE was stored at 4 °C and measured everyday for 10 days. As displayed in Fig. S4, the aptasensor maintained 86% of original response after being kept for 10 days. This revealed the satisfactory stability of the aptasensor.
The antifouling property is an important factor to assess the application prospects of aptasensor. To evaluate the antifouling performance of the fabricated aptasensor, Rct changes of the ATP aptasensor with and without antifouling Cys coating were separately recorded after incubating in single protein solution (charged positively Lys, and charged negatively BSA) and human plasma. As depicted in Fig. S5 and Fig. S6, the ATP aptasensor modified with Cys displays distinguished smaller Rct changes in contrast with the aptasensor without Cys coating both in single protein solutions and in human plasma. Compared with the reported antifouling ATP electrochemical aptasensor based on PEG and zwitterionic peptide [27, 35], the fouling resistance performance of our recommended aptasensor is inferior, but it is strong enough to enable the aptasensor to detect ATP in human plasma. In consideration of the intrinsic favorable properties including comparable antifouling capability, outstanding biocompatibility, low cost, and readily available of Cys, the suggested aptasensor Apt-Cys/PDA/GO-CNF/GCE has broad prospect of practical application in ATP tracing.
Clinical application of the Aptasensor
To examine the practical application feasibility of the aptasensor to detect ATP in biological environment, standard addition method was employed to detect ATP in 1% (V/V) human plasma samples. Different concentrations of ATP are uniformly dispersed into 1% human plasma and utilized for determination. The outcome of the experiment is listed in Table 1. As illustrated in Table 1, the fabricated aptasensor exhibits satisfactory recovery ranging from 96.9 to 104%, which means the research results are nearly equal to their real values. In summary, the constructed ATP aptasensor exhibits huge application prospect in tracing ATP in physiological samples for bioanalysis and biomedical detection.
Conclusion
In this report, a new type ultrasensitive low-fouling aptasensor based on PDA/GO-CNF was described, choosing ATP as the detecting target, zwitterionic Cys as the antifouling material. Compared with other conventional zwitterionic materials, Cys holds outstanding biocompatibility and comparable antifouling property, and it is low-cost and readily available. ATP aptamers and Cys were effectively self-assembled on PDA/GO-CNF via Michael addition or Schiff base reaction under mild conditions. In the fabricated assay interface, nanomaterial GO-CNF with good electrical conductivity and high specific surface area is employed as signal amplification matrixes, which provids tremendous binding sites for Cys and ATP aptamers anchoring. Furthermore, via the mixed self-assembly means, the antifouling interface can not only decrease background interference, but also preserve sufficient target binding ability, thus further decreases detection limits and enhances antifouling property of the aptasensor. The suggested aptasensor can be employed to detect ATP in human plasma with acceptable accuracy and none serious nonspecific adsorptions. This results indicate that the aptasensor possesses great potential application prospect in biomarkers determination for disease especially cancer early diagnosis and treatment efficacy appraisal. Furthermore, PDA/GO-CNF paves the way for other novel sensitive low-fouling aptasensors construction to detect multiple types of biomarkers in bioanalysis and biomedical detection.
References
Deng J, Wang K, Wang M, Yu P, Mao L (2017) Mitochondria targeted nanoscale Zeolitic imidazole Framework-90 for ATP imaging in live cells. J Am Chem Soc 139:5877–5882
Qu F, Sun C, Lv X, You J (2018) A terbium-based metal-organic framework@gold nanoparticle system as a fluorometric probe for aptamer based determination of adenosine triphosphate. Microchim Acta 185:359
Liu X, Lin B, Yu Y, Cao Y, Guo M (2018) A multifunctional probe based on the use of labeled aptamer and magnetic nanoparticles for fluorometric determination of adenosine 5 '-triphosphate. Microchim Acta 185
Cheng X, Cen Y, Xu G, Wei F, Shi M, Xu X, Sohail M, Hu Q (2018) Aptamer based fluorometric determination of ATP by exploiting the FRET between carbon dots and graphene oxide. Microchim Acta 185:144
El Kurdi R, Patra D (2018) Nanosensing of ATP by fluorescence recovery after surface energy transfer between rhodamine B and curcubit 7 uril-capped gold nanoparticles. Microchim Acta 185:349
Alberti D, van't Erve M, Stefania R, Ruggiero MR, Tapparo M, Geninatti Crich S, Aime S (2014) A quantitative Relaxometric version of the ELISA test for the measurement of cell surface biomarkers. Angew Chem Int Ed 53:3488–3491
Sun F, Ella-Menye J-R, Galvan DD, Bai T, Hung H-C, Chou Y-N, Zhang P, Jiang S, Yu Q (2015) Stealth surface modification of surface-enhanced Raman scattering substrates for sensitive and accurate detection in protein solutions. ACS Nano 9:2668–2676
He L, Pagneux Q, Larroulet I, Serrano AY, Pesquera A, Zurutuza A, Mandler D, Boukherroub R, Szunerits S (2017) Label-free femtomolar cancer biomarker detection in human serum using graphene-coated surface plasmon resonance chips. Biosens Bioelectron 89:606–611
Tuerk C, Gold L (1990) Systematic evolution of ligands by exponential enrichment: RNA ligands to bacteriophage T4 DNA polymerase. Science 249:505–510
Bozokalfa G, Akbulut H, Demir B, Guler E, Gumus ZP, Odaci Demirkol D, Aldemir E, Yamada S, Endo T, Coskunol H, Timur S, Yagci Y (2016) Polypeptide functional surface for the aptamer immobilization: electrochemical cocaine biosensing. Anal Chem 88:4161–4167
Park KS (2018) Nucleic acid aptamer-based methods for diagnosis of infections. Biosens Bioelectron 102:179–188
Rapini R, Marrazza G (2017) Electrochemical aptasensors for contaminants detection in food and environment: recent advances. Bioelectrochemistry 118:47–61
Farzin L, Shamsipur M, Samandari L, Sheibani S (2018) Advances in the design of nanomaterial-based electrochemical affinity and enzymatic biosensors for metabolic biomarkers: a review. Microchim Acta 185:276
Zhang X, Song C, Yang K, Hong W, Lu Y, Yu P, Mao L (2018) Photoinduced regeneration of an aptamer-based electrochemical sensor for sensitively detecting adenosine triphosphate. Anal Chem 90:4968–4971
Nowinski AK, Sun F, White AD, Keefe AJ, Jiang S (2012) Sequence, structure, and function of peptide self-assembled monolayers. J Am Chem Soc 134:6000–6005
Schlenoff JB (2014) Zwitteration: coating surfaces with Zwitterionic functionality to reduce nonspecific adsorption. Langmuir 30:9625–9636
Shih Y-J, Chang Y, Quemener D, Yang H-S, Jhong J-F, Ho F-M, Higuchi A, Chang Y (2014) Hemocompatibility of Polyampholyte copolymers with well-defined charge Bias in human blood. Langmuir 30:6489–6496
Wang P, Yang J, Zhou B, Hu Y, Xing L, Xu F, Shen M, Zhang G, Shi X (2017) Antifouling manganese oxide nanoparticles: synthesis, characterization, and applications for enhanced MR imaging of tumors. ACS Appl Mater Interfaces 9:47–53
Lin P, Chuang T-L, Chen PZ, Lin C-W, Gu FX (2019) Low-fouling characteristics of ultrathin Zwitterionic cysteine SAMs. Langmuir 35:1756–1767
Shevate R, Kumar M, Karunakaran M, Hedhili MN, Peinemann K-V (2017) Polydopamine/cysteine surface modified isoporous membranes with self-cleaning properties. J Membr Sci 529:185–194
Li P, Cai X, Wang D, Chen S, Yuan J, Li L, Shen J (2013) Hemocompatibility and anti-biofouling property improvement of poly(ethylene terephthalate) via self-polymerization of dopamine and covalent graft of zwitterionic cysteine. Colloids Surf B: Biointerfaces 110:327–332
Zhu C, Yang G, Li H, Du D, Lin Y (2015) Electrochemical sensors and biosensors based on nanomaterials and nanostructures. Anal Chem 87:230–249
Liu Y, Ai K, Lu L (2014) Polydopamine and its derivative materials: synthesis and promising applications in energy, environmental, and biomedical fields. Chem Rev 114:5057–5115
Mo R, Jiang T, DiSanto R, Tai W, Gu Z (2014) ATP-triggered anticancer drug delivery. Nat Commun 5:3364
Biniuri Y, Albada B, Willner I (2018) Probing ATP/ATP-aptamer or ATP-aptamer mutant complexes by microscale thermophoresis and molecular dynamics simulations: discovery of an ATP-aptamer sequence of superior binding properties. J Phys Chem B 122:9102–9109
Li D, Muller MB, Gilje S, Kaner RB, Wallace GG (2008) Processable aqueous dispersions of graphene nanosheets. Nat Nanotechnol 3:101–105
Wang G, Xu Q, Liu L, Su X, Lin J, Xu G, Luo X (2017) Mixed self-assembly of polyethylene glycol and aptamer on Polydopamine surface for highly sensitive and low-fouling detection of adenosine triphosphate in complex media. ACS Appl Mater Interfaces 9:31153–31160
Wen Y-F, Cao X, Yang Y-G, Li H, Guo J-Q, Liu L (2008) Carbonization of pre-oxidized polyacrylonitrile fibers. Carbon 46:2
Wang G, Han R, Su X, Li Y, Xu G, Luo X (2017) Zwitterionic peptide anchored to conducting polymer PEDOT for the development of antifouling and ultrasensitive electrochemical DNA sensor. Biosens Bioelectron 92:396–401
Labib M, Sargent EH, Kelley SO (2016) Electrochemical methods for the analysis of clinically relevant biomolecules. Chem Rev 116:9001–9090
Llaudet E, Hatz S, Droniou M, Dale N (2005) Microelectrode biosensor for real-time measurement of ATP in biological tissue. Anal Chem 77:3267–3273
Lu L, Si JC, Gao ZF, Zhang Y, Lei JL, Luo HQ, Li NB (2015) Highly selective and sensitive electrochemical biosensor for ATP based on the dual strategy integrating the cofactor-dependent enzymatic ligation reaction with self-cleaving DNAzyme-amplified electrochemical detection. Biosens Bioelectron 63:14–20
Lu L-M, Zhang X-B, Kong R-M, Yang B, Tan W (2011) A ligation-triggered DNAzyme Cascade for amplified fluorescence detection of biological small molecules with zero-background signal. J Am Chem Soc 133:11686–11691
Iliuk AB, Hu L, Tao WA (2011) Aptamer in bioanalytical applications. Anal Chem 83:4440–4452
Wang G, Su X, Xu Q, Xu G, Lin J, Luo X (2018) Antifouling aptasensor for the detection of adenosine triphosphate in biological media based on mixed self-assembled aptamer and zwitterionic peptide. Biosens Bioelectron 101:129–134
Acknowledgements
This work acknowledges support from the National Natural Science Foundation of China (No. 21375045) and Natural Science Foundation of Jilin Province (No. 20180101195JC).
Author information
Authors and Affiliations
Corresponding authors
Ethics declarations
The author(s) declare that they have no competing interests.
Additional information
Publisher’s note
Springer Nature remains neutral with regard to jurisdictional claims in published maps and institutional affiliations.
Electronic supplementary material
ESM 1
(DOCX 2.44 mb)
Rights and permissions
About this article
Cite this article
Zhang, T., Xu, H., Xu, Z. et al. A bioinspired antifouling zwitterionic interface based on reduced graphene oxide carbon nanofibers: electrochemical aptasensing of adenosine triphosphate. Microchim Acta 186, 240 (2019). https://doi.org/10.1007/s00604-019-3343-7
Received:
Accepted:
Published:
DOI: https://doi.org/10.1007/s00604-019-3343-7