Abstract
Surface litter decomposition in arid and semiarid ecosystems is often faster than predicted by climatic parameters such as annual precipitation or evapotranspiration, or based on standard indices of litter quality such as lignin or nitrogen concentrations. Abiotic photodegradation has been demonstrated to be an important factor controlling aboveground litter decomposition in aridland ecosystems, but soil fauna, particularly macrofauna such as termites and ants, have also been identified as key players affecting litter mass loss in warm deserts. Our objective was to quantify the importance of soil organisms on surface litter decomposition in the Patagonian steppe in the absence of photodegradative effects, to establish the relative importance of soil organisms on rates of mass loss and nitrogen release. We estimated the relative contribution of soil fauna and microbes to litter decomposition of a dominant grass using litterboxes with variable mesh sizes that excluded groups of soil fauna based on size class (10, 2, and 0.01 mm), which were placed beneath shrub canopies. We also employed chemical repellents (naphthalene and fungicide). The exclusion of macro- and mesofauna had no effect on litter mass loss over 3 years (P = 0.36), as litter decomposition was similar in all soil fauna exclusions and naphthalene-treated litter. In contrast, reduction of fungal activity significantly inhibited litter decomposition (P < 0.001). Although soil fauna have been mentioned as a key control of litter decomposition in warm deserts, biogeographic legacies and temperature limitation may constrain the importance of these organisms in temperate aridlands, particularly in the southern hemisphere.
Similar content being viewed by others
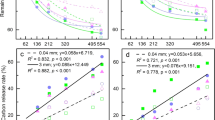
Explore related subjects
Discover the latest articles, news and stories from top researchers in related subjects.Avoid common mistakes on your manuscript.
Introduction
Plant litter decomposition is a key process in terrestrial ecosystems, determining carbon turnover, as well as the rate and timing of nutrient release. While climate and litter chemistry have been shown to be some of the most important factors controlling litter decomposition in terrestrial ecosystem at the regional scale (Meentemeyer 1978; Vitousek et al. 1994; Coûteaux et al. 1995; Austin and Vitousek 2000; Gholz et al. 2000; Cornwell et al. 2008; Bontti et al. 2009), soil organisms (soil fauna and microbes) represent a key biotic component affecting soil organic matter formation and nutrient release locally (Swift et al. 1979; Coûteaux et al. 1995; Aerts 1997). Soil fauna have body sizes large enough to disrupt physical structure of soil and litter and can affect organic matter decomposition directly through fractionation and consumption of litter (Coleman and Crossley 1996). In addition, they can affect decomposition indirectly through changes in microclimate conditions such as soil moisture and litter surface area, regulating microbial activities during litter decomposition (Bradford et al. 2002).
Surface leaf litter decomposition in many arid and semiarid ecosystems has shown little correlation with climatic parameters such as precipitation and evapotranspiration (Whitford et al. 1981; Steinberger et al. 1990; Hamadi et al. 2000; Vanderbilt et al. 2008; Austin 2011) or litter quality (Schaefer et al. 1985; Steinberger and Whitford 1988; Austin 2011). Several alternative controls have been proposed to explain the discrepancies of litter mass loss in arid and semiarid ecosystems with global climatic and litter quality models. First, beyond the actual amount of precipitation inputs, the pulsed nature of precipitation events in aridlands has been identified as an important factor affecting organic matter decomposition, through the dry/wet cycles that affect organic carbon turnover (Austin et al. 2004; Collins et al. 2008), and through the instantaneous response of microbes to soil moisture changes (Schwinning and Sala 2004). Additionally, some recent studies have quantified that photodegradation, the photochemical mineralization of organic matter, is a driver of carbon loss in aridlands (Austin and Vivanco 2006; Brandt et al. 2009; Rutledge et al. 2010). As photodegradation functions largely independently of precipitation inputs, it could explain the higher rates of litter mass loss in aridland ecosystems, which usually receive larger solar radiation inputs than mesic and humid ecosystems (Austin and Vivanco 2006; Throop and Archer 2007).
In addition, soil fauna (macro- and mesofauna) have been postulated as an important control of litter decomposition in aridlands, with effects on decomposition that are disproportionate with respect to their biomass. In particular, termites and ants in some warm deserts have been identified as key players responsible for high rates of litter mass loss and nutrient release in these ecosystems (Santos and Whitford 1981; Schuurman 2005; Wagner and Jones 2006; Ginzburg et al. 2008). Several studies have demonstrated a significant reduction in leaf litter decomposition when soil fauna have been intentionally manipulated or excluded in tropical and subtropical forests (Yamashita and Takaeda 1998; Green et al. 1999; Heneghan et al. 1999; González and Seastedt 2001; Höfer et al. 2001; Yang and Chen 2009), and grasslands (Vossbrinck et al. 1979; Bradford et al. 2002; Smith and Bradford 2003; Wall et al. 2008). However, the effect of soil fauna on litter decomposition at a global scale is not strongly apparent, as a global study of their impacts showed modest and biome-specific effects of the exclusion of mesofauna (Wall et al. 2008).
Several studies have also suggested a key role for fungi in litter decomposition in aridlands. The preponderance of stochastic precipitation pulse events (Collins et al. 2008), and low concentrations of soil organic matter distributed in patches (Schlesinger et al. 1996; Austin et al. 2004; Gonzalez-Polo and Austin 2009), provide a suite of conditions with advantages for fungi, which exhibit high desiccation tolerance (Wilson and Griffin 1975) and an ability to thrive in relatively dry conditions (Went and Stark 1968). For instance, in a recent study in a semiarid Mediterranean ecosystem, fungi resisted simulated drought more successfully than bacteria, with direct implications for soil organic matter turnover (Yuste et al. 2010). In addition, a selective reduction in the soil microbial community has demonstrated substantial reductions in litter decomposition (Santos and Whitford 1981; Parker et al. 1984). This evidence suggests that fungal abundance and activity may be important affecting litter decomposition in warm desert ecosystems.
The Patagonian steppe is a semiarid ecosystem with vegetation of a mixture of shrubs and grasses interspaced in a bare soil matrix (Golluscio et al. 1998). Soil organic matter is also heterogeneously distributed in the landscape (Austin et al. 2006; Gonzalez-Polo and Austin 2009). Aboveground litter decomposition in open areas in this ecosystem has been shown to be controlled principally by abiotic photodegradation (Austin and Vivanco 2006), but the relative contribution of soil fauna and fungi on the decomposition process in the Patagonian steppe has not been explicitly evaluated. The patchy distribution of vegetation results in two distinct microsites where bare soil patches are exposed to high levels of solar radiation and vegetated patches have high concentrations of soil resources, microbial biomass and soil enzymatic activity (López et al. 2003; Gonzalez-Polo and Austin 2009). These vegetation microsites also differentiate the feeding patterns by granivorous ants and habitat use by beetles (Folgarait and Sala 2002; Mazía et al. 2006), and in particular, darkling and ground beetle (Tenebrionidae, Carabidae) activity is greater beneath shrubs than in bare soil areas (Mazía et al. 2006).
The objective of this study was to quantify the relative importance of soil organisms on aboveground litter decomposition in vegetated patches of the semiarid Patagonian steppe. We predicted that: (1) soil fauna (macro- and mesofauna) have an important impact on litter decomposition, and their exclusion would significantly decrease rates of mass loss; and (2) fungi play a key role in biotic litter decomposition, and the reduction of fungi populations from soil and litter would significantly decrease the rate of mass loss. Our experimental approach was to manipulate the access of soil organisms to decomposing litter as a function of body size (Anderson 1988), using different mesh sizes and chemical repellents to restrict the access of specific functional groups of soil fauna to the litter. In addition, we examined the consequences of reducing fungi from soil and litter on the dynamics of litter decomposition and nutrient release.
Materials and methods
Study site
The experiment was conducted at the Río Mayo experimental station of the National Institute for Agricultural Technology (INTA), a semiarid temperate shrub-steppe, in the Patagonian region of Argentina (45°41′S, 70°16′W). Average annual precipitation is 170 mm, occurring primarily during autumn and winter (March to August). Mean monthly temperature ranges between 1°C in July and 15°C in January. The topography is flat and soils are derived from glacial and volcanic materials, where gravel and stones are commonly found (Paruelo et al. 1988). Average soil organic matter content is very low (<1%) and is heterogeneously distributed (Gonzalez-Polo and Austin 2009).
The vegetation is a mix of tussock grasses and shrubs in a 50% bare soil matrix (Golluscio et al. 1998). The dominant grasses (32% basal cover) are Stipa speciosa Trin et Rupr., Stipa humilis Cav. and Poa ligularis Nees ap., and in lesser proportion, two high-palatable grass species, Bromus pictus Hook F and B. setifolius Presl. The dominant shrubs (15% basal cover) are Mulinum spinosum (Cav.) Pers., Adesmia volckmannii Philippi and Senecio filiginoides DC (Sala et al. 1989; Golluscio and Oesterheld 2007). Long-term annual aboveground primary production is 56 g m−2 year−1 (Jobbágy and Sala 2000).
Experimental design
We conducted two field litter decomposition experiments simultaneously over a 3-year incubation period: (1) a soil fauna exclusion experiment; and (2) a fungi reduction experiment. We selected 15 shrubs of Adesmia volckmannii of approximately 0.7–1.3 m in diameter and 1 m height within a grazing exclosure established in 1984. We used the shrub microsite because it has shown highest biotic soil activity in the study site (Gonzalez-Polo and Austin 2009). All aboveground herbaceous vegetation was carefully removed below the shrub canopy prior to the beginning of the experiment (January), with an effort to minimize soil disturbance. In order to reduce the photodegradative effects on litter decomposition (Austin and Vivanco 2006), and to homogenize solar radiation interception in all shrub microsites, we covered the soil area below the shrub canopy with a 50% shadecloth, supported by a wire circle of approximately 1.5 m in diameter at 0.2 m above the soil surface. We recognize that this experimental design did not allow for an evaluation of potential interactions between photodegradation and soil faunal decomposition, but our goal was to maintain all microsites with homogenous light conditions simulating a dense shrub canopy in order to avoid confounding effects of variation in plant cover among the treatments.
We constructed litterboxes of 25 × 8 × 1 cm using wire mesh (Blair et al. 1991), with the three different mesh sizes above (10, 2, 0.01 mm mesh) to allow access to macro- meso- and microfauna, respectively (Swift et al. 1979; Bradford et al. 2002). We used a 2-mm mesh below the litterboxes for the 10-mm treatment in order to prevent litter fragments losses but allowing macrofaunal access to the litter (Vossbrinck et al. 1979; Bradford et al. 2002). We placed litter from recently senesced leaves of a native palatable grass species, Bromus pictus in the litterboxes, which was collected in the study site and then left air-dried in the laboratory. We chose this palatable species in particular in order to maximize the possibility of detecting soil faunal effects on decomposition. We placed 1.500 g of air-dried litter in each litterbox, loosely tied with a fine copper wire to minimize losses. Each litterbox was transported to and from the field in individual paper bags to minimize litter loss. Any material that remained in the paper bags after transportation was subtracted from the initial litter mass.
The shrub plots were randomly assigned either to control plots, naphthalene addition or fungi reduction treatment (n = 5 for each treatment). The soil fauna exclusion experiment (experiment 1) was established using three mesh-sizes litterboxes: (1) 10-mm mesh, which permitted access of all soil fauna (10 mm), (2) 2-mm mesh, which excluded access for macrofauna (2 mm), and (3) 0.01-mm mesh, which excluded access for both macro- and mesofauna (0.01 mm). We placed 6 litterboxes of each mesh size (10, 2 and 0.01 mm) in control and naphthalene plots for a total of 18 litterboxes in each plot. We added a chemical exclusion product (napthalene) on the soil surface below one-third of the shrub plots (n = 5), a repellant which has been used in a number of other studies for chemical exclusion of soil arthropods (Yamashita and Takaeda 1998; Green et al. 1999; Heneghan et al. 1999; González and Seastedt 2001; Höfer et al. 2001; Wall et al. 2008; Yang and Chen 2009). Any artifact of mesh-size effect on mass loss could thus be evaluated comparing litter decomposition for the three types of mesh exclusion with and without naphthalene addition.
The fungi reduction experiment (experiment 2) was established with 0.01-mm mesh litterboxes, placed in shrub plots treated with a fungicide (0.01 mm + fungicide). We placed six litterboxes of the 0.01 mm treatment in each fungicide treated plots (n = 5), while the 0.01-mm mesh litterboxes in the control shrub plots from experiment 1 functioned as the control treatment for this experiment. All litterboxes (210 in total) were placed in mid-summer (January) on the soil surface and anchored to the soil securely with wire clips.
Naphthalene and fungicide were applied to each of the five shrubs assigned to each treatment at the beginning of the incubation period and then three times a year during 3 years. Naphthalene in crystalline form (“mothballs”) was placed on soil surface near the litterboxes at 100 g m−2 (Blair et al. 1989), and fungicide (Captan®) was applied on the soil and litterbox surfaces in an aqueous solution (17.5 g m−2 dissolved in 3 l of water) (Beare et al. 1992). In order to compensate for any effects of water addition among treatments, the same amount of water was applied to control and naphthalene shrub plots at each time point.
Litterboxes were collected at 4, 9, 12, 16, 24 and 36 months from the beginning of the experiment, transported in paper bags, and placed immediately in an oven for at least 24 h. The litter of each sample was carefully cleaned by removing foreign plant material and soil, and dried in an oven at 60°C for 48 h for dry mass determination. In order to correct the samples for inorganic soil contamination, we estimated initial and final organic matter content with determinations of ash-free dry mass (400°C, 4 h) (Harmon et al. 1999). We estimated the annual rate decay constant (k, year−1) using a simple negative exponential model by regressing the logarithm of the fraction of mass remaining against time, using the equation:
where M0 is the initial ash-free dry mass, Mt is the ash-free dry mass at time t, b is the intercept and k (the slope of the function) is the decay constant (Olson 1963). Linear regressions were performed setting the intercept to zero (Vivanco and Austin 2008) and we estimated a decomposition constant (k) for each shrub plot and mesh size. All regressions used to estimate litter mass loss through time were highly significant (r 2 > 0.90).
In the fungi reduction experiment, we analyzed litter nitrogen (N) release through time. Litter N concentration at each sampling date (initial included) was determined in both 0.01-mm and 0.01-mm + fungicide treatments by Dumas combustion with a TruSpec® elemental analyzer (LECO, St. Joseph, MI, USA) at the laboratory in the School of Agronomy, University of Buenos Aires. Litter from these fine-mesh treatments had very little soil contamination but were not corrected for possible inclusion of soil N, as total soil N under shrub canopies is less than 0.10% (Gonzalez-Polo and Austin 2009).
Site and litter characterization
During two consecutive years, we took surface soil samples (0–5 cm) in control and fungicide-treated shrub plots. We sieved soils to pass a 2-mm mesh and then a subsample was dried in a 105°C oven for 48 h to determine gravimetric soil water content. Another subsample of 5 g was extracted in the field with 25 ml of KCl 2 N for determinations of inorganic nitrogen. In the laboratory, these samples were filtered and analyzed colorimetrically for NH4-N and NO3-N on an Alpkem® autoanalyzer (O–I Analytical, College Station, TX, USA).
To assess the abundance of macrofauna in the site, we placed five pitfall traps (Gist and Crossley 1973) containing a solution of 70% ethylene glycol and 30% alcohol (95 cm2) for a 5-day period at the end of the first year of incubation (December, late spring) beneath control shrub canopies (n = 5). All macrofauna trapped was preserved in the solution, hand picked in the laboratory and then sorted under a magnifying glass (Nikon SMZ 800) to the taxonomic level of order (e.g., Aranae, Hymenoptera, Coleoptera).
The effect of fungicide addition on fungal colonization of litter was tested after 1 year of incubation in the field. Litter subsamples (100 mg) from the 0.01-mm and 0.01-mm + fungicide treatments (n = 5) were collected and placed in dilution bottles (falcon tubes) with 2 ml extraction buffer (0.88%. NaCl). Ten-fold dilutions were spread-plated in nutritive agar (potato-dextrose agar, with 50 µg ml−1 chlortetracycline and 200 µg ml−1 cycloheximide) for determination of fungal coloniziation (Donegan et al. 2001). Plates were incubated at 25°C for 72 h and the colony-forming units (CFUs) were counted.
Statistical analyses
Organic matter remaining (%) at each sampling date, litter decomposition constants (k) and nutrient release data (expressed as percentage of original values) were compared using a one-way ANOVA, as well as data for gravimetric soil water content, and soil NH4-N and NO3-N content in control and fungicide shrub plots. Mass loss (%) of years 1, 2, and 3 of incubation for each mesh size were regressed against mass loss (%) of years 1, 2, and 3 for each exclusion treatment (mesh size and chemical exclusion), assuming that any deviations from the 1:1 line could be associated with potential treatments effects (Wall et al. 2008). Independent t tests were performed with equal variances, with the hypothesis that the mesh size and chemical exclusions would only decrease litter mass loss. When necessary, data were transformed to account for the assumptions of the analysis of variance, and a Kruskal–Wallis non-parametric test was used for soil nitrate. Unless otherwise stated, we used 5% for statistical significance; all mean values stated in the text are followed by standard errors in parentheses. We analyzed data with INFOSTAT/Profesional (1.1 Version; Universidad Nacional de Córdoba, Estadística y Diseño, Argentina).
Results
Effects of exclusion of soil fauna on litter decomposition
Among macrofauna evaluated in the soil surface with the pitfall traps, six taxonomic groups of arthropods were identified (Table 1). Two of the most abundant groups of soil organisms, Coleoptera and Hymenoptera, are known to include litter decomposer organisms (Brussaard 1997), although we did not have the taxonomic resolution to identify them. On average, a total of 3.8 (±0.41) individuals trap−1 were found under each shrub, with no evidence of termite presence, and very low number of ants (Hymenoptera, Table 1).
Physical exclusion of macro and mesofauna (‘soil fauna’ from this point forward) had no effect on litter decomposition. Organic matter remaining (%) was similar among mesh exclusion treatments of 10, 2, and 0.01 mm at all sampling dates (Fig. 1a). On average, between 31 and 33% of the initial litter was decomposed after 3 years of field incubation among all treatments (Fig. 1a). Consequently, litter decomposition constants (k, year−1) did not differ significantly among treatments (P = 0.36; Fig. 1b), with average values of 0.14 (±0.009), 0.12 (±0.006) and 0.14 (±0.009) for the 10, 2 and 0.01 mm treatments, respectively (standard error in parentheses).
Bromus pictus litter decomposition with physical exclusion of macro and mesofauna. a Mean values of organic matter remaining over time (±SE, n = 5) for each treatment: no soil fauna exclusion (10 mm mesh, open circles); macrofauna exclusion (2-mm mesh, gray circles); and macro and mesofauna exclusion (0.01-mm mesh, black circles). b Mean litter decomposition constants after 3 years (k, year−1) (±SE, n = 5) for 10 mm (open bar), 2 mm (gray bar), and 0.01 mm (black bar)
Chemical exclusion of soil fauna (litterboxes of 10, 2 and 0.01 mm with naphthalene addition) showed no effect on litter decomposition as litter decomposition constants were similar to control treatments without naphthalene addition (Fig. 2). Together, manipulative soil fauna exclusion (either physical or chemical) demonstrated no effect on litter mass loss (T 1,34 = 0.04, P = 0.51; Fig. 2).
Comparison of physical and chemical exclusion of soil fauna on litter decomposition in the Patagonian steppe. Solid line represents 1:1 relationship either between chemical exclusion and no chemical exclusion treatments, or between different mesh-size exclusion treatments. Chemical exclusion (diamonds), physical exclusion (triangles); values represent comparisons of treatment means at 1, 2 and 3 years. Different shading represents different mesh sizes in both types of exclusion: chemical exclusions effects (10 mm vs. 10 mm + naphthalene, open diamonds; 2 mm vs. 2 mm + naphthalene, gray diamonds; 0.01 mm vs. 0.01 mm + naphthalene, black diamonds); and mesh-size exclusion effects (10 vs. 2 mm, open triangles; 10 vs. 0.01 mm, gray triangles; 2 vs. 0.01 mm, black triangles). No significant exclusion effect was found (T 1,34 = 0.04, P = 0.51)
Effects of fungi reduction on litter decomposition and litter nitrogen release
The fungicide application significantly reduced fungal colonization on litter. CFUs from the 0.01 mm + fungicide treatment were significantly lower than in the 0.01 mm (average CFU for fungicide treatment = 20 (±10.6); untreated litter = 219 (±73.8), F 1,8 = 16.54, P = 0.0036). Soil water content and soil NH4-N and NO3-N content were similar between control and fungicide plots in all sampling dates (Table 2). Mean ammonium concentration in surface soils underneath shrubs for the first year was 3.92 (±1.44) μg g−1 dry soil, and mean nitrate concentration was 2.29 (±0.61) μg g−1 dry soil (n = 5).
Fungi reduction had significant effects on litter decomposition and nitrogen release (Fig. 3). Litter decomposition was significantly slowed in the fungicide treatment, with k constants of 0.14 (±0.004) year−1 and 0.06 (±0.002) year−1, for the control and fungicide treatments, respectively (n = 5). Significant differences in the organic matter remaining (%) between treatments were evident from the second sampling date (Fig. 3a). Only 12% of the initial litter was lost after 3 years of decomposition in the fungicide treatment, whereas 33% was lost in the 0.01 mm treatment (Fig. 3a). Litter decomposing in both fine-mesh treatments immobilized nitrogen during the 3 years of litter decomposition, with stronger immobilization in the 0.01 mm + fungicide treatment, particularly in the second and third years of the incubation (Fig. 3b). Differences in C:N ratios of the decomposing litter also varied over time (Fig. 3c), with a stronger decline in C:N ratios of the 0.01 mm treatment for the first 2 years, which is consistent with increased rates of mass loss in this treatment. However, the immobilization of nitrogen in the 0.01 mm + fungicide treatment continued well into the third year when C:N ratios of both fine mesh exclusion treatments declined (Fig. 3b, c).
Microbial decomposition of litter of Bromus pictus and N release with reduction of soil fungi. a Mean organic matter remaining over time, (%, ±SE, n = 5) at each time point: macro-and mesofauna exlusion (0.01 mm, filled circles) and fungi reduction (0.01 mm + fungicide, filled triangles); b Mean nitrogen remaining (% of initial N, ±SE, n = 5) over time. c C:N ratios over time (±SE, n = 5). Significant differences between treatments are noted (*P < 0.05, **P < 0.01, ***P < 0.001)
Discussion
In this study, we evaluated the contribution of soil organisms on aboveground leaf litter decomposition in the temperate Patagonian steppe, using litterboxes with different mesh sizes and chemical repellents that selectively excluded soil fauna groups. Our results do not provide evidence of an important control of soil fauna in aboveground litter decomposition in this semiarid ecosystem. We found that the physical and chemical exclusion of macro- and mesofauna did not affect litter mass loss and consequently, litter decomposition constants were similar among soil fauna exclusion treatments. However, we found that litter decomposed much more slowly when fungal populations were reduced, suggesting an important role of fungi in aboveground litter decomposition and nutrient dynamics in this semiarid shrub steppe (Fig. 3).
Soil fauna exclusion
The physical and chemical exclusion of macro- and mesofauna did not modify litter mass loss (%) or decomposition constants integrated over the 3-year incubation period (Figs. 1 and 2). Recent studies have demonstrated biome-specific effects of soil fauna on litter decomposition in humid or tropical regions (e.g., Heneghan et al. 1999; González and Seastedt 2001; Höfer et al. 2001; Bradford et al. 2002; Yang and Chen 2009), but strong soil faunal effects were not observed in cold and/or arid ecosystems (Wall et al. 2008). Our results support a minimal role of soil invertebrates on litter decomposition in the semiarid Patagonian steppe, which is in agreement with results from the global experiment of Wall et al. (2008). It appears that the role of soil fauna affecting litter decomposition in arid and semiarid ecosystems may be restricted to particular sites where the combination of faunal community and climate allow for these organisms to flourish, but it does not appear that it is a general characteristic of temperate aridland ecosystems.
The disproportionate role of some macrofauna on litter decomposition may be a particular trait of some warm aridlands, particularly where termite or ant guilds are an abundant component of the soil fauna. In fact, studies in North American and African deserts attribute faster litter decomposition than that predicted by climate indices specifically to termite activity (Santos and Whitford 1981; Schuurman 2005); however, these studies were conducted in warm desert ecosystems where termites are abundant (but see Noble et al. 2009). In contrast, in cold deserts, particularly in the southern hemisphere where temperature could constrain biological activity (Wood 1988) and biogoeographic barriers could limit the distribution of termite species, their role as ecosystem engineers may not be prominent, which is consistent with the results of this study.
Considering that we did not find any of the key ‘desert decomposers’ such as termites or ants in our study site, and that the overall macrofauna abundance was relatively low compared to other studies in deserts (Doblas-Miranda et al. 2007) (Table 1), it is reasonable that we observed no response in aboveground litter decomposition to soil macro- and mesofauna exclusion. There are a number of characteristics of the Patagonian steppe that might explain the lack of key macrofaunal decomposers. First, the months of highest rainfall do not coincide with the growing season (Golluscio and Oesterheld 2007) and hence, peaks of resource availability for the soil fauna occur under conditions of low moisture availability. In addition, the asynchrony between water availability and favorable temperatures may constrain the abundance and activity of soil organisms (Heneghan et al. 1999; Schwinning and Sala 2004). Additionally, soil fauna density diminishes with latitude globally (Swift et al. 1979; Heneghan et al. 1999), and deserts are the biomes with the lowest overall soil faunal biomass (Fierer et al. 2009). This combination of climatic and biogeographic factors could explain the absence of soil fauna contribution in aboveground litter decomposition in the semiarid Patagonian steppe when compared to tropical and hot desert ecosystems.
Independent of the effects of faunal exclusion, litter decomposition in this study was lower than others reported in the same study site. Comparing k constants for the same litter incubation period, we reported on average, 0.14 year−1 for the control treatment, while average k constants from others studies in the same site ranged from 0.24 to 0.35 year−1 (Austin et al. 2006; Austin and Vivanco 2006; Yahdjian et al. 2006). The effects of the shadecloth and shrub canopies in reducing incident solar radiation at the soil surface appears to have had a much larger effect than our soil faunal exclusion treatments, supporting the role of photodegradation as a major control on decomposition in this site (Austin and Vivanco 2006; Austin 2011). These results echo other studies in aridlands suggesting abiotic factors such as solar radiation, wind and freeze–thaw cycles are important controls degrading, fragmenting and redistributing aboveground litter (Moorhead and Reynolds 1989; Gallo et al. 2006; Throop and Archer 2007; Noble et al. 2009; Uselman et al. 2011) rather than a direct biotic control on rates of mass loss. Nevertheless, this experiment did not allow for the evaluation of indirect effects of photodegradation on litter quality, which could potentially interact with soil organisms affecting carbon turnover and litter decay (Gallo et al. 2009; Austin and Ballaré 2010).
Fungi reduction
In contrast with the results for soil faunal exclusion, the role of fungi in biotic degradation of litter is important in the semiarid Patagonian steppe, as originally hypothesized. When fungi were manipulatively reduced, litter decomposed significantly less and with significantly stronger nitrogen immobilization (Fig. 3).
Fungi tolerance to desiccation and the capacity to secrete extracellular enzymes to assimilate several substrates simultaneously (Wilson and Griffin 1975; Whitford and Parker 1989; Austin et al. 2004; Yuste et al. 2010) may explain why litter decomposition was diminished when fungi were reduced. A similar reduction in the magnitude of decomposition was shown in the Chihuahuan desert when fungi were reduced with chemical inhibitors (Santos and Whitford 1981; Parker et al. 1984; Whitford et al. 1986). In this study, it is not clear whether the reduction in fungal abundance changed bacterial populations or other microfloral and microfaunal groups, although we did not observe any compensatory microbial responses which resulted in positive effects on rates of mass loss in litter from fungicide-treated plots. We were not able to evaluate the potential fungi reduction–soil organism interactions, which have been shown to be important in affecting soil faunal dynamics in warm desert ecosystems (Parker et al. 1984) and could be particularly important when termites are abundant (Schuurman 2005). Overall, the strong reduction in decomposition appears to arise from the direct impact on fungal populations and their controls on carbon turnover in this ecosystem.
Net nitrogen immobilization was observed over time in both treatments (Fig. 3b), but a stronger immobilization occurred in the fungicide treatments and continued over the entire incubation period. The C:N ratios tracked mass loss changes during the first 2 years of the experiment, suggesting that decreased C:N ratios could be explained in part by loss of carbon from decomposition. Additionally, the differences in nitrogen immoblization between the two treatments could stem from stoichiometric constraints on different microbial groups such as fungi or bacteria (Manzoni et al. 2008). In other experiments in the Patagonian steppe, where litterbags were placed in bare soil microsites, litter nitrogen immobilization was very low (Yahdjian et al. 2006) or non-existent (Austin et al. 2006). The results from this study support the assertion that the area beneath shrubs is a hotspot for biotic activity in this ecosystem, as opposed to bare soil areas where abiotic controls dominate (Gonzalez-Polo and Austin 2009).
Although soil fauna have been mentioned as a key control of litter decomposition in some aridlands, our results highlight that this could not be generalized globally to all arid and semiarid ecosystems. Alternatively, although we did not observe any effect of soil fauna on aboveground litter decomposition, soil organisms may be critical belowground (Collins et al. 2008) while position of litter, aboveground or buried, could be critical in determining the principal controls on mass loss (Austin et al. 2009). In addition, the contribution of soil fauna to litter decomposition appear to depend on site-specific factors, such as faunal richness and abundance (Wall et al. 2008) which in turn may be influenced by factors such as soil fertility, trophic interactions and evolutionary history (Powers et al. 2009).
References
Aerts R (1997) Climate, leaf litter chemistry and leaf litter decomposition in terrestrial ecosystems: a triangular relationship. Oikos 79:439–449
Anderson JM (1988) Spatiotemporal effects of inverterbrates on soil processes. Biol Fert Soils 6:216–227
Austin AT (2011) Has water limited our imagination for aridland biogeochemistry? Trends Ecol Evol 26:229–235
Austin AT, Ballaré CL (2010) Dual role of lignin in plant litter decomposition in terrestrial ecosystems. Proc Natl Acad Sci USA 107:4618–4622
Austin AT, Vitousek PM (2000) Precipitation, decomposition, and litter decomposability of Metrosideros polymorpha on Hawaii. J Ecol 88:129–138
Austin AT, Vivanco L (2006) Plant litter decomposition in semi-arid ecosystems controlled by photodegradation. Nature 442:555–558
Austin AT et al (2004) Water pulses and biogeochemical cycles in arid and semiarid ecosystems. Oecologia 141:221–235
Austin AT, Sala OE, Jackson RB (2006) Inhibition of nitrification alters carbon turnover in the Patagonian steppe. Ecosystems 9:1257–1265
Austin AT, Araujo PI, Leva PE (2009) Interaction of position, litter type, and water pulses on decomposition of grasses from the semiarid Patagonian steppe. Ecology 90:2642–2647
Beare MH, Parmelee RW, Hendrix PF, Cheng W, Coleman DC, Crossley DA Jr (1992) Microbial and faunal interactions and effects on litter nitrogen and decomposition in agroecosystems. Ecol Monogr 62:569–591
Blair JM, Crossley DA Jr, Rider S (1989) Effects of napthalene on microbial activity and nitrogen pools in soil-litter microcosms. Soil Biol Biochem 21:507–510
Blair JM, Crossley DA Jr, Callaham LC (1991) A litterbasket technique for measurement of nutrient dynamics in forest floors. Agric Ecosys Envir 34:465–471
Bontti EE et al (2009) Litter decomposition in grasslands of Central North America (US Great Plains). Glob Change Biol 15:1356–1363
Bradford MA, Tordoff GM, Eggers T, Jones TH, Newington JE (2002) Microbiota, fauna, and mesh-size interactions in litter decomposition. Oikos 99:317–323
Brandt LA, Bohnet C, King JY (2009) Photochemically induced carbon dioxide production as a mechanism for carbon loss from plant litter in arid ecosystems. J Geophys Res 114:G02004
Brussaard L (1997) Biodiversity and ecosystem functioning in soil. Ambio 26:563–570
Coleman DC, Crossley DA (1996) Fundamentals of soil ecology. Academic, New York
Collins SL et al (2008) Pulse dynamics and microbial processes in aridland ecosystems. J Ecol 96:413–420
Cornwell WK et al (2008) Plant species traits are the predominant control on litter decomposition rates within biomes worldwide. Ecol Lett 11:1065–1071
Coûteaux MM, Bottner P, Berg B (1995) Litter decomposition, climate and litter quality. Trends Ecol Evol 10:63–66
Doblas-Miranda E, Sánchez-Pi ero F, González-Megías A (2007) Soil macroinvertebrate fauna of a Mediterranean arid system: composition and temporal changes in the assemblage. Soil Biol Biochem 39:1916–1925
Donegan KK et al (2001) Soil and litter organisms in Pacific northwest forests under different management practices. Appl Soil Ecol 18:159–175
Fierer N, Strickland MS, Liptzin D, Bradford MA, Cleveland CC (2009) Global patterns in belowground communities. Ecol Lett 12:1238–1249
Folgarait PJ, Sala OE (2002) Granivory rates by rodents, insects, and birds at different microsites in the Patagonian steppe. Ecography 25:417–427
Gallo ME, Sinsabaugh RL, Cabaniss SE (2006) The role of ultraviolet radiation in litter decomposition in arid ecosystems. Appl Soil Ecol 34:82–91
Gallo ME, Porras-Alfaro A, Odenbach KJ, Sinsabaugh RL (2009) Photoacceleration of plant litter decomposition in an arid environment. Soil Biol Biochem 41:1433–1441
Gholz HL, Wedin D, Smitherman SM, Harmon ME, Parton WJ (2000) Long-term dynamics of pine and hardwood litter in contrasting environments: toward a global model of decomposition. Glob Change Biol 6:751–765
Ginzburg O, Whitford G, Steinberger Y (2008) Effects of harvester ant (Messor spp.) activity on soil properties and microbial communities in a Negev Desert ecosystem. Biol Fertil Soils 45:165–173
Gist CS, Crossley DA (1973) A method for quantifying pitfall trapping. Environ Entom 2:951–952
Golluscio RA, Oesterheld M (2007) Water use efficiency of twenty-five co-existing Patagonian species growing under different soil water availability. Oecologia 154:207–217. doi:10.1007/s00442-007-0800-5
Golluscio RA, Sala OE, Lauenroth WK (1998) Differential use of large summer rainfall events by shrubs and grasses: a manipulative experiment in the Patagonian steppe. Oecologia 115:17–25
González G, Seastedt TR (2001) Soil fauna and plant litter decomposition in tropical and subalpine forests. Ecology 82:955–964
Gonzalez-Polo M, Austin AT (2009) Spatial heterogeneity provides organic matter refuges for soil microbial activity in the Patagonian steppe, Argentina. Soil Biol Biochem 41:1348–1351
Green PT, Lake PS, O’Dowd DJ (1999) Monopolization of litter processing by a dominant land crab on a tropical oceanic island. Oecologia 119:435–444
Hamadi Z, Stenberger Y, Kutiel P, Lavee H, Barness G (2000) Decomposition of Avena sterilis litter under arid conditions. J Arid Environ 46:281–293
Harmon ME, Nadelhoffer KJ, Blair JM (1999) Measuring decomposition, nutrient turnover, and stores in plant litter. In: Robertson GP, Coleman DC, Bledsoe CS, Sollins P (eds) Standard soil methods for long-term ecological research. Oxford University Press, Oxford, pp 202–240
Heneghan L, Coleman DC, Zou X, Crossley DA, Haines BL (1999) Soil microarthropod contributions to decomposition dynamics: tropical-temperate comparisons of a single substrate. Ecology 80:1873–1882
Höfer H et al (2001) Structure and function of soil fauna communities in Amazonian anthropogenic and natural ecosystems. Eur J Soil Biol 37:229–235
Jobbágy EG, Sala OE (2000) Controls of grass and shrub aboveground production in the Patagonian steppe. Ecol Appl 10:541–549
López NI, Austin AT, Sala OE, Méndez B (2003) Controls on nitrification in a water-limited ecosystem: experimental inhibition of ammonia-oxidizing bacteria in the Patagonian steppe. Soil Biol Biochem 35:1609–1613
Manzoni S, Jackson R, Trofymow J, Porporato A (2008) The global stoichiometry of litter nitrogen mineralization. Science 321:684
Mazía CN, Chaneton EJ, Kitzberger T (2006) Small-scale habitat use and assemblage structure of ground-dwelling beetles in a Patagonian shrub steppe. J Arid Environ 67:177–194
Meentemeyer V (1978) Macroclimate and lignin control of litter decomposition rates. Ecology 59:465–472
Moorhead DL, Reynolds JF (1989) Mechanisms of surface litter mass loss in the northern Chihuahuan desert: a reinterpretation. J Arid Environ 16:157–163
Noble JC, Müller WJ, Whitford WG, Pfitzner GH (2009) The significance of termites as decomposers in contrasting grassland communities of semi-arid eastern Australia. J Arid Environ 73:113–119
Olson JS (1963) Energy storage and the balance of producers and decomposers in ecological systems. Ecology 44:331–332
Parker L, Santos P, Phillips J, Whitford W (1984) Carbon and nitrogen dynamics during the decomposition of litter and roots of a Chihuahuan desert annual, Lepidium lasiocarpum. Ecol Monogr 54:339–360
Paruelo JM, Aguiar MR, Golluscio RA (1988) Soil water availability in the Patagonian arid steppe: gravel content effect. Arid Soil Res Rehab 2:67–74
Powers JS et al (2009) Decomposition in tropical forests: a pan-tropical study of the effects of litter type, litter placement and mesofaunal exclusion across a precipitation gradient. J Ecol 97:801–811
Rutledge S, Campbell DI, Baldocchi D, Schipper LA (2010) Photodegradation leads to increased CO2 losses from terrestrial organic matter. Glob Change Biol 16:3065–3074. doi:10.1111/j.1365-2486.2009.02149.x
Sala OE, Golluscio RA, Lauenroth WK, Soriano A (1989) Resource partitioning between shrubs and grasses in the Patagonian steppe. Oecologia 81:501–505
Santos PF, Whitford WG (1981) The effect of microarthropods on litter decomposition in Chihuahuan Desert Ecosystem. Ecology 62:654–663
Schaefer D, Steinberger Y, Whitford WG (1985) The failure of nitrogen and lignin control of decomposition in a North American desert. Oecologia 65:382–386
Schlesinger WH, Raikes JA, Hartley AE, Cross AF (1996) On the spatial pattern of soil nutrients in desert ecosystems. Ecology 77:364–374
Schuurman G (2005) Decomposition rates and termite assemblage composition in semiarid Africa. Ecology 86:1236–1249
Schwinning S, Sala OE (2004) Hierarchy of responses to resource pulses in arid and semi-arid ecosystems. Oecologia 141:211–220
Smith VC, Bradford MA (2003) Litter quality impacts on grassland litter decomposition are differently dependent on soil fauna across time. Appl Soil Ecol 24:197–203
Steinberger Y, Whitford WG (1988) Decomposition process in Negev ecosystems Oecologia 75:61–66
Steinberger Y, Shmida A, Whitford WG (1990) Decomposition along a rainfall gradient in the Judean desert, Israel. Oecologia 82:322–324
Swift MJ, Heal OW, Anderson JM (1979) Decomposition in terrestrial ecosystems. University of California Press, Berkeley
Throop H, Archer S (2007) Interrelationships among shrub encroachment, land management, and litter decomposition in a semidesert grassland. Ecol Appl 17:1809–1823
Uselman SM, Snyder KA, Blank RR, Jones TJ (2011) UVB exposure does not accelerate rates of litter decomposition in a semi-arid riparian ecosystem. Soil Biol Biochem 43:1254–1265
Vanderbilt KL, White CS, Hopkins O, Craig JA (2008) Aboveground decomposition in arid environments: Results of a long-term study in central New Mexico. J Arid Environ 72:696–709
Vitousek PM, Turner DR, Parton WJ, Sanford RL (1994) Litter decomposition on the Mauna Loa environmental matrix, Hawai’i: patterns, mechanisms, and models. Ecology 75:418–429
Vivanco L, Austin AT (2008) Tree species identity alters forest litter decomposition through long-term plant and soil interactions in Patagonia, Argentina. J Ecol 96:727–736
Vossbrinck CR, Coleman DC, Woolley TA (1979) Abiotic and biotic factors in litter decomposition in a semiarid grassland. Ecology 60:265–271
Wagner D, Jones JB (2006) The impact of harvester ants on decomposition, N mineralization, litter quality, and the availability of N to plants in the Mojave Desert. Soil Biol Biochem 38:2593–2601
Wall DH et al (2008) Global decomposition experiment shows soil animal impacts on decomposition are climate-dependent. Glob Change Biol 14:2661–2677
Went FW, Stark N (1968) The biological and mechanical role of soil fungi. Proc Nat Acad Sci USA 60:497–504
Whitford WG, Parker LW (1989) Contributions of soil fauna to decomposition and mineralization processes in semiarid and arid ecosystems. Arid Soil Res Rehab 3:199–215
Whitford WG et al (1981) Exceptions to the AET model: deserts and clear-cut forest. Ecology 62:275–277
Whitford WG et al (1986) Rainfall and decomposition in the Chihuahuan desert. Oecologia 68:512–515
Wilson JM, Griffin DM (1975) Water potential and the respiration of microorganisms in the soil. Soil Biol Biochem 7:199–204
Wood TG (1988) Termites and the soil environment. Biol Fert Soils 6:228–236
Yahdjian L, Sala OE, Austin AT (2006) Differential controls of water input on litter decomposition and nitrogen dynamics in the Patagonian steppe. Ecosystems 9:128–141
Yamashita T, Takaeda H (1998) Decomposition and nutrient dynamics of leaf litter in litter bags of two mesh sizes set in two dipterocarp forest sites in Peninsular Malaysia. Pedobiologia 42:11–21
Yang X, Chen J (2009) Plant litter quality influences the contribution of soil fauna to litter decomposition in humid tropical forests, southwestern China. Soil Biol Biochem 41:1–9
Yuste J et al (2010) Drought resistant fungi control soil organic matter decomposition and its response to temperature. Glob Change Biol 17:1475–1486. doi:10.1111/j.1365-2486.2010.02300.x
Acknowledgments
We acknowledge the University of Buenos Aires (UBACyT 812), ANPCyT (PICT 4/21247 5/31970 and 2008-108), and the Fundación Antorchas of Argentina for funding support. We are grateful to INTA for permission to use the facilities at the Río Mayo Experimental Field station. We thank L. Gherardi, P. Flombaum, M. Gonzalez-Polo, J.Vrsalovic and A. Grasso for field assistance and A. González-Arzac, A. Fernández-Souto P.Rojas, A. Tornese, and Lorena Grion for laboratory assistance. Lucía Vivanco provided invaluable helpful discussions regarding the project. We thank two anonymous reviewers who provided constructive criticism on a previous version of this manuscript. P.I.A. was supported by a University of Buenos Aires fellowship; L.Y. and A.T.A. are career investigators from CONICET. The experiments described here comply with the current laws of Argentina in which the experiments were performed.
Conflict of interest
None.
Author information
Authors and Affiliations
Corresponding authors
Additional information
Communicated by Zoe Cardon.
Rights and permissions
About this article
Cite this article
Araujo, P.I., Yahdjian, L. & Austin, A.T. Do soil organisms affect aboveground litter decomposition in the semiarid Patagonian steppe, Argentina?. Oecologia 168, 221–230 (2012). https://doi.org/10.1007/s00442-011-2063-4
Received:
Accepted:
Published:
Issue Date:
DOI: https://doi.org/10.1007/s00442-011-2063-4