Abstract
Aims
Fungi play a central role in litter decomposition, a key process controlling the terrestrial carbon cycle and nutrient availability for plants and microorganisms. Climate change and elevated CO2 affect soil fungi, but the relative importance of the global change variables for litter decomposition is still uncertain. The main objective was therefore to assess the short-term litter decomposition and associated fungal community in a global change manipulated temperate heath ecosystem.
Methods
The heath had been exposed to 6 years of warming, elevated atmospheric CO2 and an extended pre-summer drought. Litterbags with litter from heather (Calluna vulgaris) and wavy-hair grass (Deschampsia flexuosa) were incubated in the litter layer for 6 months, where after we analyzed the litter-associated fungal community, litter loss, CO2 respiration, and total content of carbon, nitrogen and phosphorus.
Results
Elevated temperature tended to increase litter decomposition rates, whereas elevated CO2 had no effect on the process. The pre-summer drought treatment had a positive impact on litter decomposition, CO2 respiration and fungal abundance in the litterbags, although we observed no major changes in fungal community composition.
Conclusions
The drought treatment during pre-summer had a legacy effect on litter decomposition as decomposition rates were positively affected later in the year. The community structure of litter-decomposing fungi was not affected by the drought treatment. Hence, the legacy effect was not mediated by a change in the fungal community structure.
Similar content being viewed by others
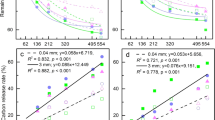
Explore related subjects
Discover the latest articles, news and stories from top researchers in related subjects.Avoid common mistakes on your manuscript.
Introduction
Litter decomposition is a key process in the terrestrial ecosystem carbon cycle and controls nutrient availability for plants and microorganisms. In terrestrial ecosystems, fungi play a central role in litter decomposition due to their abilities to produce a wide range of extracellular enzymes that attack complex organic compounds such as lignin, which other organisms are unable to decompose (Kjøller and Struwe 1982; de Boer et al. 2005). Since the composition of fungal communities are known to shift due to climate change (Zhang et al. 2005; Yuste et al. 2011) and elevated atmospheric CO2 (Drigo et al. 2008; Anderson et al. 2013), these global change factors can potentially alter litter decomposition and thereby ecosystem carbon and nutrient turnover (Couteaux et al. 1995; Aerts 1997).
Although previous studies have investigated factors controlling litter decomposition, the relative importance of global change variables on decomposition remain uncertain. For example although increased temperature can stimulate litter mass loss (van Meeteren et al. 2008; Andresen et al. 2010; Incerti et al. 2011) possibly as a result of increased microbial activity (van Meeteren et al. 2008), the positive effect of warming is reduced when soil moisture becomes limiting for microbial activity (Bontti et al. 2009; Butenschoen et al. 2011). Similarly, interactive effects of soil moisture and warming can reduce fungal abundance and activity (Allison and Treseder 2008). In connection with these observations, periodic drought has been shown to decrease litter decomposition (van Meeteren et al. 2008; Andresen et al. 2010; Sanaullah et al. 2012) probably due to reduced microbial activity (Jensen et al. 2003; Emmett et al. 2004; Selsted et al. 2012) and reduced enzyme activity (Toberman et al. 2008). Furthermore, drought can shift the microbial community towards a higher fungal dominance compared to bacteria (Yuste et al. 2011; Haugwitz et al. 2014).
The impact of elevated CO2 on litter decomposition and litter respiration rate does not show consistent patterns (Norby et al. 2001; Liu et al. 2009), but the effect is considered to be plant-mediated through the general nutrient status of the plants and for example the lignin to N ratio of the plant litter (Cotrufo et al. 1998; Norby and Cotrufo 1998). In addition, elevated CO2 can alter soil fungal community composition and increase fungal abundance, which also has a potential to change litter decomposition (Drigo et al. 2008; Anderson et al. 2013). Taken together, the global change effects on litter decomposition are complex with no emerging, consistent patterns, when considering the interactions between global change factors.
Here, we investigated the short-term litter decomposition and the associated fungal community in a heath ecosystem that had been subjected to 6 years of warming, elevated CO2 and pre-summer drought. Litterbags with litter of two dominant temperate heath plants, Calluna vulgaris and Deschampsia flexuosa, were incubated in the litter layer for 6 months followed by analyzing the decomposed litter with a combination of a molecular description of the litter-associated fungal community, litter loss, microbial CO2 respiration, and total carbon (C), nitrogen (N) and phosphorus (P) content of the litter. Increased temperature can directly affect microorganisms and stimulate activity (van Meeteren et al. 2008), whereas positive effects of elevated atmospheric CO2 on microorganisms are mostly mediated through plant roots (Drigo et al. 2008; Anderson et al. 2013). Consequently, we hypothesized that (H1) warming would stimulate the litter decomposing fungal community most strongly, that (H2) the positive effects of elevated CO2 on litter decomposition would be less pronounced and (H3) that the pre-summer drought treatment has a legacy effect on litter decomposition in autumn and early winter due to drought mediated changes in the composition of the litter-degrading fungal communities. Taken together, this experiment enabled us to investigate single-factor and interactive effects of increased temperature, elevated CO2 and pre-summer drought periods on litter decomposition in a mixed grass- and shrubland.
Materials and methods
Site description and experimental setup
The CLIMAITE experiment is a temperate mixed grass- and shrubland on nutrient poor, sandy soil located in Denmark (55°53′N, 11°58′E). The mean annual temperature at the site is 8.5 °C and the annual precipitation averages 600 mm. The soil consists of 71.5 % sand, 20.5 % coarse sand and 2.2 % clay (Nielsen et al. 2009) and the average pH in the top soil (0–5 cm) is 4.9. The perennial grass Deschampsia flexuosa (L.) and the evergreen dwarf shrub Calluna vulgaris (L.) dominate the dense vegetation, which also includes a scattered distribution of mosses and herbs.
The climate change experiment was initiated in October 2005 with the intent to simulate the climate change scenario for Denmark within the next 70 years. The global change treatments were elevated atmospheric CO2 (CO2), increased temperature (T) and extended drought (D). All treatment combinations were included in the experiment: CO2 and drought (DCO2), CO2 and temperature (TCO2), temperature and drought (TD) as well as CO2, temperature and drought (TDCO2). This resulted in seven treatments and an ambient untreated reference plot (A) all with six replicates, giving a total of 48 plots (Fig. S1a).
The experimental setup and climate change manipulations have previously been described by Mikkelsen et al. (2008). In brief, the atmospheric CO2 was elevated by “free air CO2 enrichment” (FACE ) technique from 380 ppm to 510 ppm, whereas temperature was increased by passive night-time warming by 0.60 ± 0.01 °C at the soil surface (Fig. S2b). The experimental drought period was induced by curtains to exclude all precipitation during late spring and early summer each year since 2006 (Mikkelsen et al. 2008) and was continued for four to 5 weeks until the soil water content was below 0.05 m3 m−3 in the top 20 cm (Fig. S2a). In 2011, the drought period was from the 2nd of May to 3rd of June (Fig. S2b).
Litterbag experiment
Dead standing litter of C. vulgaris (leaves and branches) and D. flexuosa (leaves) were collected at the field site in early spring, but outside of the treated areas in order to provide similar litter to all litter bags. The litter was dried at 70 °C, put in a sieve and dipped in 70 % ethanol (2–5 s) to surface sterilize the litter. The sterilization enables us to test if differences in the fungal communities under the different treatments will influence litter decomposition rates. Immediately thereafter the litter was washed four times in double demineralized water (ddH2O) and finally re-dried at 70 °C. Because the relative mass of C. vulgaris is higher than that of D. flexuosa due to e.g. lignified branches, the litter was mixed based on volume in a 1:1 ratio, which corresponded to the even distribution of the plants at the experimental site, using two 5 L glass jars. After mixing, the litter was fragmented to fit into litterbags of 13 × 13 cm. The mesh size of the bags was 50 μm, which allowed in-growth of fungal hyphae, but not of plant roots and each litterbag consisted of 4–5 g dry litter. Because it rained 18.2 mm previously the same day as the litterbag incubation started (Fig. S2a), the litterbags were rewetted before they were placed in the litter layer to reduce the moisture gradient between the litter inside the litterbags and the litter in the plots. Three litterbags were incubated in each plot for 6 months (July 2011 to January 2012) underneath a mixed plant community of the two dominant species C. vulgaris and D. flexuosa where we presume UV radiation to have a negligible effect on litter degradation rates (Fig. S1b). Also, since the litterbags were incubated in the litter layer on top of the soil, the litterbags had not been exposed to the mineral soil and did not contain visible soil particles, we did not use ash-correction of the collected litter (Harmon et al. 1999).
After collecting the litterbags, the litter was weighed, and put into 119 ml air tight glass flasks, which were stored in a cooling room at 5 °C overnight, where after the CO2 respiration was measured to assess the microbial activity in the litterbag after 6 months incubation. The CO2 respiration measurements were done at 5 °C which corresponded to the air temperature in the field at the sampling day. Headspace samples (3 ml) were taken with a syringe from each flask at 0 h, 3 h and 6 h and transferred to 3-ml vials (Exetainer, Labco, Ceredigion, UK) from which the CO2 concentration was measured within 5 days on a gas chromatograph (HP 5890, Agilent, Santa Clara, CA, USA). Afterwards the contents of the three litterbags from each plot were combined and homogenized and subsamples were taken for water content determination, DNA analyses, and nutrient analyses.
Litter analyses
Total litter C and N were determined on freeze-dried litter using a LECO TrueSpec™ CN analyzer (LECO Corporation, St. Joseph, MI, USA). Total soil P was analyzed by digesting 100 mg freeze-dried litter in H2SO4 with Se as catalyst and then analyze the samples by the molybdenum blue method on a FIAstar 5000 (Jonasson et al. 1996).
A subsample of 5 g fresh litter was extracted for 1 h in 25 ml demineralised water to recover dissolved organic C (DOC). All extracts were filtered through Whatman GF-D filters and frozen until DOC was analyzed using a Shimadzu TOC-5000A total organic C analyser (Shimadzu, Kyoto, Japan).
DNA extraction, PCR and sequencing of fungal ITS
DNA was extracted from 0.5 g freeze dried litter, using a genomic mini spin kit for universal DNA isolation (A&A biotechnology, Gdynia, Poland) with a standard protocol (Yu and Mohn 1999).
The primers gITS7 (Ihrmark et al. 2012) and ITS4 (White et al. 1990) were used to amplify the ITS2 region of the fungal rDNA, and the PCR amplifications were performed in two steps. The first step was done using illustra™ puReTaq Ready-To-Go PCR Beads (GE Healthcare, United Kingdom) only added primers (300 nM gITS7 and ITS4) and template DNA (1 μl). The PCR conditions for the fungal ITS primers were 95 °C for 2 min; 30 cycles of 95 °C for 30 s, 56 °C for 30 s, 72 °C for 30 s, followed by 72 °C for 5 min. After the PCR amplifications, the samples were kept at 70 °C for 3 min and then placed on ice. Thereafter the PCR products were separated on a 1 % agarose gel followed by gel purification by the Montage Gel extraction kit (Millipore, Billerica, MA, USA). The second PCR step was done using primers with tags and adapters and each 30 μl reaction contained 5 μl of 5 × Phusion HF Buffer, 1 μl of dNTP mix, tagged ITS4 primer (final concentration 600 nM), gIT7 primer (final concentration 300 nM), 0.25 μl DNA polymerase (Phusion Hot Start DNA polymerase (Finnzymes Oy, Espoo, Finland)) and 1 μl template DNA. The conditions for this PCR were 98 °C for 30 s; 12 cycles of 98 °C for 5 s, 56 °C for 20 s, 72 °C for 20 s followed by 72 °C for 5 min.
After separating the tagged PCR products on a gel and purifying them as above, the concentration of PCR products from all tagged samples were measured by Qubit® Fluorometer (Thermo Fisher Scientific, Waltham, MA, USA) and pooled for sequencing on a GS FLX titanium platform (Roche).
Real-time quantitative PCR
Fungal abundance in the litterbags was quantified using 20 μL SYBR Green reactions on Mx 3000 (Stratagene, Cedar Creek, Texas) and the quantitative PCR (qPCR) targeted the ITS2 region by the fungal primers fITS9 (Ihrmark et al. 2012) and ITS4 (White et al. 1990). All qPCR reactions were run in technical duplicates and contained 10 μL of Brilliant SYBR Green III QPCR Master Mix (Stratagene, Cedar Creek, TX, USA), 1 μL forward primer (final concentration 385 nM), 1 μL reverse primer (final concentration 385 nM), 1 μL template DNA, and 7 μL ddH2O. The qPCR program combined the annealing and extension step (Fierer et al. 2005): 95 °C for 3 min followed by 40 cycles of 95 °C for 10 s, 60 °C for 20 s, and a final dissociation curve. The standard curve was generated from a plasmid containing ITS amplified from Pilidium concavum (Desm.) Hoehm.
DNA sequence analyses
The fungal ITS-sequences were processed using the bioinformatic pipeline SCATA (http://scata.mykopat.slu.se). Sequences were trimmed and filtered based on an average read quality of 20 and a minimum sequence length of 150 bp, and sequences missing one of the ITS primers were removed. The sequences were clustered into operational taxonomic units (OTUs) corresponding to species level (1.5 % distance in single-linkage clustering) and all singletons were removed, because they have been shown to contain a high number of technical artefacts (Unterseher et al. 2011). Afterwards an evenly sampled OTU table was generated by randomly picking 930 sequences from each sample, which was the highest subsample size in which all samples could still be present. The final data set consists of 321 fungal OTUs. For identification of the OTUs, representative sequences from each cluster were compared to reference databases; the UNITE database (Abarenkov et al. 2010) and NCBI’s DNA sequence database using the BLASTn algorithm.
Statistical analyses
All data distributions were visually checked for variance homogeneity based on residual plots and where needed the data were log-transformed. We used the PROC MIXED procedure (a mixed analysis of variance (ANOVA)) in SAS 9.3 (SAS Institute Inc., Cary, North Carolina, USA) to test the effects of elevated CO2, temperature and drought and their interactions (all assigned as fixed effects) on litter, CO2 respiration and fungi. Block and octagon (Fig. S1a) were set as random factors to account for the spatial variation at the experimental site. All non-significant terms with P-values above 0.25 were successively removed from the models, starting with the terms with the highest P-value, to the extent that it improved the model. All significant (P < 0.05) values are reported in figures and tables.
The treatment effects on the fungal community composition were analyzed by principal component analysis (PCA) of the relative abundance of fungal species (OTUs) in the litterbags after incubation. The PCA was performed in CANOCO (Microcomputer Power, Ithaca, New York, USA) and included all 48 plots and 351 fungal OTUs.
Accession numbers
The fungal ITS sequences have been deposited in MG-RAST with accession number 9969.
Results
The litterbags were incubated from July to January and therefore not during the period of the experimental pre-summer drought treatment (Fig. S2a) and at the time of sampling, there was no significant difference in the volumetric water content in the litterbags between the global change manipulations (Table 1).
Litter decomposition and microbial CO2 respiration
After incubation for 6 months the percentage of litter mass loss was 47.7 ± 1.2 % in ambient plots (Table 1). Litter mass loss was highest in litterbags that were incubated in drought plots (F = 4.94, P < 0.05), but less pronounced when combined with elevated CO2 (D x CO2 interaction F = 8.36, P < 0.01). In accordance, litterbags from the drought plots had the highest CO2 release (F = 8.93, P < 0.01) (Table 1), thus showing that the largest respiration was from litterbags with the highest decomposition (Fig. 1). Increased temperature and elevated CO2 did not affect litter decomposition and microbial CO2 respiration (Table 1).
Relationship between CO2 respiration rates and litter loss (%) after 6 months of incubation of litterbags at these global change treatments: A ambient, CO2 elevated CO2 concentration, T increased temperature, D extended drought, and combinations in a complete factorial design. Error bars indicate standard error of the mean (n = 6)
Litter C, N and P content
The concentrations of total C and dissolved organic C in litter after 6 months of incubation were not affected by the global change manipulations (Table 1). Likewise, there was no change in the concentration of total N and the C:N ratio of the remaining litter did not differ between the global change manipulations (Fig. 2c and d). The total P concentration (mg P g−1 litter) tended to decrease when litterbags were incubated in drought plots (F = 4.03, P < 0.05), and this effect was even more pronounced when drought and elevated CO2 were combined (F = 9.72, P < 0.01) (Fig. 2b).
Abundance of fungal ITS copies (a), total litter content of phosphorus (b), nitrogen (c) and the litter C to N ratio (d) in litterbags after 6 months of incubation at the following global change treatments: A ambient, CO2 elevated CO2 concentration, T increased temperature, D extended drought, and combinations in a complete factorial design. The columns are mean ± SE and the statistical significant effects of the treatments (mixed ANOVA) are indicated: *P < 0.05; **P < 0.01
Fungal community
In agreement with litter loss and microbial CO2 respiration the abundance of fungal ITS sequences were higher in litterbags incubated in drought plots (F = 5.45, P < 0.05, Fig. 2a), although there was no significant correlation between fungal ITS copy number and litter mass loss (Fig. S3). Furthermore, the fungal community composition in litterbags from the drought plots was not distinguishable from other treatments (Fig. 3) and taken together, the principal component analysis (PCA) did not show any clear correlation between overall fungal species composition and the global change manipulations (Fig. 3).
Principal component analysis (PCA) of the fungal community composition in the litterbags after 6 months of incubation. The size of the symbols is scaled based on the Shannon-Wiener index. The incubation period was from July 2011 until January 2012 and the treatments illustrated in the plot where: A ambient, CO2 elevated CO2 concentration, T increased temperature, D extended drought, combinations in a complete factorial design. The first axis (12.2 %) and the second axis (9.9 %) explained 22.1 % of the variation in the abundance of fungal OTUs
After the litterbag incubation, the fungal community was largely dominated by Ascomycetes followed by Basidiomycetes and Zygomycetes (Table 2). Elevated CO2 further decreased the relative abundance of Basidiomycetes (F = 4.69, P < 0.05) relative to Ascomycetes (F = 6.34, P < 0.05) in the litterbags (Table 2), which was likely a result of a higher relative abundance of especially Hypocreales (F = 9.57, P < 0.01, Table 2), an order mostly dominated by Trichoderma and Fusarium species (Table S1). Litterbags incubated in the drought plots had an increased relative abundance of Sphaerobolus species (Geastrales) (F = 4.13, P < 0.05, Table 2) (Table S1), whereas Coniochaetales species decreased (F = 5.70, P < 0.05, Table 2).
Discussion
Climate effects on litter decomposition
In this field experiment, we investigated effects on short-term litter decomposition rates and the associated fungal community following 6 years of global change treatments. We were surprised to find that the legacy effects of pre-summer drought were more pronounced than either warming or elevated CO2 treatments. The increased litter decomposition rates (except when combined with elevated CO2) and higher CO2 respiration rates from the litterbags in plots previously subjected to the drought treatment contrasted direct drought effects on litter decomposition in similar ecosystems (Emmett et al. 2004; van Meeteren et al. 2008).
Extended pre-summer drought has previously been shown to reduce litter decomposition at our experimental site (Andresen et al. 2010). In that study, litterbags were incubated for six and 12 months after the global change manipulations had been running for 2 years, whereas after 5 years of treatments significantly more ITS copies in drought treated soils compared to soils in the ambient control plots (Haugwitz et al. 2014). This was further supported by the present study, where we found an increased abundance of fungal ITS copies in the incubated litterbags from the drought plots. Also, van Meeteren et al. (2008) found an increased microbial biomass C in litterbags in drought treated plots at a Dutch heathland, but that was concurrent with a decrease in litter decomposition rates.
Our litterbags exclude animals larger than 50 μm. Thus, degradation of the litter is related to the activity of fungi and microfauna (and maybe to a small extent to bacteria) and not to the enchytraeids, mites and springtails present in the belowground food web at the study site (Andresen et al. 2011). Springtails and mites are important grazers of fungi and enhance litter degradation by contributing to fragmentation of the litter (Seastedt 1984; Faber 1991). However, arthropods are not prone to desiccation (Manzoni et al. 2012; Holmstrup et al. 2013). In contrast drought has been found to decrease the adundance of enchytraeids at our study site (Maraldo et al. 2010), although the enchytraeid communities recovered within 2 months of the pre-summer treatment (Maraldo and Holmstrup 2009). Thus, the higher litter degradation rates caused by a higher abundance of fungi in the litterbags in the drought plots are not likely due to a decreased grazing of the fungal biomass.
Increased temperature did not significantly affect litter decomposition, which was in contrast with our initial expectations (H1) and with previous studies where litter decomposition is stimulated by warming (van Meeteren et al. 2008; Bontti et al. 2009; Butenschoen et al. 2011; Incerti et al. 2011). Similar to this result, there was no effect of elevated CO2 on litter decomposition and H2 was therefore rejected.
Global change effects on litter-associated fungal community
In contrast to litter decomposition rates, the community structure of fungal species (OTUs) remained unchanged after 6 years of global change treatments compared to the ambient plots thus rejecting H3 (Fig. 3). Since the litter quality in the litterbags is the same in all plots, the faster litter decomposition rates in drought-treated plots suggest that the litter colonizing fungi have adapted to drier conditions. Even though litter-associated microorganisms in general have a lower water-stress threshold compared to soil microorganisms (Manzoni et al. 2012), drought-resistant fungi have been found to control organic matter decomposition in Mediterranean ecosystems with experimental exclusion of precipitation (Yuste et al. 2011), thus supporting our suggestion.
Despite no clear changes in the fungal community composition were evident as a result of global change manipulations, we did find a higher overall abundance of the order Geatrales in the litterbags from the drought-treated plots (Table 2). The order consisted of Sphaerobolus species and the most dominant of these species was identified as Sphaerobolus stellatus, which has been shown to decompose lignin (Robinson et al. 1993). Thus, the increased abundance of S. stellatus could explain the higher decomposition in the litterbags in the drought-treated plots, although the species was not big enough to show clear treatment effects on the overall community structure.
At phylum level, the fungal community composition in the litterbags was not affected by drought or increased temperature, but by elevated CO2 resulting in a relative higher abundance of Ascomycetes compared to Basidiomycetes (Table 2). It is generally accepted that the early stages of litter decomposition are dominated by Ascomycetes with Basidiomycetes dominating later (Frankland 1998) and this finding could thus indicate that elevated CO2 may have changed the rate of succession of litter-degrading fungi. A change that could have long-term effects on litter decomposition in the ecosystem, since Ascomycetes have a lower litter-decomposing capacity compared to Basidiomycetes (Osono and Takeda 2002).
Consequences of future climate on litter decomposition
Fungi are the main contributor to decomposition in this ecosystem (Haugwitz et al. 2014), both because fungi are better adapted to low soil pH and they cope better with extended drought periods than, for example, bacteria (Frey et al. 1999; Khalvati et al. 2005; Yuste et al. 2011; de Vries et al. 2012; Manzoni et al. 2012; Barnard et al. 2013). In this study, 6 years of extended pre-summer drought increased the short-term litter decomposition after the drought treatment ended, although the community structure of litter-decomposing fungi remained intact. Hence, we suggest that the legacy effect of drought was mediated by drought-adaptation of the fungal community or smaller changes in the distribution of less frequent fungal species.
Grass- and shrub-dominated ecosystems are widespread and a large fraction of these is expected to experience decreased precipitation and possibly more intense drought periods in the future (Christensen and Christensen 2007; IPCC 2007; Gonzalez et al. 2010; Trenberth et al. 2014), which can reduce plant productivity (Penuelas et al. 2004). Since these ecosystems are nutrient limited, drought-induced changes in turnover of organic material to periods, where plants are less active, could potentially decrease nutrient availability during the growing season and increase the risk of nutrient loss by leaching in the winter period. Although we only analyzed the short-term litter decomposition, these findings are a valid contribution to understanding the implications of global change for grass- and shrub dominated ecosystems.
References
Abarenkov K, Nilsson RH, Larsson KH, Alexander IJ, Eberhardt U, Erland S, Høiland K, Kjøller R, Larsson E, Pennanen T, Sen R, Taylor AFS, Tedersoo L, Ursing BM, Vrålstad T, Liimatainen K, Peintner U, Kõljalg U (2010) The UNITE database for molecular identification of fungi - recent updates and future perspectives. New Phytol 186:281–285
Aerts R (1997) Climate, leaf litter chemistry and leaf litter decomposition in terrestrial ecosystems: a triangular relationship. Oikos 79:439–449
Allison SD, Treseder KK (2008) Warming and drying suppress microbial activity and carbon cycling in boreal forest soils. Glob Chang Biol 14:2898–2909
Anderson IC, Drigo B, Keniry K, Ghannoum O, Chambers SM, Tissue DT, Cairney JWG (2013) Interactive effects of preindustrial, current and future atmospheric CO2 concentrations and temperature on soil fungi associated with two Eucalyptus species. FEMS Microbiol Ecol 83:425–437
Andresen LC, Michelsen A, Jonasson S, Schmidt IK, Mikkelsen TN, Ambus P, Beier C (2010) Plant nutrient mobilization in temperate heathland responds to elevated CO2, temperature and drought. Plant Soil 328:381–396
Andresen LC, Konestabo HS, Maraldo K, Holmstrup M, Ambus P, Beier C, Michelsen A (2011) Organic matter flow in the food web at a temperate heath under multifactorial climate change. Rapid Commun Mass Spectrom 25:1485–1496
Barnard RL, Osborne CA, Firestone MK (2013) Responses of soil bacterial and fungal communities to extreme desiccation and rewetting. ISME J 7:2229–2241
Bontti EE, Decant JP, Munson SM, Gathany MA, Przeszlowska A, Haddix ML, Owens S, Burke IC, Parton WJ, Harmon M (2009) Litter decomposition in grasslands of Central North America (US Great Plains). Glob Chang Biol 15:1356–1363
Butenschoen O, Scheu S, Eisenhauer N (2011) Interactive effects of warming, soil humidity and plant diversity on litter decomposition and microbial activity. Soil Biol Biochem 43:1902–1907
Christensen JH, Christensen OB (2007) A summary of the PRUDENCE model projections of changes in European climate by the end of this century. Clim Chang 81:7–30
Cotrufo MF, Ineson P, Scott A (1998) Elevated CO2 reduces the nitrogen concentration of plant tissues. Glob Chang Biol 4:43–54
Couteaux MM, Bottner P, Berg B (1995) Litter decomposition, climate and litter quality. Trends Ecol Evol 10:63–66
de Boer W, Folman LB, Summerbell RC, Boddy L (2005) Living in a fungal world: impact of fungi on soil bacterial niche development. FEMS Microbiol Rev 29:795–811
de Vries FT, Liiri ME, Bjørnlund L, Bowker MA, Christensen S, Setälä HM, Bardgett RD (2012) Land use alters the resistance and resilience of soil food webs to drought. Nat Clim Chang 2:276–280
Drigo B, Kowalchuk GA, van Veen JA (2008) Climate change goes underground: effects of elevated atmospheric CO2 on microbial community structure and activities in the rhizosphere. Biol Fertil Soils 44:667–679
Emmett BA, Beier C, Estiarte M, Tietema A, Kristensen HL, Williams D, Peñuelas J, Schmidt I, Sowerby A (2004) The response of soil processes to climate change: results from manipulation studies of shrublands across an environmental gradient. Ecosystems 7:625–637
Faber JH (1991) Functional classification of soil fauna: a new approach. Oikos 62:110–117
Fierer N, Jackson JA, Vilgalys R, Jackson RB (2005) Assessment of soil microbial community structure by use of taxon-specific quantitative PCR assays. Appl Environ Microbiol 71:4117–4120
Frankland JC (1998) Fungal succession — unravelling the unpredictable. Mycol Res 102:1–15
Frey SD, Elliott ET, Paustian K (1999) Bacterial and fungal abundance and biomass in conventional and no-tillage agroecosystems along two climatic gradients. Soil Biol Biochem 31:573–585
Gonzalez P, Neilson RP, Lenihan JM, Drapek RJ (2010) Global patterns in the vulnerability of ecosystems to vegetation shifts due to climate change. Glob Ecol Biogeogr 19:755–768
Harmon ME, Nadelhoffer KJ, Blair JM (1999) Measuring decomposition, nutrient turnover, and stores in plant litter. In: Robertson GP, Coleman DC, Bledsoe CS, Sollins P (eds) Standard soil methods for long-term ecological research. Oxford University Press, Oxford, United Kingdom, pp. 202–240
Haugwitz MS, Bergmark L, Priemé A, Christensen S, Beier C, Michelsen A (2014) Soil microorganisms respond to five years of climate change manipulations and elevated atmospheric CO2 in a temperate heath ecosystem. Plant Soil 374:211–222
Holmstrup M, Sørensen JG, Schmidt IK, Nielsen PL, Masonc S, Tietemac A, Smith AR, Bataillon T, Beier C, Ehlers BK (2013) Soil microarthropods are only weakly impacted after 13 years of repeated drought treatment in wet and dry heathland soils. Soil Biol Biochem 66:110–118
Ihrmark K, Bödeker ITM, Cruz-Martinez K, Friberg H, Kubartova A, Schenck J, Strid Y, Stenlid J, Brandström-Durling M, Clemmensen KE, Lindahl BD (2012) New primers to amplify the fungal ITS2 region – evaluation by 454-sequencing of artificial and natural communities. FEMS Microbiol Ecol 82:666–677
Incerti G, Bonanomi G, Giannino F, Rutigliano FA, Piermatteo D, Castaldi S, De Marco A, Fierro A, Fioretto A, Maggi O, Papa S, Persiani AM, Feoli E, De Santo AV, Mazzoleni S (2011) Litter decomposition in Mediterranean ecosystems: Modelling the controlling role of climatic conditions and litter quality. Appl Soil Ecol 49:148–157
IPCC (2007) Climate change 2007: the physical science basis. In: Solomon S, Qin D, Manning M, et al. (eds) Climate change 2007: impacts, adaptation and vulnerability. Contribution of Working Group II to the Fourth Assessment Report of the Intergovernmental Panel on Climate Change. Cambridge University Press, Cambridge, United Kingdom
Jensen KD, Beier C, Michelsen A, Emmett BA (2003) Effects of experimental drought on microbial processes in two temperate heathlands at contrasting water conditions. Appl Soil Ecol 24:165–176
Jonasson S, Michelsen A, Schmidt IK, Nielsen EV, Callaghan TV (1996) Microbial biomass C, N and P in two arctic soils and responses to addition of NPK fertilizer and sugar: implications for plant nutrient uptake. Oecologia 106:507–515
Khalvati MA, Hu Y, Mozafar A, Schmidhalter U (2005) Quantification of water uptake by arbuscular mycorrhizal hyphae and its significance for leaf growth, water relations, and gas exchange of barley subjected to drought stress. Plant Biol (Stuttgart) 7:706–712
Kjøller A, Struwe S (1982) Microfungi in ecosystems - fungal occurrence and activity in litter and soil. Oikos 39:391–422
Liu LL, King JS, Giardina CP, Booker FL (2009) The influence of chemistry, production and community composition on leaf litter decomposition under elevated atmospheric CO2 and tropospheric O3 in a northern hardwood ecosystem. Ecosystems 12:401–416
Manzoni S, Schimel JP, Porporato A (2012) Responses of microbial communties to water stress: results from a meta-analysis. Ecology 93:930–938
Maraldo K, Holmstrup M (2009) Recovery of enchytraeid populations after severe drought events. Appl Soil Ecol 42:227–235
Maraldo K, Krogh PH, van der Linden L, Christensen B, Mikkelsen TN, Beier C, Holmstrup M (2010) The counteracting effects of elevated atmospheric CO2 concentrations and drought episodes: studies of enchytraeid communities in a dry heathland. Soil Biol Biochem 42:1958–1966
Mikkelsen TN, Beier C, Jonasson S, et al. (2008) Experimental design of multifactor climate change experiments with elevated CO2, warming and drought: the CLIMAITE project. Funct Ecol 22:185–195
Nielsen PL, Andresen LC, Michelsen A, Schmidt IK, Kongstad J (2009) Seasonal variations and effects of nutrient applications on N and P and microbial biomass under two temperate heathland plants. Appl Soil Ecol 42:279–287
Norby RJ, Cotrufo MF (1998) Global change - a question of litter quality. Nature 396:17–18
Norby RJ, Cotrufo MF, Ineson P, O'Neill EG, Canadell JG (2001) Elevated CO2, litter chemistry, and decomposition: a synthesis. Oecologia 127:153–165
Osono T, Takeda H (2002) Comparison of litter decomposing ability among diverse fungi in a cool temperate deciduous forest in Japan. Mycologia 94:421–427
Penuelas J, Gordon C, Llorens L, Nielsen T, Tietema A, Beier C, Bruna P, Emmett B, Estiarte M, Gorissen A (2004) Nonintrusive field experiments show different plant responses to warming and drought among sites, seasons, and species in a north-south European gradient. Ecosystems 7:598–612
Robinson CL, Dighton J, Frankland J (1993) Resource capture by interacting fungal colonizers of straw. Mycol Res 97:547–558
Sanaullah M, Rumpel C, Charrier X, Chabbi A (2012) How does drought stress influence the decomposition of plant litter with contrasting quality in a grassland ecosystem? Plant Soil 352:277–288
Seastedt TR (1984) The role of microarthropods in decomposition and mineralization processes. Annu Rev Entomol 29:25–46
Selsted MB, van der Linden L, Ibrom A, Michelsen A, Larsen KS, Pedersen JK, Mikkelsen TN, Pilegaard K, Beier C, Ambus P (2012) Soil respiration is stimulated by elevated CO2 and reduced by summer drought: three years of measurements in a multifactor ecosystem manipulation experiment in a temperate heathland (CLIMAITE). Glob Chang Biol 18:1216–1230
Toberman H, Evans CD, Freeman C, Fenner N, Artz RRE (2008) Summer drought effects upon soil and litter extracellular phenol oxidase activity and soluble carbon release in an upland Calluna heathland. Soil Biol Biochem 40:1519–1532
Trenberth KE, Dai A, van der Schrier G, Jones PD, Barichivich J, Briffa KR, Sheffield J (2014) Global warming and changes in drought. Nat Clim Chang 4:17–22
Unterseher M, Jumpponen A, Opik M, Tedersoo L, Moora M, Dormann CF, Schnittler M (2011) Species abundance distributions and richness estimations in fungal metagenomics - lessons learned from community ecology. Mol Ecol 20:275–285
van Meeteren MM, Tietema A, van Loon EE, Verstraten JM (2008) Microbial dynamics and litter decomposition under a changed climate in a Dutch heathland. Appl Soil Ecol 38:119–127
White TJ, Bruns TD, Lee SB, Taylor JW (1990) Amplification and direct sequencing of fungal ribosomal RNA genes for phylogenetics. In: Gelfand DH, Sninsky JJ, White TJ (eds) Innis MA. PCR Protocols - a Guide to Methods and Applications. Academic Press, San Diego, CA, USA, pp. 315–322
Yu ZT, Mohn WW (1999) Killing two birds with one stone: simultaneous extraction of DNA and RNA from activated sludge biomass. Can J Microbiol 45:269–272
Yuste JC, Penuelas J, Estiarte M, Garcia-Mas J, Mattana S, Ogaya R, Pujol M, Sardans J (2011) Drought-resistant fungi control soil organic matter decomposition and its response to temperature. Glob Chang Biol 17:1475–1486
Zhang W, Parker KM, Luo Y, Wan S, Wallace LL, Hu S (2005) Soil microbial responses to experimental warming and clipping in a tallgrass prairie. Glob Chang Biol 11:266–277
Acknowledgments
This study was carried out within the CLIMAITE project, financially supported by the Villum Kann Rasmussen Foundation. We thank Karin Vestberg, Esben V. Nielsen and Anette Hørdum Løth for excellent technical assistance.
Author information
Authors and Affiliations
Corresponding author
Additional information
Responsible Editor: Duncan D. Cameron.
Electronic supplementary material
ESM 1
(DOCX 15 kb)
ESM 2
Fig. S1 The CLIMAITE experimental site; a) A schematic of the global change treatments with each block consisting of two octagons: one with ambient CO2 and one with elevated CO2. Each octagon includes the treatments drought (−H2O), warming (+T) and the combination plus either an ambient “control plot” or an elevated CO2 plot. In total, there are 12 octagons at the experimental site giving 48 plots. b) Picture of the vegetation density at the CLIMAITE experimental site in October 2011. The dominant plant species are Calluna vulgaris and Deschampsia flexuoxa. (PNG 21290 kb)
ESM 3
Fig. S2 Weekly averages of climatic data from 2011 at the CLIMAITE experimental site; a) Soil water content (vol.% in 0–20 cm soil depth) in plots without pre-summer drought treatment (filled triangles) and plots with pre-summer drought treatment (open triangles). Daily precipitation (mm) is shown in light-grey bars; b) Soil surface temperature in plots without temperature treatment (filled circles) and plots with temperature treatment (open circles). (GIF 64 kb)
ESM 4
Fig. S3 The relationship between litter loss (%) and fungal ITS copy number (g−1 soil) after six months of incubation of litterbags in the global change experiment CLIMIATE. Each filled circle represents one sample (n = 48). (GIF 15 kb)
Rights and permissions
About this article
Cite this article
Haugwitz, M.S., Michelsen, A. & Priemé, A. The legacy of climate change effects: previous drought increases short-term litter decomposition rates in a temperate mixed grass- and shrubland. Plant Soil 408, 183–193 (2016). https://doi.org/10.1007/s11104-016-2913-2
Received:
Accepted:
Published:
Issue Date:
DOI: https://doi.org/10.1007/s11104-016-2913-2