Abstract
Single and double-labeling immunocytochemistry has been used to learn about the localization, distribution, and possible relationship between beta-amyloid protein (Aβ) deposition and tau hyperphosphorylation in the canine cerebral cortex with age. Behavioral impairment, as reported by the owners and tested in all dogs, correlated with increased Aβ burden in old dogs. Aβ plaques were diffuse and they were not accompanied by modifications in synaptic protein expression. Plaques were not associated with increased active mitogen activated protein kinase (MAPK/ERK-P) and p38 kinase (p38-P) expression, and tau hyperphosphorylation in neighboring cell processes. Yet tau hyperphosphorylation, as revealed with phospho-specific antibodies to tauThr181 and tauSer396, increased with age in individual neurons. Moreover, the subcellular pattern shifted from perinuclear localization to granular cytoplasmic and nuclear distribution with age. Our results in dog suggest that Aβ diffuse plaque formation and tau hyperphosphorylation are independent events, both occurring during the process of aging. Although increased cognitive dysfunction is associated with increased tau hyperphosphorylation, further investigation is needed to understand whether tau hyperphosphorylation is causative of cognitive impairment or an independent process related to aging.
Similar content being viewed by others
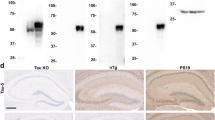
Avoid common mistakes on your manuscript.
Introduction
The principal hallmarks of Alzheimer’s disease (AD) are the formation and deposition of beta-amyloid protein (Aβ) in the form of senile plaques (diffuse and neuritic plaques) and cerebral amyloid angiopathy, and the hyperphosphorylation and fibrillization of tau, which comprises neurofibrillary tangles (NFTs), neuropil threads, and dystrophic neurites of neuritic plaques [15, 65]. Combined clinical and neuropathological studies in AD have shown a strong relationship between cognitive impairment and load of neuropathological hallmarks [12]. In the same line, behavioral deficits correlate with age-related changes described in the brain of old dogs [3, 8, 10, 11, 14, 48]. Aged dogs spontaneously developed Aβ diffuse plaques that are neuropathologically and sequentially identical to those observed in humans [30]. The deposition of the Aβ protein is widely distributed in cortex and hippocampus, but the brain stem and cerebellum are usually spared [29]. Yet amyloid plaques in dogs are only diffuse plaques that do not fulfill the β-pleated-sheet conformation and are Congo red and thioflavine negative, with no apparent neuritic component [8, 14, 48, 57]. In humans, diffuse plaques are also encountered in Down’s syndrome [22, 27], in normal aging [31, 38], and in response to trauma [42, 47, 53], in addition to AD.
Despite the similarity of Aβ deposition and cognitive decline, development of NFTs is markedly different between canine and human brain. In human tissue, NFTs are mainly composed of hyperphosphorylated tau that aggregates as paired helical filaments (PHFs). In addition to NFTs, hyperphosphorylated tau is found in neuropil threads and in dystrophic neurites surrounding senile plaques [1, 32]. Increased expression of several kinases including p38 kinase (p38), cyclin-dependent kinase-5 (CDK-5), glycogen synthase kinase-3β (GSK-3β), mitogen-activated protein kinase, and extracellular signal-regulated kinase (MAPK/ERK1-2) has been implicated in tau hyperphosphorylation with different efficiencies for particular sites, and formation of PHFs [18, 19, 25, 45, 46]. Thus, phosphorylation of tau at Thr181 and at Ser396 can be conveyed by MAPK/ERK, stress-activated protein kinases c-Jun N-terminal kinase (SAPK/JNK), p38 or GSK-3β, whereas at Tau Ser202 phosphorylation may be due to MAPK/ERK, SAPK/JNK, p38 or GSK-3α/β [2]. Neurofibrillary changes have been described, in the absence of Aβ deposition, in nonmammalian models [61], and in aged nonhuman primates [59], ruminants [5], polar bears and cats [9, 24, 52].
Phosphorylation at the tau-1 site—amino acid residues 189 and 207 of the human tau sequence [21]—takes place in several neuronal populations and oligodendrocytes in dogs [64]. However, no transition from normal specific tau phosphorylation to excessive phosphorylation leading to cell degeneration and NFT formation has been detected in dogs [10, 44].
The present study examines the relationship among Aβ deposition, tau phosphorylation, and synaptic protein expression in the vicinity of cerebral Aβ plaques in aged dogs with clinically verified progressive cognitive deficits. This study is geared to increasing our understanding of the neuropathological bases of cognitive deficits in old dogs.
Materials and methods
Dog samples
The study was performed on the brains of ten dogs, seven males and three females, of various breeds and from 1 to 20 years of age, all certified by their medical records from the veterinary hospital Ars Veterinaria of Barcelona, Spain (Table 1). In addition to the clinical history, the cognitive status of every dog was evaluated using a cognitive test with the aid of the pet’s owner [49]. The final total score reflected the cognitive status of the animal. In all cases, brain donation was formally approved by the owner and euthanasia justified for medical reasons; the animals were euthanized with an intravenous overdose of sodium thiopental (75 mg/kg) (Thiobarbital, Braun Medical S.A., Spain). All animals were treated according to European legislation on animal handling and experiments (86/609/EU) and procedures were approved by the Ethical Committee of the University of Barcelona, Barcelona, Spain. All efforts were made to minimize animal suffering and to use no more than the number of animals needed for reliable scientific data.
Tissue preparation
The tissue preparation was adapted from human brain bank methods [34]. Immediately after death, the brains were rapidly removed from the skull and 1-cm-thick coronal sections of cerebral cortex were immersion-fixed in 10% neutral buffered formalin for 2 weeks. After a 3-day cryoprotection, they were frozen on powdered dry ice and stored at −40°C until use.
Immunohistochemistry
Serial consecutive 40-μm-thick sections of the dorsal anterior prefrontal cortex corresponding to area 8a on the proreal gyrus [28] were obtained with a cryostat and processed free-floating with the streptavidin–biotin (LSAB) method (Dako LSAB + kit, Dako, Barcelona, Spain) following the instructions of the supplier. Briefly, after blocking endogenous peroxidases, the sections were incubated with normal serum for 2 h and then incubated overnight at 4°C with one of the primary antibodies. The monoclonal antibody to synaptic-associated 25,000 molecular weight protein (SNAP-25; raised against recombinant SNAP-25) and the monoclonal antibody against synaptophysin (Dako) were used at dilutions of 1:500. The phospho-specific tau Thr181, tau Ser202, and tau Ser396 polyclonal rabbit antibodies (Calbiochem, Madrid, Spain) were used at dilutions of 1:500, 1:500, and 1:100, respectively. The phospho-specificity of these antibodies was previously tested in human brain samples with AD by pre-incubation of the antibodies with alkaline phosphatase (Sigma, Madrid, Spain). Immunostaining of neurofibrillary tangles largely faded following this treatment. Similar results were obtained in the aged canine brain, after pre-incubation of the antibody with phosphatase. The immunoreaction was abolished thus indicating specificity of the antibody for phosphorylated species. The anti-MAP kinase phospho-specific (Tyr204) rabbit polyclonal antibody (MAPK/ERK-P) was used at a dilution of 1:200. The phosphorylation-dependent rabbit polyclonal antibody to p38(Thr180/Tr182) (p38-P) (Calbiochem) was used at a dilution of 1:100. For Aβ immunohistochemistry, a slight variation of the protocol was introduced. Before blocking endogenous peroxidases, the sections were incubated with 98% formic acid for 3 min to enhance antigenicity. The Aβ8–17 mouse monoclonal antibody (Dako) was used at a dilution of 1:50. After washing, the sections were incubated with LSAB for 15 min each at room temperature. The peroxidase reaction was visualized, as a dark blue precipitate, with NH4NiSO4 (0.05 M) in phosphate buffer (0.1 M), 0.05% diaminobenzidine, NH4Cl and 0.01% hydrogen peroxide, or as a brown precipitate with 3,3′-diaminobenzidine and hydrogen peroxide. Finally, the sections were dehydrated and mounted with DPX (Scharlau Chemie, Barcelona, Spain). Blank sections stained only with the secondary antibodies were used as negative controls.
Double-labeling immunofluorescence and confocal microscopy
Cryostat sections, 40 μm thick, were rinsed in PBS and incubated with 98% formic acid for 3 min. After that, they were mounted on glass slices and stained with a saturated solution of Sudan black B (Merck, Madrid, Spain) for 10 min to block the autofluorescence of lipofucsin granules present in nerve cell bodies, rinsed in 70% ethanol, and washed in distilled water. The sections were incubated in a blocking solution containing 0.2% gelatine, 0.2% azide, 0.2% Triton X-100, and 20% fetal bovine serum in PBS, pH 7.5 for 1 h at room temperature. Immediately afterwards, the sections were incubated at 4°C overnight with the Aβ1–42 rabbit polyclonal antibody (kindly provided by Dr. M. Sarasa, Zaragoza, Spain) [50] at a dilution of 1:500 and with monoclonal antibody against synaptophysin (Dako) (1:100) in a vehicle solution composed of 0.2% gelatine, 0.2% azide, 0.2% Triton X-100, and 1% fetal bovine serum in PBS, pH 7.5. Other sections were incubated with the Aβ8–17 monoclonal antibody at a dilution of 1:50 and with one of the following rabbit polyclonal antibodies against SNAP-25 (1:500), phospho-specific tau Thr181 (1:500), tau Ser202 (1:100), or Ser396 (1:100) (Calbiochem). Finally, other sections were double-stained for amyloid and anti-MAPK/ERK-P (1:200), or anti-p38-P (1:100) (Calbiochem), in the same vehicle solution. After washing in PBS, the sections were incubated in the dark with a cocktail of secondary antibodies and diluted in the same vehicle solution as the primary antibodies for 45 min at room temperature. Secondary antibodies were Alexa488 antirabbit and Alexa546 antimouse (both from Molecular Probes, Madrid, Spain); these were used at a dilution of 1:400. Sections only with the cocktail of secondary antibodies were used as controls. Finally, TO-PRO-3 (Invitrogen Life Technologies, Barcelona, Spain) was used to detect the cell nuclei. After that, sections were mounted in Immuno-Fluore Mounting medium (ICN Biomedicals, Barcelona, Spain), sealed and dried overnight. Sections were examined with a Leica TCS-SL confocal microscope. For each marker, two sections per animal were evaluated for total neuronal staining intensity, and rankings were done by one investigator (MP) blind with respect to the age of the animal.
Bielschowsky staining
The Bielschowsky staining method was performed according to the Yamamoto and Hirano modification using 20% silver nitrate [68]. Briefly, 40-μm-thick sections were washed in distilled water and placed in 20% silver nitrate in the dark at 37°C for 30 min. After that, strong ammonia was added to the silver nitrate solution and the sections were left in this solution for 10 min at 37°C. Then the sections were washed in 0.1% ammonia and 6–10 drops of developer solution (10% formalin, distilled water, concentrated nitric acid and citric acid) were added to silver hydroxide solution and the sections were stained for 3–5 min. After that, the sections were toned in 0.2% gold chloride for 1–2 min and fixed in 5% sodium thiosulphate for 1 min. Finally, the sections were washed, dehydrated, and mounted in DPX (Scharlau Chemie).
Results
Based on the presence of established housetraining habits, disorientation in familiar surroundings, decreased activity, playfulness, vitality, decreased interaction with the owner, and modifications of the sleep/awake cycle, dogs were categorized as young control (YC), i.e. with no signs of behavioral disorder, light cognitive deficits (LCD), and severe cognitive deficits (SCD) (Table 1).
Starting at the age of 8 years, and increasing with age and with cognitive deficit severity, Aβ immunohistochemistry revealed the presence of delicate (Fig. 1a, c) and more compact (Fig. 1b, d) diffuse deposits throughout all cortical layers of the cerebral cortex in a characteristic four-stage distribution (I–IV) (Table 1) [48, 57]. No plaques were observed in the subcortical white matter. The Aβ plaques were not stained with antibodies to the synaptic proteins synaptophysin and SNAP-25 (data not shown). Increasing with age from moderate (+) to strong (+++++), immunoreactivity with phosphorylation-dependent tau antibodies Thr181 and Ser396 was found in all cases (Table 1). In young animals, the tau Thr181 and tau Ser396 immunoreactivity was observed in sparse neurons characterized by a widespread distribution within all the different cortical layers. Labeling was localized in the perinuclear cytoplasm and the nuclear membrane of some neurons (Figs. 2a, b, 3a). Yet increased tau Thr181 and tau Ser396 immunoreactivity in all the cortical layers was localized in the cytoplasm and, rarely, in the nuclear membrane in dogs aged between 8 and 20 years (Figs. 2c, 3b). No positivity was noticed with antitau antibodies Ser202.
Representative microphotographs of Aβ immunoreactivity in the prefrontal cortex of two dogs visualized with anti-Aβ8–17 antibody (a–d). a, c: In stage II, Aβ deposition was localized in the deep layers of the cortex (V–VI) and showed a diffuse and cloud-like aspect (Animal no. 5, 10 year old). b, d: In stage IV, progressively more compact Aβ deposits extended throughout all cortical layers (Animal no. 9, 16 year old). a, b bar = 50 μm; c, d bar = 200 μm
Confocal images of progressive increased tau Thr181 immunoreactivity with age, without (a–c) and with Aβ deposition (d–f) in prefrontal cortex of dogs. a Dog no. 1 (1 year old) presented light tau Thr181 immunofluorescence (green) showing a granular pattern in the perinuclear cytoplasm and in the nuclear membrane. b In dog no. 4 (8 year old), the number of tau Thr181-immunoreactive neurons increased and the granular aspect became more evident. c In old dogs (in this example, dog no. 10, 20 year old), tau Thr181 immunofluorescence was very strong and the granular precipitate was localized mainly in the nucleus in cortical regions without Aβ deposition. d–f The same dog with strong tau Thr181 immunoreactivity (green) and Aβ deposition (Aβ8–17, red). In merge (f), no relationship between tau Thr181 and Aβ deposition was found. No positive neurites surrounding plaque were detected. The sections were counterstained with TO-PRO-3 (blue). a bar = 20 μm, b–f bar = 16 μm
Confocal images of progressive increased tau Ser396 immunoreactivity in aged dogs. a Dog no. 3 (6 year old) presented a light pattern of tau Ser396 (green) immunoreactivity mainly localized in the perinuclear region. The nuclei are visualized with TO-PRO-3 (blue). b–d Strong tau Ser396 immunofluorescence found in dog no. 8 (16 year old). Panel b corresponds to phospho-tau Ser396 only (green), c represents only Aβ8–17 deposition (red), and d is merged. All sections were counterstained with TO-PRO-3 (blue). e, f Regional distribution of tau Ser396 staining in dog no. 8 (16 year old). Tau Ser396 immunoreactivity (green) was observed throughout all the cortical layers in region without amyloid deposition. The nuclei are visualized with TO-PRO-3 (blue) (e). When amyloid deposition was present, no apparent association between tau Ser396 phosphorylation (green) and amyloid plaque (red) was observed (f). a bar = 16 μm, b–d bar = 20 μm, e, f bar = 40 μm
In all situations, the distribution of phospho-tau immunoreactivity lacked any spatial relation with amyloid deposition. More precisely, neuronal Thr181 and Ser396 tau phosphorylation did not localize in the surroundings of diffuse plaques, thus indicating no hyperphosphorylation of tau in neurites surrounding amyloid plaques (Figs. 2d–f, 3b–d). Conversely, amyloid deposition was absent in the vicinity of neurons bearing hyperphosphorylated tau (Fig. 3e, f). Moreover, diffuse plaque maturation between stage I and IV observed in aged dogs did not parallel increased tau Thr181 and Ser396 phosphorylation in neurons (Table 1). Bielschowsky silver staining confirmed the absence of dystrophic neurites and neurofibrillary tangles in all dogs. Finally, immunoreactivity to MAPK/ERK-P and p38-P in relation with amyloid plaques was negative in every case (Table 1).
Discussion
The results of this study support previous observations regarding amyloid plaque formation and tau phosphorylation in aging canine brain. As previously reported [49], a variety of behavioral changes can be evidenced in aged dogs in the present series despite the limited number of cases studied. These alterations include disorientation in familiar surroundings, decreased activity and playfulness, altered social relationship and interaction with the owner and with other animals, decreased self-hygiene, alteration of adaptative capabilities, and modifications of the sleep/awake cycle [49]. As in humans, the severity of the cognitive deficit in dogs has been correlated with the density of the Aβ deposits in hippocampus and frontal cortex [11, 12]. In agreement with other reports, our results have shown that the aged canine brain contains predominantly diffuse plaques classified into four stages [8, 48, 57]. In this paper, we found that the first steps of Aβ deposition take place around 8 years, an age associated with high levels of oxidative stress and a dramatic drop in antioxidant defenses [23, 44] that clinically responds to behavioral enrichment and dietary fortification [36, 55]. In AD, Aβ deposition, a source of oxidative damage and oxidative stress, is considered to be a proximal event in the pathogenesis of AD [60]. A direct toxic effect of the Aβ deposition related with cognitive deficits has been demonstrated in humans and canines [11, 12]. The reduced dog life span may explain the absence of neuritic plaques, as it does not allow sufficient time for the 1–40 Aβ-fragment responsible for the cored plaque to precipitate [8, 13]. Other authors have shown that the presence of longer Aβ peptides is not central to the seeding of amyloid in vitro [67], and, therefore, the absence of neuritic plaques in dogs may be related rather to other factors such as apolipoprotein E, metal ions or specific astroglial protein expression and microglial reaction [33, 39, 54, 63, 66]. Because the cognitive impairments seen in AD seem largely related with the concomitant regional and laminar tau pathology [4, 37, 40, 41], several authors correlate the functional deterioration of aged dogs with excessive tau phosphorylation and resulting abnormalities [64]. The present study has shown that tau phosphorylation is a common process in the dog brain during aging, and that this process occurs in the absence of intraneuronal neurofibrillary changes, neuropil threads, and dystrophic neurites surrounding amyloid deposits. Some studies have reported cytoskeletal abnormalities in dogs [44, 56], but our present results and others [16] indicate that the process of neurodegeneration does not involve NFTs formation. Furthermore, in AD, abnormal synaptic protein expression occurs in human senile plaques [7, 17], and a similar situation is found in transgenic mice [26, 43, 62]. Yet no abnormal synaptic protein expression has been noticed in canine amyloid plaques.
In our study, no-housed aging dogs have been characterized under several paradigms that include in every animal, study of cognitive function, amyloid plaque stage deposition, and sites of Tau phosphorylation. As shown previously, despite the relatively small number of animals included in this study, our cognitive test is able to differentiate between three groups of canine cognitive status, that includes the LCD group proposed as the normal aging one [49]. Our data give evidences for the first time that increasing tau Thr181 and tau Ser396 immunoreactivity in canine cortical neurons may be associated, apart from aging, with the degree of cognitive dysfunction, but not with the stage of amyloid plaque deposition. Whether tau hyperphosphorylation is a cause of cognitive impairment or an independent phenomenon both related with aging is an open question. Discrete tau hyperphosphorylation in LCD animals, in absence of clinical signs of cognitive dysfunction, may reflect normal aging in dogs [49]. Because the presence of cognitive dysfunction, either light or severe, is also age-dependent, further experiments done with a large number of animals are needed to properly interpret the relationship between aging, cognitive dysfunction, and tau phosphorylation. Phosphorylation of tau at Ser202 observed by other authors in housed beagles [24, 35] has not been detected in the present series. Other studies have shown dog-specific tau deposition, using Tau-1 monoclonal antibodies that recognize the 189 and 207 residues of the human tau sequence [21, 64]. The accumulation of phospho-tau-positive material in the nucleoplasm of neurons observed here is in agreement with the nuclear localization of tau reported in human frontal cortex [6].
Activation of stress kinases c-Jun N-terminal kinase (SAPK/JNK) and p-38 in association with tau phosphorylation has been reported in neurites surrounding Aβ plaques in double mutants for APP (Tg2576) and PS-1 (P264L) mice [58], APP transgenic mice [20, 50] and double APP/tau transgenic mice, which show Aβ plaques, neurofibrillary tangles, and dystrophic neurites in senile plaques [51]. However, no increased p38-P immunoreactivity has been found in the vicinity of amyloid plaques in aged dogs. Thus, the lack of reciprocal interactions between Aβ deposition and tau alterations challenges the idea that tau pathology is merely a downstream effect of Aβ production and deposition. In summary, our results in dogs suggest that Aβ diffuse plaque formation and tau hyperphosphorylation are independent processes, both occurring during the process of aging. Whether tau hyperphosphorylation is part of the process leading to severe cognitive dysfunction would have to be confirmed in future experiments.
References
Anderton BH, Callahan L, Coleman P, Davies P, Flood D, Jicha GA, Ohm T, Weaver C (1998) Dendritic changes in Alzheimer’s disease and factors that may underlie these changes. Prog Neurobiol 55:595–609
Anderton BH, Betts J, Blackstock WP, Brion JP, Chapman S, Connell J, Dayanandan R, Gallo JM, Gibb G, Hanger DP, Hutton M, Kardalinou E, Leroy K, Lovestone S, Mack T, Reynolds CH, Van Slegtenhorst M (2001) Sites of phosphorylation in tau and factors affecting their regulation. Biochem Soc Symp 73–80
Borras D, Ferrer I, Pumarola M (1999) Age-related changes in the brain of the dog. Vet Pathol 36:202–211
Braak H, Braak E (1991) Neuropathological staging of Alzheimer-related changes. Acta Neuropathol 82:235–259
Braak H, Braak E, Strothjohann M (1994) Abnormally phosphorylated tau protein related to the formation of neurofibrillary tangles and neuropil threads in the cerebral cortex of sheep and goat. Neurosci Lett 171:1–4
Brady RM, Zinkowski RP, Binder LI (1995) Presence of tau in isolated nuclei from human brain. Neurobiol Aging 16:479–486
Bugiani O, Giaccone G, Verga L, Pollo B, Ghetti B, Frangione B, Tagliavini F (1990) Alzheimer patients and Down patients: abnormal presynaptic terminals are related to cerebral preamyloid deposits. Neurosci Lett 119:56–59
Colle AA, Hauw JJ, Crespeau F, Uchihara T, Akiyama H, Checler F, Pageat P, Duykaerts C (2000) Vascular and parenchymal Aβ deposition in the aging dog: correlation with behavior. Neurobiol Aging 21:695–704
Cork LC, Powers RE, Selkoe DJ, Davies P, Geyer JJ, Price DL (1988) Neurofibrillary tangles and senile plaques in aged bears. J Neuropathol Exp Neurol 47:629–641
Cummings BJ, Su JH, Cotman CW, White R, Russell MJ (1993) β-Amyloid accumulation in aged canine brain: a model of early plaque formation in Alzheimer’s disease. Neurobiol Aging 14:547–560
Cummings BJ, Head E, Afagh AJ, Milgram NW, Cotman CW (1996) β-Amyloid accumulation correlates with cognitive dysfunction in the aged canine. Neurobiol Learn Mem 66:11–23
Cummings BJ, Pike CJ, Shankle R, Cotman CW (1996) Beta-amyloid deposition and other measures of neuropathology predict cognitive status in Alzheimer’s disease. Neurobiol Aging 17:921–933
Cummings BJ, Satou T, Head E, Milgram NW, Cole GM, Savage MJ, Podlisny MB, Selkoe DJ, Siman R, Greenberg BD, Cotman CW (1996) Diffuse plaques contain C-terminal A beta 42 and not A beta 40: evidence from cats and dogs. Neurobiol Aging 17:653–659
Cummings BJ, Head E, Ruehl W, Milgram NW, Cotman CW (1996) The canine as an animal model of human aging and dementia. Neurobiol Aging 17:259–268
Dickson DW (2001) Neuropathology of Alzheimer’s disease and other dementias. Clin Geriatr Med 17:209–228
Dimakopoulos AC, Mayer RJ (2002) Aspects of neurodegeneration in the canine brain. J Nutr 132:1579S–1582S
Ferrer I, Marti E, Tortosa A, Blasi J (1998) Dystrophic neurites of senile plaques are defective in proteins involved in exocytosis and neurotransmission. J Neuropathol Exp Neurol 57:218–225
Ferrer I, Blanco R, Carmona M, Puig B (2001) Phosphorylated mitogen-activated protein kinase (MAPK/ERK-P), protein kinase of 38 kDa (p38-P), stress-activated protein kinase (SAPK/JNK-P), and calcium/calmodulin-dependent kinase II (CaM kinase II) are differentially expressed in tau deposits in neurons and glial cells in tauopathies. J Neural Transm 108:1397–1415
Ferrer I, Barrachina M, Puig B (2002) Glycogen synthase kinase-3 is associated with neuronal and glial hyperphosphorylated tau deposits in Alzheimer’s disease, Pick’s disease, progressive supranuclear palsy and corticobasal degeneration. Acta Neuropathol (Berl) 104:583–591
Ferrer I, Gomez-Isla T, Puig B, Freixes M, Ribe E, Dalfo E, Avila J (2005) Current advances on different kinases involved in tau phosphorylation, and implications in Alzheimer’s disease and tauopathies. Curr Alzheimer Res 2:3–18
Goedert M, Spillantini MG, Jakes R, Rutherford D, Crowther RA (1989) Multiple isoforms of human microtubule-associated protein tau: sequences and localization in neurofibrillary tangles of Alzheimer’s disease. Neuron 3:519–526
Gyure KA, Durham R, Stewart WF, Smialek JE, Troncoso JC (2001) Intraneuronal abeta-amyloid precedes development of amyloid plaques in Down syndrome. Arch Pathol Lab Med 125:489–492
Head E, Thornton PL, Tong L, Cotman CW (2000) Initiation and propagation of molecular cascades in human brain aging: insight from the canine model to promote successful aging. Prog Neuropsychopharmacol Biol Psychiatr 24:777–786
Head E, Moffat K, Das P, Sarsoza F, Poon WW, Landsberg G, Cotman CW, Murphy MP (2005) Beta-amyloid deposition and tau phosphorylation in clinically characterized aged cats. Neurobiol Aging 26:749–763
Hensley K, Floyd RA, Zheng NY, Nael R, Robinson KA, Nguyen X, Pye QN, Stewart CA, Geddes J, Markesbery WR, Patel E, Johnson GV, Bing G (1999) p38 kinase is activated in the Alzheimer’s disease brain. J Neurochem 72:2053–2058
Higgins LS, Holtzman DM, Rabin J, Mobley WC, Cordell B (1994) Transgenic mouse brain histopathology resembles early Alzheimer’s disease. Ann Neurol 35:598–607
Hirayama A, Horikoshi Y, Maeda M, Ito M, Takashima S (2003) Characteristic developmental expression of amyloid beta 40, 42 and 43 in patients with Down syndrome. Brain Dev 25:180–185
Hof P, Bogaert Y, Rosenthal R, Fiskum G (1996) Distribution of neuronal populations containing neurofilament protein calcium-binding proteins in the canine neocortex: regional analysis and cell typology. J Chem Neuroanat 11:81–98
Hou Y, White RG, Bobik M, Marks JS, Russell MJ (1997) Distribution of beta-amyloid in the canine brain. NeuroReport 8:1009–1012
Johnstone E, Chaney M, Norris F, Pascual R, Little S (1991) Conservation of the sequence of the Alzheimer’s disease amyloid peptide in dog, polar bear, and five other mammals by cross-species polymerase chain reaction analysis. Brain Res 10:299–305
Knopman DS, Parisi JE, Salviati A, Floriach-Robert M, Boeve BF, Ivnik RJ, Smith GE, Dickson DW, Johnson KA, Petersen LE, McDonald WC, Braak H, Petersen RC (2003) Neuropathology of cognitively normal elderly. J Neuropathol Exp Neurol 62:1087–1095
LaFerla FM, Oddo S (2005) Alzheimer’s disease: Abeta, tau and synaptic dysfunction. Trends Mol Med 11:170–176
Mackenzie IR, Hao C, Munoz DG (1995) Role of microglia in senile plaque formation. Neurobiol Aging 16:797–804
Mahy N (1993) Brain banks and research in neurochemistry. J Neural Transm 1:119–126
Mailliot C, Podevin-Dimster V, Rosenthal RE, Sergeant N, Delacourte A, Fiskum G, Buee L (2000) Rapid tau protein dephosphorylation and differential rephosphorylation during cardiac arrest-induced cerebral ischemia and reperfusion. J Cereb Blood Flow Metab 20:543–549
Milgram NW, Head E, Zicker SC, Ikeda-Douglas CJ, Murphey H, Muggenburg B, Siwak C, Tapp D, Cotman CW (2005) Learning ability in aged beagle dogs is preserved by behavioral enrichment and dietary fortification: a two-year longitudinal study. Neurobiol Aging 26:77–90
Mitchell TW, Mufson EJ, Schneider JA, Cochran EJ, Nissanov J, Han LY, Bienias JL, Lee VM, Trojanowski JQ, Bennett DA, Arnold SE (2002) Parahippocampal tau pathology in healthy aging, mild cognitive impairment, and early Alzheimer’s disease. Ann Neurol 51:182–189
Morris JC, Storandt M, McKeel DW Jr, Rubin EH, Price JL, Grant EA, Berg L (1996) Cerebral amyloid deposition and diffuse plaques in “normal” aging: evidence for presymptomatic and very mild Alzheimer’s disease. Neurology 46:707–719
Mrak RE, Sheng JG, Griffin WS (1996) Correlation of astrocytic S100 beta expression with dystrophic neurites in amyloid plaques of Alzheimer’s disease. J Neuropathol Exp Neurol 55:273–279
Nagy Z, Esiri MM, Jobst KA, Morris JH, King EM, McDonald B, Litchfield S, Smith A, Barnetson L, Smith AD (1995) Relative roles of plaques and tangles in the dementia of Alzheimer’s disease: correlations using three sets of neuropathological criteria. Dementia 6:21–31
Nagy Z, Esiri MM, Jobst KA, Morris JH, King EM, McDonald B, Joachim C, Litchfield S, Barnetson L, Smith AD (1997) The effects of additional pathology on the cognitive deficit in Alzheimer disease. J Neuropathol Exp Neurol 56:165–170
Olsson A, Csajbok L, Ost M, Hoglund K, Nylen K, Rosengren L, Nellgard B, Blennow K (2004) Marked increase of beta-amyloid(1–42) and amyloid precursor protein in ventricular cerebrospinal fluid after severe traumatic brain injury. J Neurol 251:870–876
Otth C, Concha II, Arendt T, Stieler J, Schliebs R, Gonzalez-Billault C, Maccioni RB (2002) AbetaPP induces cdk5-dependent tau hyperphosphorylation in transgenic mice Tg2576. J Alzheimers Dis 4:417–430
Papaioannou N, van Ederen AM, Tsangaris T, Bohl JRE, Gruys E (2001) Immunohistochemical investigation of the brain of aged dogs. I. Detection of neurofibrillary tangles and of 4-hydroxynonenal protein, an oxidative damage product, in senile plaque. Amyloid: J Protein Fold Disord 8:11–21
Pei JJ, Braak E, Braak H, Grundke-Iqbal I, Iqbal K, Winblad B, Cowburn RF (1999) Distribution of active glycogen synthase kinase 3beta (GSK-3beta) in brains staged for Alzheimer disease neurofibrillary changes. J Neuropathol Exp Neurol 58:1010–1019
Perry G, Roder H, Nunomura A, Takeda A, Friedlich AL, Zhu X, Raina AK, Holbrook N, Siedlak SL, Harris PL, Smith MA (1999) Activation of neuronal extracellular receptor kinase (ERK) in Alzheimer disease links oxidative stress to abnormal phosphorylation. NeuroReport 10:2411–2415
Pierce JE, Trojanowski JQ, Graham DI, Smith DH, McIntosh TK (1996) Immunohistochemical characterization of alterations in the distribution of amyloid precursor proteins and beta-amyloid peptide after experimental brain injury in the rat. J Neurosci 16:1083–1090
Pugliese M, Carrasco JL, Geloso MC, Mascort J, Michetti F, Mahy N (2004) g-Aminobutyric acidergic interneuron vulnerability to aging in canine frontal cortex. J Neurosci Res 77:913–920
Pugliese M, Carrasco JL, Andrade C, Mas E, Mascort J, Mahy N (2005) Severe cognitive impairment correlates with higher cerebrospinal fluid levels of lactate and pyruvate in a canine model of senile dementia. Prog Neuropsychopharmacol Biol Psychiatr 29:603–610
Puig B, Gomez-Isla T, Ribe E, Cuadrado M, Torrejon-Escribano B, Dalfo E, Ferrer I (2004) Expression of stress-activated kinases c-Jun N-terminal kinase (SAPK/JNK-P) and p38 kinase (p38-P), and tau hyperphosphorylation in neurites surrounding betaA plaques in APP Tg2576 mice. Neuropathol Appl Neurobiol 30:491–502
Ribe EM, Perez M, Puig B, Gich I, Lim F, Cuadrado M, Sesma T, Catena S, Sanchez B, Nieto M, Gomez-Ramos P, Moran MA, Cabodevilla F, Samaranch L, Ortiz L, Perez A, Ferrer I, Avila J, Gomez-Isla T (2005) Accelerated amyloid deposition, neurofibrillary degeneration and neuronal loss in double mutant APP/tau transgenic mice. Neurobiol Dis 20:814–822
Riederer BM, Mourton-Gilles C, Frey P, Delacourte A, Probst A (2001) Differential phosphorylation of tau proteins during kitten brain development and Alzheimer’s disease. J Neurocytol 30:145–158
Roberts GW, Allsop D, Bruton C (1990) The occult aftermath of boxing. J Neurol Neurosurg Psychiatry 53:373–378
Rofina J, van Andel I, van Ederen AM, Papaioannou N, Yamaguchi H, Gruys E (2003) Canine counterpart of senile dementia of the Alzheimer type: amyloid plaques near capillaries but lack of spatial relationship with activated microglia and macrophages. Amyloid 10:86–96
Roudebush P, Zicker SC, Cotman CW, Milgram NW, Muggenburg BA, Head E (2005) Nutritional management of brain aging in dogs. J Am Vet Med Assoc 227:722–728
Russell MJ, White R, Patel E, Markesbery WR, Watson CR, Geddes JW (1992) Familial influence on plaque formation in the beagle brain. NeuroReport 3:1093–1096
Satou T, Cummings BJ, Head E, Nielson KA, Hahn FF, Milgram NW, Velazquez P, Cribbs DH, Tenner AJ, Cotman CW (1997) The progression of β-amyloid deposition in the frontal cortex of the aged canine. Brain Res 774:35–43
Savage MJ, Lin YG, Ciallella JR, Flood DG, Scott RW (2002) Activation of c-Jun N-terminal kinase and p38 in an Alzheimer’s disease model is associated with amyloid deposition. J Neurosci 22:3376–3385
Schultz C, Hubbard GB, Rub U, Braak E, Braak H (2000) Age-related progression of tau pathology in brains of baboons. Neurobiol Aging 21:905–912
Smith MA, Rottkamp CA, Nunomura A, Raina AK, Perry G (2000) Oxidative stress in Alzheimer’s disease. Biochim Biophys Acta 1502:139–144
Stoothoff WH, Johnson GV (2005) Tau phosphorylation: physiological and pathological consequences. Biochim Biophys Acta 1739:280–297
Tomidokoro Y, Harigaya Y, Matsubara E, Ikeda M, Kawarabayashi T, Shirao T, Ishiguro K, Okamoto K, Younkin SG, Shoji M (2001) Brain Abeta amyloidosis in APPsw mice induces accumulation of presenilin-1 and tau. J Pathol 194:500–506
Vehmas AK, Kawas CH, Stewart WF, Troncoso JC (2003) Immune reactive cells in senile plaques and cognitive decline in Alzheimer’s disease. Neurobiol Aging 24:321–331
Wegiel J, Wisniewski HM, Soltysiak Z (1998) Region- and cell-type-specific pattern of tau phosphorylation in dog brain. Brain Res 802:259–266
Wenk GL (2003) Neuropathologic changes in Alzheimer’s disease. J Clin Psychiatry 64(Suppl 9):7–10
Wisniewski T, Lalowski M, Golabek A, Vogel T, Frangione B (1995) Is Alzheimer’s disease an apolipoprotein E amyloidosis? Lancet 345:956–958
Wisniewski T, Lalowski M, Bobik M, Russell M, Strosznajder J, Frangione B (1996) Amyloid b 1–42 deposits do not lead to Alzheimer’s neuritic plaques in aged dogs. Biochem J 313:575–580
Yamamoto T, Hirano A (1986) A comparative study of modified Bielschowsky, Bodian and thioflavin S stains on Alzheimer’s neurofibrillary tangles. Neuropathol Appl Neurobiol 12:3–9
Acknowledgements
The research projects V-2003REDCIEN IDIBAPS-ISCIIIRTIC C03/06 and V-2003-REDG167B-0 of the Spanish Ministerio de Sanidad and SAF 2005-04314 of the Spanish Ministerio de Educación y Ciencia provided support for this study. We thank T. Yohannan for editorial advice.
Author information
Authors and Affiliations
Corresponding author
Rights and permissions
About this article
Cite this article
Pugliese, M., Mascort, J., Mahy, N. et al. Diffuse beta-amyloid plaques and hyperphosphorylated tau are unrelated processes in aged dogs with behavioral deficits. Acta Neuropathol 112, 175–183 (2006). https://doi.org/10.1007/s00401-006-0087-3
Received:
Revised:
Accepted:
Published:
Issue Date:
DOI: https://doi.org/10.1007/s00401-006-0087-3