Abstract
The brassinosteroids (BRs) constitute a recently defined class of plant hormone that can enhance the resistance of plants to multiple stresses. However, the effects of BRs on salt-stressed woody plants, notably on photosynthesis and chloroplast ultrastructure, have received little attention. Black locust (Robinia pseudoacacia L.) seeds and seedlings were pretreated with 1.04 µmol L− 1 24-epibrassinolide (24-epiBL) by soaking and root dipping, respectively, and grown under non-saline or saline conditions (0, 100, 200 mmol L− 1 NaCl). Salinity stress decreased photosynthesis, chlorophyll concentration, transpiration, and stomatal conductance but also decreased the water-use efficiency, while chlorophyll fluorescence indicated a decrease in photochemical quenching and in maximum potential quantum efficiency. Indicators of oxidative stress (for example, H2O2 and antioxidant enzymes), membrane leakage, and amounts of Na+ ions in leaves and chloroplasts were increased and, at the highest stress, chloroplast ultrastructure was severely disrupted. Exogenous 24-epiBL improved membrane stability and reduced foliar Na+ levels, while substantially alleviating stress-induced changes in photosynthetic gas exchange. Improvements in chlorophyll content and indicators of oxidative stress were not as large but were still highly significant. Thylakoid membrane structure was protected. Both methods of applying 24-epiBL were effective, but root-dipped seedlings performed marginally better. The results suggest that treatment of black locust seedlings with 24-epiBL prior to planting may improve performance and aid establishment on salt-affected soils.
Similar content being viewed by others
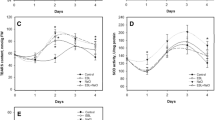
Explore related subjects
Discover the latest articles, news and stories from top researchers in related subjects.Avoid common mistakes on your manuscript.
Introduction
Abiotic stresses such as soil salinity, drought, flooding, or extreme temperatures reduce crop productivity and impact global food security (Thakur et al. 2010). Worldwide, over 1 billion hectares of land have been damaged by salt (Shahid 2013) causing huge losses in agricultural production (Bartels and Sunkar 2005; Wang et al. 2003). Vegetative bioremediation is a crucially important reclamation approach on saline–alkali soils (Qadir and Oster 2004); for example, trees can be used in agroforestry applications to reduce dryland salinity and related soil degradation problems (Ellis and van Dijk 2009).
China, the world’s most populous country (1.38 billion people in 2015) has the third largest area of saline soils (366,500 km2), accounting for 4.88% of the country’s arable land (Li et al. 2014). Black locust (Robinia pseudoacacia L.), an arboreal member of the Fabaceae indigenous to the eastern United States, has been widely cultivated in China since the early twentieth century (Tateno et al. 2007; Akatov et al. 2016) where it is now the third most planted tree after hybrid poplar and eucalyptus (DeGomez and Wagner 2001). The species has also been introduced to Africa, Europe, Australia, New Zealand, and other parts of Asia and North America. The popularity of black locust is connected with rapid growth, symbiotic nitrogen-fixation, adaptability to a wide range of environmental conditions, abundant seed crops, and high timber and honey yields. It is a major tree species for planting into degraded areas such as exposed sand (Rahmonov 2009), mine wastes (DeGomez and Wagner 2001), and saline–alkali soils (Wang et al. 2013) for purposes of soil amelioration, erosion control, and reforestation (Sitzia et al. 2012). Studies have shown, however, that black locust is only weakly salt-tolerant, withstanding salinity contents of up to only 0.3% under laboratory conditions, or to just over 0.5% under field conditions (Liu et al. 2010). As of 2001 (CSDI of Ministry of Construction, China), saline–alkali soils in China have been classified into four degree levels according to the content of NaCl per 100 g dry weight of soil: weak (0.3–1%), moderate (1–5%), heavy (5–8%), and super heavy (> 8%). Therefore, the salt tolerance of black locust needs improvement if it is to be successful on even weakly affected soils.
Plant hormones have long been implicated in regulating plant development and tolerance or susceptibility to diverse stresses, including salinity stress. Brassinosteroids (BRs) are a group of plant steroid hormones originally indicated by the work of Mitchell et al. (1970), and brassinolide was the first BR to be isolated and identified (Grove et al. 1979). Brassinosteroids are perceived by a family of plasma membrane-localized leucine-rich-repeat-receptor kinases in interaction with a co-receptor. The ensuing signal cascade releases several transcription factors that regulate the expression of over a 1000 genes involved in a broad range of physiological functions. BRs participate in the regulation of cell division and expansion, germination, vascular differentiation, root growth, vegetative and reproductive development, homeostasis, and programmed cell death (Kemmerling et al. 2007; Divi and Krishna 2009; Park et al. 2010).
Protective effects of BRs against biotic and abiotic stresses have been frequently reported; e.g., in wheat (Talaat and Shawky 2012), rice (Thussagunpanit et al. 2015), zucchini (Tao et al. 2015), tea (Li et al. 2016), radish (Choudhary et al. 2011), Arabidopsis (Park et al. 2010), grape (Wang et al. 2015), and eggplant (Wu et al. 2014). BR-mediated responses to stress occur across different levels of organization and are not fully understood (Sharma et al. 2017). A general observation, however, is that application of BRs lowers oxidative stress. Cui et al. (2016) showed that exogenous application of 0.1 μM 24-epibrassinolide (24-epiBL) promoted antioxidant enzyme systems in tomato. Similarly, a 24-epiBL pretreatment (0.2–0.3 mM) could restrain reactive oxygen species (ROS) and lipid peroxidation of young grapevine seedlings under chilling stress (Xi et al. 2013). Brassinosteroids may reduce oxidative stress in part through interactions with signal transduction pathways for salicylic acid (Sharma et al. 2017), ethylene (Wei et al. 2015), and nitric oxide (Zhu et al. 2015).
The growth and productivity of plants has a close relationship with photosynthesis, which is one of the most severely affected processes during salinity stress (Sudhir and Murthy 2004). Under salt stress, the root application of 24-epiBL was able to enhance the photosynthetic capacity of wheat plants (Ali et al. 2008b). Similarly, foliar application of 24-epiBL enhanced photosynthesis in Brassica juncea (L.) Czern. (Ali et al. 2008a). Hayat et al. (2010) reported that BRs could maintain photosynthesis by protecting the quantum yield of PSII under salt stress. Existing reports of the effects of BRs on salt-resistance mainly deal with herbaceous crops, but there is little data pertaining to woody plants, particularly at the organelle (that is, chloroplast) level.
To devise new strategies to enhance salt-affected soils, we sought to improve the early tolerance of black locust to salinity stress. To that end, we compared growth and photosynthesis in control and 24-epiBL-treated plants exposed to two different salt levels and conducted measurements of oxidative stress, membrane integrity, chlorophyll content, Na+ content, fluorescence parameters, and chloroplast ultrastructure. Our objective was to determine whether exogenous 24-epiBL could improve the performance of black locust seedlings exposed to higher levels of salt than they would normally tolerate, to enhance the availability of alkaline land for food production.
Materials and Methods
Plant Material and 24-epiBL Treatments
The seeds of black locust (Robinia pseudoacacia L.) used in this study were obtained from South Forest Tree and Seed Testing Center of the State Forestry Administration in China and experiments were conducted from September 2014 to July 2015. Seeds were surface sterilized in hot deionized water (85–95 °C) for a few seconds to remove the waxy cuticle. Seeds were then soaked in either distilled water or 1.04 µM 24-epiBL (Sigma-Aldrich, St. Louis, MO, USA) for 24 h before sowing into trays containing quartz sand moistened with either distilled water or 1.04 µM 24-epiBL, respectively. The 24-epiBL was prepared by first dissolving 0.1 mg 24-epiBL in 10 mL absolute ethanol as a stock solution; this was diluted just before use to yield the 1.04 µM treatment solution in 0.1% Teepol. Seeds were germinated at 25 °C under constant light at a photosynthetic photon flux density (PPFD) of 40 µmol m− 2 s− 1. After 10 days, when cotyledons were fully expanded, seedlings were transplanted into seedling bags (10 cm diameter × 15 cm depth) filled with a mixture of vermiculite, peat, and perlite (1:2:2, v/v). Seedlings were cultivated under controlled conditions in a greenhouse at the College of Forestry, Nanjing Forestry University (32°7′N, 119°12′E), Jiangsu Province, China. Supplemental lighting provided a minimum 12-h photoperiod and PPFD of 350 µmol m− 2 s− 1, and the day/night temperature regime was 26 °C/16 °C.
Seedlings grew without stress for 6 months until the spring of 2015, by which time they were ~ 45 cm tall. At this point, all seedlings were washed of rooting medium. Seedlings from seeds pretreated with 24-epiBL were replanted directly into plastic pots (40 cm × 25 cm × 15 cm; 1 plant per pot) containing coarse sand and vermiculite 2:1 (v:v). Seedlings from untreated seeds were split into two groups and root-dipped for 48 h in either distilled water or 1.04 µM 24-epiBL (refreshed every 12 h) prior to being replanted in the same way. Afterwards, seedlings were watered to the drip-point every 7 days with a modified Hoagland’s nutrient solution (Hoagland and Arnon 1950) containing 0.2 mM KH2PO4, 1.0 mM K2SO4, 2.0 mM Ca(NO3)2, 0.5 mM MgSO4, 200 µM Fe-EDTA, 5 µM H3BO3, 2 µM MnSO4, 0.5 µM ZnSO4, 0.3 µM CuSO4, and 0.01 µM (NH4)2Mo7O24, with pH adjusted to 7.0.
Groups and Salt Stress Treatments
After a further 2 weeks, seedlings of uniform height (~ 50 cm) were selected for the salt stress treatments. There were three groups: seedlings never exposed to 24-epiBL (CK); seedlings from seeds treated with 24-epiBL (Sew); and seedlings root-dipped in 24-epiBL (Diw). Fifteen seedlings of each group were subjected to each of three levels of salt stress: 0, 100, and 200 mM NaCl, all in Hoagland’s solution. The overall experiment, therefore, consisted of nine treatment combinations in a 2-way factorial: CK-0, CK-100, CK-200, Sew-0, Sew-100, Sew-200, Diw-0, Diw-100, and Diw-200. Pots were flushed with their respective nutrient solutions and then, thereafter, the water content was kept near saturation (40%) by automatic drip-feeding with fresh solution delivered by an Intelligent Automatic Watering System (patent CN 201398356 Y). Data for gas exchange, chlorophyll fluorescence, antioxidant systems, and chloroplast ultrastructure were collected as described below, beginning 45 days after treatments commenced. Over the measurement period, the day/night temperature regime was 29 °C/18 °C, relative humidity varied from 45 to 80%, and natural lighting provided a photoperiod of about 14 h and a mid-day PPFD of ~ 1000 µmol m− 2 s− 1.
Determination of Gas Exchange Variables
Leaf gas exchange was measured using an infrared gas exchange analyzer (LI-6400, LI-COR Inc., Lincoln, NE, USA) from 09:30 to 11:30 h local time, on clear days in May. The net photosynthetic rate (An), stomatal conductance (gs), transpiration rate (E), and intercellular CO2 concentration (Ci) were measured on 0.7 cm × 3.0 cm (2.1 cm2) of leaf area using an ambient CO2 concentration (Ca) of 380 µmol mol− 1. The relative air humidity and leaf temperature in the chamber were maintained at ~ 35% and 25 °C, respectively, while PPFD ranged from 1200 to 1400 μmol m− 2 s− 1. For each treatment, four fully expanded leaves from each of three seedlings were assayed. The stomatal limitation (Ls) and the water-use efficiency (WUE) were calculated as Ls=1−Ci/Ca and WUE = An/E, respectively, according to Li et al. (2013).
Chlorophyll Fluorescence
Chlorophyll fluorescence was measured using a portable fluorometer (PAM-2500; Walz, Effeltrich, Germany) as described by Roháček (2002). The data were taken on the fourth fully expanded leaf of each seedling, numbered basipetally. The minimal (Fo) and maximal (Fm) chlorophyll fluorescence emission was determined after 30 min of dark adaptation, and minimal (\(F_{{\text{o}}}^{\prime }\)) and maximal (\(F_{\rm m}^{\prime }\)) fluorescence level in the light-adapted state, and the steady-state value of fluorescence (Fs), were measured after light adaption at 600 μmol m− 2 s− 1. We report the maximum quantum yield of PSII as Fv/Fm, the size and number of open reaction centers as Fv/Fo, photochemical quenching (Qp) as \((F_{{\text{m}}}^{\prime } - {F_{\text{s}}})/(F_{{\text{m}}}^{\prime } - F_{{\text{o}}}^{\prime }),\) and non-photochemical quenching (NPQ) as \(({F_{\text{m}}} - F_{{\text{m}}}^{\prime })/F_{{\text{m}}}^{\prime }.\)
Estimation of Membrane Stability Index
The membrane stability index (MSI) was estimated by taking 200 mg leaf material in 10 mL of double-distilled water (DDW) in two sets. One set was heated at 40 °C for 30 min in a water bath and the electrical conductivity (C1) was measured on a conductivity meter. The second set was boiled at 100 °C in a boiling water bath for 10 min and its conductivity was also measured (C2). MSI was calculated using the formula given by Sairam (1994):
Determination of Relative Water Content
Relative water content (RWC) was determined on fresh leaf discs of 2 cm diameter. Discs were weighed quickly (FW) and immediately floated on DDW for 24 h in the dark. Discs were blotted dry and reweighed to obtain mass at full turgor (TW), then dried at 70 °C for 48 h to obtain the dry mass (DW). RWC was calculated according to Hayat et al. (2007):
Element Analysis by Inductively Coupled Plasma Optical Emission Spectrometry (ICP-OES)
At the final harvest time (day 50), leaves (numbers 6–8, counting from the base of the shoot) were sampled from control and salt-stressed plants. Following the procedures of Hajibagheri and Flowers (1984), 30–50 mg of oven-dried leaf tissue was added to 2 mL 65% HNO3 and pressure-extracted for 12 h at 140 °C on a SpeedDigester K-436 (BUCHI, Switzerland) until the liquid was almost clear. It was then filtered, diluted as necessary, and used for elemental analysis on a ICP-OES spectrometer (Spectro Ciros CCD, GmbH & Co KG, Kleve, Germany) at wavelengths of 226–502 nm (Abo-Ogiala et al. 2014).
Observation of Chloroplast Ultrastructure
The ultrastructure of the chloroplasts was observed as described by Shu et al. (2013). Leaf segments were cut into pieces of approximately 1 mm2. The leaf pieces were immersed in a mixture of 3% glutaraldehyde and 1% formaldehyde in a 0.1 M phosphate buffer (pH 7.4) for 2 h (primary fixation) and then 2% osmic acid in the same buffer for 2 h (secondary fixation). After dehydration in acetone and embedding in Durcupan ACM (Fluka), ultrathin sections were cut, stained with uranium acetate and lead citrate in series, and examined under a Hitachi transmission electron microscope (Carl Zeiss, Göttingen, Germany) using an 80-kV acceleration voltage.
Isolation of Chloroplasts
Chloroplasts were isolated from fully expanded leaves by differential and density gradient centrifugation as described by Cerović and Plesnicar (1984) and Song et al. (2006) with some modifications. Briefly, 5 g of leaflets were chopped using a blender (HR-2826; Philips, Zhuhai, China) with five volumes of a medium containing 0.4 M sucrose, 2 mM EDTA, 5 mM MgCl2·6H2O, and 0.1% BSA in 50 mM Tris–HCl buffer (pH 7.6). The homogenate was squeezed through six layers of gauze and the filtrate was centrifuged at 2000×g for 2 min. The resulting pellet was resuspended in 2 mL of medium containing 0.3 M sorbitol, 2 mM EDTA, and 1 mM MgCl2·6H2O in 50 mM HEPES-KOH buffer (pH 7.8), which was put into a tube containing 8 mL of resuspension medium plus 40–80% (v/v) Percoll and centrifuged for 3 min at 2000×g. The interlayer (7 mL) between 40% and 80% Percoll contained intact, isolated chloroplasts. All procedures were carried out at 4 °C.
A 2 mL aliquot of the interlayer was brought up to 10 mL with 0.05 M sodium phosphate buffer (pH 7.8) containing 5 mM EDTA, for analysis of antioxidant enzymes, H2O2, and malondialdehyde (MDA). For measurement of chlorophyll a (Chl a), chlorophyll b (Chl b) and total chlorophyll, another 2 mL aliquot was spun down (1000×g for 10 min at 4 °C) and the pelleted chloroplasts were resuspended in 8 mL of 80% acetone and assayed spectrophotometrically according to Arnon (1949) by reading the absorbance at 645 and 663 nm. For chloroplast Na+ content, pelleted chloroplasts from a final 1 mL aliquot were extracted with 5 mL of 0.5 M HCl by shaking on a water bath at 50 °C for 45 min. The volume was brought up to 10 mL with 0.5 M HCl before filtering and diluting for ICP-OES as described above.
Assays for H2O2, MDA, and Antioxidant Enzyme Activities
The H2O2 assay was based on the formation and spectrophotometric measurement of a titanium peroxide complex according to Patterson et al. (1984) as modified by Diao et al. (2014). MDA was measured in terms of thiobarbituric acid reactive substances (TBARS) following Heath and Packer (1968). Superoxide dismutase (SOD) was assayed by monitoring its ability to inhibit the photochemical reduction of nitro blue tetrazolium (NBT) at 560 nm (Beyer and Fridovich 1987). One unit of SOD activity is the amount required to reduce the rate of reduction by 50% at 560 nm. Ascorbate peroxidase (APX) activity was measured according to Nakano and Asada (1981) by following the decrease in absorbance at 290 nm as ascorbate was oxidized by added H2O2. One unit of APX is the amount of enzyme required to oxidize ascorbate at a rate of 1 mM min− 1.
Statistical Analysis
All biochemical analyses had at least three biological replicates. Data were statistically analyzed with R 3.3.0 (SPSS Inc., Chicago, IL, USA) using a two-factorial completely randomized design. Two-way analyses of variance (ANOVA) were employed to test the effects of salt stress, 24-epiBL and their interaction, and means were separated using Duncan’s multiple range tests. Where necessary (APX, MDA, MSI, and Na+ concentrations in leaves and chloroplasts), data were square root and/or log transformed to meet assumptions of normality and homogeneity of variance. Standard error bars in figures are based on untransformed data. Differences were considered significant at P < 0.05.
Results
Gas Exchange
In the absence of salt stress, neither of the 24-epiBL treatments had a significant effect on photosynthesis (An), transpiration (E), stomatal conductance (gs), water-use efficiency (WUE), or the degree of stomatal limitation (Ls), but the Diw treatment did result in a lower intercellular CO2 concentration (Ci). Salt stress, in the absence of any 24-epiBL treatment, reduced An to about a quarter the control rate (Fig. 1a). This dramatic inhibition of photosynthesis was partially prevented by treatment with 24-epiBL, particularly with Diw. Indeed, 24-epiBL-treated plants had photosynthetic rates that were 3.0–3.8 times higher than CK-100 or CK-200 plants. Transpiration rates showed very similar though less dramatic differences. Concurrent with the effects of salt stress on An and E was a general reduction in gs. Stomatal conductance was unaffected by 24-epiBL treatment at 100 mM NaCl but both Sew and Diw prevented any further decrease in gs at 200 mM NaCl. Overall, the net effect of the changes in An and E under salt stress was a large reduction in WUE (Fig. 1e), but this effect was entirely reversible by pretreatment with 24-epiBL. These changes in WUE occurred in the absence of any consistent change in Ci or Ls; raising the possibility that the effects of salt stress, and the protective effects of 24-epiBL, were at the level of the mesophyll.
Changes in a net photosynthetic rate (An), b intercellular CO2 concentration (Ci), c transpiration rate (E), d stomatal conductance (gs), e water-use efficiency (WUE), and f stomatal limitation (Ls) in leaves of black locust seedlings at three levels of salt stress, with or without prior treatment with 24-epiBL (CK: no 24-epiBL; Sew: seeds soaked in 1.04 µM 24-epiBL; Diw: roots dipped in 1.04 µM 24-epiBL). Each bar represents the mean (±SE) of three replicates. Dc, Dw, and Dc*Dw are the P values for the salt concentration effect, the 24-epiBL application effect, and the interaction effect between salt concentration and 24-epiBL application, respectively. Different letters above bars show significant differences between means (P < 0.05)
Chlorophyll Fluorescence
The 24-epiBL pretreatments had no effect on the maximum quantum yield of PSII (Fv/Fm) or the potential photochemical efficiency of PSII (Fv/Fo) in unstressed black locust seedlings, but did increase both the photochemical (Qp) and non-photochemical (NPQ) quenching of fluorescence in these seedlings (Fig. 2), suggesting an increase in open PSII reaction centers and enhanced heat dissipation of excitation energy. Salt stress led to a small decrease in Fv/Fm (P = 0.002), which was prevented by 24-epiBL pretreatment of roots (Diw) but not seeds (Sew). There were large decreases in Fv/Fo at 100 mM NaCl (P < 0.001) but no further decline in this variable at 200 mM NaCl (Fig. 2b). As in the zero salt controls, the 24-epiBL pretreatments had no effect on Fv/Fo. Non-photochemical quenching was about 1.2–1.3 times higher at 100 mM NaCl, and perhaps slightly more at 200 mM NaCl (Fig. 2c). Although 24-epiBL increased NPQ under control conditions, it had no further effect under salt stress (P = 0.011 for the interaction effect). Photochemical quenching was substantially reduced by salt stress (P < 0.001) and more so at 200 than at 100 mM NaCl (Fig. 2d). Pretreatment of seeds with 24-epiBL enhanced Qp by 31% at 100 mM NaCl but was not significantly effective at 200 mM; in contrast, unlike under control conditions, the root-dipping pretreatment was without effect under either level of salinity stress.
Effects of exogenous 24-epiBL on chlorophyll fluorescence parameters in leaves of black locust seedlings at three levels of salt stress, with or without prior treatment with 24-epiBL (CK: no 24-epiBL; Sew: seeds soaked in 1.04 µM 24-epiBL; Diw: roots dipped in 1.04 µM 24-epiBL). Other details as in Fig. 1
Chloroplast Ultrastructure and Chlorophyll Levels
Alterations in the structure of chloroplasts and thylakoids induced by salt stress are illustrated in Fig. 3. Relative to controls (Fig. 3a, b), the chloroplasts of salt-stressed plants were swollen and partly separated from the plasma membrane (Fig. 3c, e). The shape of chloroplasts changed from elliptical to nearly round, and the lamellar structure of thylakoids was less regular, particularly at 200 mM NaCl (Fig. 3e, f). Plastoglobuli were swollen but fewer in number compared to the control. Under the higher salt stress (200 mM NaCl), the cell wall was bent and starch granules were smaller and less well defined (Fig. 3e, f). These severe impacts on chloroplast structure were partly alleviated by the 24-epiBL pretreatments. Application of 24-epiBL increased the number of plastoglobuli and maintained the fabric of thylakoid lamellae relative to stressed controls (Fig. 3g–n). At 100 mM NaCl, both 24-epiBL pretreatments decreased the swelling of chloroplasts (compare panels g and i with c) and preserved the structure of thylakoid lamellae (compare panels h and j with d). Swelling of chloroplasts at 200 mM NaCl was not avoided by the 24-epiBL pretreatments, but lamellae appeared relatively normal, particularly with the Diw pretreatment (Fig. 3k–n).
The effects of salt stress on the ultrastructure of chloroplasts and thylakoid membranes in leaves of black locust seedlings at three levels of salt stress, with or without prior treatment with 24-epiBL. a, b CK-0; c, d CK-100; e, f CK-200; g, h Sew-100; i, j Diw-100; k, l Sew-200; m, n Diw-200. The second fully expanded leaves, numbered basipetally, were sampled for ultramicroscopic observation after 50 days of salt stress. CW cell wall, PM plasma membrane, ChM chloroplast membrane, SG starch granule, OS osmiophilic plastoglobuli, Thl thylakoid, G grana, Thl thylakoid lamella. Scale bars for chloroplasts and thylakoids are 5 µm and 1 µm, respectively
Pretreatment with 24-epiBL had a highly significant positive effect on Chl a, Chl b, and total chlorophyll concentrations of chloroplasts (Table 1). Concentrations reached their highest levels in Diw, but these were only significantly greater than Sew in the case of Chl a. Salt-stressed plants had substantially lower chlorophyll concentrations than the unstressed controls. The 24-epiBL pretreatments partially ameliorated this decline, but not statistically so in all cases (the interaction effect was significant for Chl a and total chlorophyll, but not for Chl b). The root-dipping pretreatment was the most effective, increasing total chlorophyll by 81% at 200 mM NaCl (Table 1).
Tissue and Chloroplast Na+ Concentrations
Sodium concentrations in leaves (per unit fresh mass) and chloroplasts (per unit protein) followed very similar trends (Fig. 4). The 100 and 200 mM NaCl treatments increased foliar Na+ by 2.8-fold and 11.7-fold, and chloroplast Na+ by 5.5-fold and 16.8-fold, respectively. The 24-epiBL pretreatments reduced Na+ levels in leaves of controls and salt-stressed plants, with root dipping being most effective in the latter (Fig. 4a). In contrast, the 24-epiBL pretreatments did not result in lower Na+ in chloroplasts at 200 mM NaCl, but did result in a 24 ~ 28% reduction at 100 mM NaCl (interaction effect significant at P = 0.003).
Changes in Na+ concentrations in a leaves and b chloroplasts of black locust seedlings at three levels of salt stress, with or without prior treatment with 24-epiBL (CK: no 24-epiBL; Sew: seeds soaked in 1.04 µM 24-epiBL; Diw: roots dipped in 1.04 µM 24-epiBL). Other details as in Fig. 1
Membrane Stability Index and Relative Water Content
The effects of salt stress, pretreatment with 24-epiBL, and their interaction on membrane stability were all highly significant (Fig. 5a). The 24-epiBL pretreatments had no effect on the MSI in the absence of stress. However, under the NaCl treatments, both Sew and Diw were dramatically effective in maintaining the MSI near the control CK-0 level. In sharp contrast, the MSI fell to 67.8% and 46.1% of the control level at 100 and 200 mM NaCl, respectively, in seedlings that received no 24-epiBL pretreatment. Changes in relative water content (RWC) were similar in direction to changes in MSI, but not as pronounced (Fig. 5b). Only the Diw pretreatment was significantly different from the seedlings that were not treated with 24-epiBL, and only under salt stress.
Changes in a membrane stability index (MSI) and b relative water content (RWC) of leaves of black locust seedlings at three levels of salt stress, with or without prior treatment with 24-epiBL (CK: no 24-epiBL; Sew: seeds soaked in 1.04 µM 24-epiBL; Diw: roots dipped in 1.04 µM 24-epiBL). Each bar represents the mean (±SE) of three replicates. Other details as in Fig. 1
H2O2, MDA, and Antioxidant Systems
Chloroplasts of salt-stressed plants (CK-100, CK-200) had increased levels of H2O2 (Table 2) and, especially, MDA when compared to the unstressed control (CK-0). Pretreatment with 24-epiBL had no effect in the absence of salt stress but substantially reduced accumulations of H2O2 and MDA in the presence of stress. Again, Diw was generally more effective than Sew, but not statistically so in every case (interaction effect significant at P < 0.0001). Root dipping decreased the amounts of H2O2 and MDA by 68% and 92% at 100 mM NaCl, and by 59% and 74% at 200 mM NaCl.
Activities of APX and SOD were increased by 2.37 and 2.65 times, respectively, in chloroplasts under 100 mM NaCl stress, but under 200 mM NaCl the APX activity decreased dramatically (by 85% relative to CK-0) and SOD activity was also reduced but still exceeded the unstressed controls by 1.58 times (Table 2). Treatment of no salt controls with 24-epiBL increased the APX activity by 73–79% but had no effect on SOD activity in CK-0 plants. Both 24-epiBL pretreatments increased the chloroplast APX activity by 2.4–3.2 times in stressed plants, but only Diw stimulated the SOD activity and only at 200 mM NaCl (by 34%).
Discussion
Numerous workers have reported the benefits of 24-epiBL applications on plants and the efficacy of those treatments in alleviating the symptoms of abiotic stress, including high- and low-temperature stress, freezing, drought, heavy metals, and salt stress. The dose and method of application of 24-epiBL varies from study to study and species to species. For example, protective effects of exogenous 24-epiBL have been reported for heat stress in rice (Thussagunpanit et al. 2015), melon (Zhang et al. 2013), and eggplant (Wu et al. 2014), chilling in cucumber (Jiang et al. 2013), zinc-induced oxidative stress in radish (Ramakrishna and Rao 2012), and salt stress in wheat (Talaat and Shawky 2012). There are few reports, however, that involve woody plants. In experiments with grapevine, Xi et al. (2013) found that exogenous 24-epiBL enhanced the activity of antioxidants systems during chilling stress, whereas Wang et al. (2015) reported that 24-epiBL alleviated stomatal and non-stomatal limitations on photosynthesis and associated effects on chlorophyll fluorescence and chloroplast ultrastructure during water stress. Below, we evaluate the impact of salt stress on photosynthesis and related variables in black locust, and follow this with a discussion of how these impacts were ameliorated by exogenous 24-epiBL.
Impacts of Salinity Stress on Black Locust Seedlings
As shown in Fig. 1, even the 100 mM NaCl treatment caused a severe decline in An in black locust seedlings. Stomatal conductance and transpiration were also reduced, suggesting a diffusion limitation on An associated with osmotic stress. The possibility that there was some osmotic stress is supported by a reduction in RWC, albeit a non-significant one (Fig. 5b). The Ci, however, was relatively constant, consistent with a concurrent biochemical limitation on An (Flexas and Medrano 2002). Consequently, although the calculated stomatal limitation (Ls) in salt-stressed plants was higher than in controls, the difference was not significant (Fig. 1f). Indeed, salinity had less severe impacts on gs and E than on An, resulting in a large drop in WUE. The drop in WUE independent from any significant change in Ci or Ls suggests a negative effect of salt stress on the mesophyll conductance (gm). We did not explore this possibility, but the change in shape of the chloroplasts to a more rounded form, less appressed to the cell wall (Fig. 3c, e), would lengthen the aqueous phase of the CO2 diffusion pathway (Milla-Moreno et al. 2016). Xu et al. (2013) found that gm was much more seriously affected than gs in poplar (Populus cathayana Rehder) under alkaline (Na2CO3) stress. Swelling of chloroplasts and their positioning towards a more central location less closely associated with the cell wall are common phenomena in salt-stressed crop plants; for example, rice (Yamane et al. 2008) and potato (Gao et al. 2014).
Chlorophyll fluorescence monitoring has been widely used to assay the health of the photosynthetic mechanism under environmental stress (Sayed 2003). The incremental decrease in Fv/Fm we observed in salt-stressed plants indicates a progressive reduction in the maximum quantum yield of photosynthesis (Fig. 2), whereas the sharp decrease in Fv/Fo suggests a more immediate impact on the water-splitting complex (Kalaji et al. 2011). Photochemical quenching (Qp) decreased while NPQ increased, consistent with reduced rates of carbon fixation and greater energy dissipation as heat (Oxborough 2004). Our findings are in agreement with the salt stress studies of Wu et al. (2012) on eggplant, Wang et al. (2007) on poplar, and Shu et al. (2013) on cucumber; the latter authors found that once the photosynthetic capacity was too low to use all incident radiation absorbed, excess excitation energy was dissipated in the thylakoids to reduce photoinhibition. Shu et al. (2013) also reported reductions in Chl a, Chl b, and total chlorophyll in leaves of salt-stressed cucumber that were similar to, but not as dramatic as the reductions in black locust chloroplast chlorophyll levels that we observed (Table 1).
Another indication that specific ion stress, rather than osmotic stress, was primarily responsible for the negative impacts on black locust seedlings is seen in the cell membrane stability index (MSI), which was sharply reduced by salt stress (Fig. 5a). Talaat and Shawky (2012) observed a similar reduction in MSI in salt-stressed wheat, but in contrast to our results there was also a substantial decrease in RWC. We found that Na+ concentrations of intact leaf tissue and isolated chloroplasts roughly paralleled each other and were increased several fold (more in chloroplasts) when black locust seedlings were grown with NaCl (Fig. 4). Similarly, Wang et al. (2007) reported large accumulations of Na+ and Cl− in chloroplasts of salt-stressed poplar. In the present work, there was a simultaneous increase in chloroplast H2O2 and MDA (Table 2), indicative of oxidative stress. Diao et al. (2014) observed similar increases in chloroplast H2O2 and MDA levels in salt-stressed tomato. We also measured APX and SOD activity, but changes in these activities were less consistent. The efficiency of the ROS scavenging system is believed to play an important role in protecting cells from oxidative damage (Mittler 2002). It is unclear whether the oxidative stress was a symptom or cause of the increase in MSI and/or photoinhibition, but the three symptoms are likely to be related. Agrawal et al. (2013) observed a similar overall syndrome in response to salinity in leaves of the Indian jujube (Ziziphus mauritiana L.) tree. In black locust, this syndrome is accompanied by changes in chloroplast ultrastructure (Fig. 3), including chloroplast swelling, disruption of thylakoid membranes, larger but fewer plastoglobuli, and smaller, less distinct starch granules.
Similar effects on thylakoid membranes were apparent in potato (Gao et al. 2014) and rice plants (Yamane et al. 2008) exposed to salinity, in the latter case in association with decreases in Fv/Fm and increases in Fo. Likewise, drought stress caused chloroplast swelling and disrupted the lamellar arrangement of thylakoids in poplar (Zhang et al. 2012) but, in contrast to the effects of salt stress on black locust, increased not only the size but also the number of plastoglobuli.
Protective Effects of 24-epiBL
Beyond some increases in NPQ and Qp, and higher activities of APX and SOD, there were no observable effects of our 24-epiBL pretreatments on unstressed black locust seedlings. There were, however, very strong positive effects of 24-epiBL on the salt-stressed seedlings. The most dramatic results were a complete or almost complete reversal of the effects of salinity stress on An, MSI, and WUE. The positive effect on WUE in combination with little change in gs suggested that 24-epiBL promotes photosynthesis at the level of the mesophyll, by protecting the photosynthetic apparatus and by helping to maintain gm. This is consistent with the maintenance of MSI, RWC, and the positive effects of 24-epiBL on chloroplasts (improved shape and positioning, lower Na+ content, increased numbers of plastoglobuli and enhanced condition of thylakoid membranes), less severe reductions in chlorophylls, and improvements in Fv/Fm. Pretreatment with 24-epiBL also reduced levels of oxidative stress, as evidenced by reduced levels of H2O2 and MDA in the salt-stressed plants.
Although both 24-epiBL pretreatment protocols provided substantial protection, seed soaking (Sew) was somewhat less effective than root dipping (Diw), particularly at the highest stress level (200 mM NaCl). Root dipping resulted in better maintenance of total chlorophyll concentrations, higher Fv/Fm, higher Fv/Fo, lower foliar Na+, lower MDA levels, lower H2O2 levels, and greater SOD activity. The lower effectiveness of seed soaking was likely caused by a greater dilution of applied 24-epiBL by biomass accretion and the greater time since treatment, an intervening period of over 6 months.
Many authors report similar effects of exogenous 24-epiBL in alleviating the symptoms of salt and other stresses. Wu et al. (2017) reported that spraying the foliage of perennial ryegrass seedlings with 24-epiBL could increase their chlorophyll content, An, gs, and antioxidant enzyme activities to protect them from salinity. Treatment of salt-stressed barley seedlings with 24-epiBL significantly increased growth and RWC, and improved salinity tolerance by reducing K+ efflux from roots via depolarization-activated K+ channels (Azhar et al. 2017). Similar to the present paper, but working with a moderately salt-sensitive cultivar of wheat, Ali et al. (2008b) concluded that improvements in photosynthesis induced by pretreatment with 24-epiBL were related to non-stomatal factors and the removal of biochemical limitations in the mesophyll.
Under drought conditions induced by polyethylene glycol (PEG), Wang et al. (2015) reported that application of 24-epiBL to grape seedlings increased their chlorophyll content, maximal fluorescence, Fv/Fm, and NPQ. At the same time, chloroplasts were less swollen and the lamellae maintained a relatively normal appearance. Treatment with 24-epiBL similarly reduced the effects of PEG-induced water stress in blue mustard (Chorispora bungeana Fisch. & C.A. Mey.) plants as evidenced by higher Fv/Fm and Chl a content, accompanied by up-regulation of the ROS scavenging system and reduced lipid peroxidation (Li et al. 2012). The latter function might reflect the involvement of BRs in modifying plasma membrane stability during stress (Hamada 1986). Ahammed et al. (2013) showed that pretreatment of tomato seedlings with 24-epiBL improved the ability of the ROS scavenging system to reduce the harm caused by H2O2 accumulation and membrane lipid peroxidation upon exposure to polychlorinated biphenyls.
Although BRs are widely considered to regulate tolerance to various stresses in plants, little is known regarding the underlying mechanisms. Proximally, they may act in a manner similar to other agents where improved salt tolerance has been associated with many of the same physiological and structural changes reported here. For example, in studying the effects of silicon and salt stress on Japanese honeysuckle (Lonicera japonica L.), Gengmao et al. (2015) observed that exogenous K2SiO3 helped to maintain chloroplast lamellae and reduce foliar Na+ levels, leading to increased An. Hu et al. (2014) reported similar effects of Si in maintaining chloroplast ultrastructure (including plastoglobuli and starch grains) in salt-stressed tomato accompanied by an improvement in An and Qp, and reduced NPQ, in parallel with increased gs and decreased Ls, but without any change in Ci. Similarly, Shu et al. (2013) reported that application of spermine to salt-stressed cucumber improved chlorophyll fluorescence parameters and increased the activities of antioxidant enzymes and levels of antioxidants in chloroplasts. Mycorrhization of poplars by Paxillus involutus (Batsch) Fr. improved their salt tolerance by reducing the amounts of Na+ in leaves (Luo et al. 2011). Higher salinity tolerance in Euphrates poplar (Populus euphratica Oliv.) relative to a hybrid cultivar (P. × popularis) was associated with a lower Na+ content in the chloroplasts of the former (Wang et al. 2007).
Conclusion
In conclusion, our results show that salt stress induces photoinhibition in black locust seedlings. Exogenous 24-epiBL pretreatments alleviated the stress-induced inhibition of photosynthesis by reducing Na+-ion toxicity and ROS in chloroplasts, and by stabilizing chloroplast and thylakoid membrane structure. Applications of 24-epiBL by seed soaking or by root dipping were both effective, but plants produced using the latter method performed somewhat better under salt stress. The beneficial influence of 24-epiBL was relatively long-lasting (several months from time of application), and potentially long enough to promote establishment in practice, on salt-affected soils in agroforestry applications in the field.
References
Abo-Ogiala A, Carsjens C, Diekmann H, Fayyaz P, Herrfurth C, Feussner I, Polle A (2014) Temperature-induced lipocalin (TIL) is translocated under salt stress and protects chloroplasts from ion toxicity. J Plant Physiol 171:250–259. https://doi.org/10.1016/j.jplph.2013.08.003
Agrawal R, Gupta S, Gupta NK, Khandelwal SK, Bhargava R (2013) Effect of sodium chloride on gas exchange, antioxidative defense mechanism and ion accumulation in different cultivars of Indian jujube (Ziziphus mauritiana L.). Photosynthetica 51:95–101. https://doi.org/10.1007/s11099-013-0003-8
Ahammed GJ, Ruan YP, Zhou J, Xia XJ, Shi K, Zhou YH, Yu JQ (2013) Brassinosteroid alleviates polychlorinated biphenyls-induced oxidative stress by enhancing antioxidant enzymes activity in tomato. Chemosphere 90:2645–2653. https://doi.org/10.1016/j.chemosphere.2012.11.041
Akatov VV, Akatova TV, Shadzhe AE (2016) Robinia pseudoacacia L. in the Western Caucasus. Russ J Biol Invasions 7:105–118. https://doi.org/10.1134/S2075111716020028
Ali B, Hayat S, Fariduddin Q, Ahmad A (2008a) 24-Epibrassinolide protects against the stress generated by salinity and nickel in Brassica juncea. Chemosphere 72:1387–1392. https://doi.org/10.1016/j.chemosphere.2008.04.012
Ali Q, Athar HUR, Ashraf M (2008b) Modulation of growth, photosynthetic capacity and water relations in salt stressed wheat plants by exogenously applied 24-epibrassinolide. Plant Growth Regul 56:107–116. https://doi.org/10.1007/s10725-008-9290-7
Arnon D (1949) Copper enzymes in isolated chloroplasts. Polyphenoloxidase in Beta vulgaris. Plant Physiol 20:1–16
Azhar N, Su N, Shabala L, Shabala S (2017) Exogenously applied 24-epibrassinolide (EBL) ameliorates detrimental effects of salinity by reducing K+ efflux via depolarization-activated K+ channels. Plant Cell Physiol 58:802–810. https://doi.org/10.1093/pcp/pcx026
Bartels D, Sunkar R (2005) Drought and salt tolerance in plants. CRC Crit Rev Plant Sci 24:23–58. https://doi.org/10.1080/07352680590910410
Beyer WF, Fridovich I (1987) Assaying for superoxide dismutase activity: some large consequences of minor changes in conditions. Anal Biochem 161:559–566. https://doi.org/10.1016/0003-2697(87)90489-1
Cerović ZG, Plesnicar M (1984) An improved procedure for the isolation of intact chloroplasts of high photosynthetic capacity. Biochem J 223:543–545
Choudhary SP, Kanwar M, Bhardwaj R, Gupta BD, Gupta RK (2011) Epibrassinolide ameliorates Cr (VI) stress via influencing the levels of indole-3-acetic acid, abscisic acid, polyamines and antioxidant system of radish seedlings. Chemosphere 84:592–600. https://doi.org/10.1016/j.chemosphere.2011.03.056
Cui L, Zou Z, Zhang J, Zhao Y, Yan F (2016) 24-Epibrassinoslide enhances plant tolerance to stress from low temperatures and poor light intensities in tomato (Lycopersicon esculentum Mill.). Funct Integr Genomics 16:29–35. https://doi.org/10.1007/s10142-015-0464-x
DeGomez T, Wagner MR (2001) Culture and use of black locust. Horttechnology 11:279–288
Diao M, Ma L, Wang J, Cui J, Fu A, Liu H (2014) Selenium promotes the growth and photosynthesis of tomato seedlings under salt stress by enhancing chloroplast antioxidant defense system. J Plant Growth Regul 33:671–682. https://doi.org/10.1007/s00344-014-9416-2
Divi UK, Krishna P (2009) Brassinosteroid: a biotechnological target for enhancing crop yield and stress tolerance. N Biotechnol 26:131–136. https://doi.org/10.1016/j.nbt.2009.07.006
Ellis T, van Dijk A (2009) Agroforestry for the management of water, salt and agricultural diffuse source pollutants. In: Nuberg I, George B, Reid R (eds) Agroforestry for natural resource management. CSIRO, Collingwood, pp 53–68
Flexas J, Medrano H (2002) Drought-inhibition of photosynthesis in C3 plants: stomatal and non-stomatal limitations revisited. Ann Bot 89:183–189. https://doi.org/10.1093/aob/mcf027
Gao HJ, Yang HY, Bai JP, Liang XY, Lou Y, Zhang JL, Wang D, Zhang JL, Niu SQ, Chen YL (2014) Ultrastructural and physiological responses of potato (Solanum tuberosum L.) plantlets to gradient saline stress. Front Plant Sci 5:787. https://doi.org/10.3389/fpls.2014.00787
Gengmao Z, Shihui L, Xing S, Yizhou W, Zipan C (2015) The role of silicon in physiology of the medicinal plant (Lonicera japonica L.) under salt stress. Sci Rep 5:12696. https://doi.org/10.1038/srep12696
Grove MD, Spencer GF, Rohwedder WK, Mandava N, Worley JF, Warthen JD Jr, Steffens GL, Flippen-Anderson JL, Cook JC Jr (1979) Brassinolide, a plant growth-promoting steroid isolated from Brassica napus pollen. Nature 281:216–217
Hajibagheri MA, Flowers TJ (1984) Photosynthetic oxygen evolution in relation to ion contents in the chloroplasts of Suaeda maritima. Plant Sci Lett 34:353–362
Hamada K (1986) Brassinolide in crop cultivation. Plant growth regulators in agriculture. FFTC Book Ser 34:188–196
Hayat S, Ali B, Hasan SA, Ahmad A (2007) Brassinosteroid enhanced the level of antioxidants under cadmium stress in Brassica juncea. Environ Exp Bot 60:33–41. https://doi.org/10.1016/j.envexpbot.2006.06.002
Hayat S, Hasan SA, Yusuf M, Hayat Q, Ahmad A (2010) Effect of 28-homobrassinolide on photosynthesis, fluorescence and antioxidant system in the presence or absence of salinity and temperature in Vigna radiata. Environ Exp Bot 69:105–112. https://doi.org/10.1016/j.envexpbot.2010.03.004
Heath RL, Packer L (1968) Photoperoxidation in isolated chloroplasts. Arch Biochem Biophys 125:189–198. https://doi.org/10.1016/0003-9861(68)90654-1
Hoagland DR, Arnon DI (1950) The water-culture method for growing plants without soil. Calif Agric Exp Stn Circ 347:1–32. doi: citeulike-article-id:9455435
Hu L, Xiang L, Zhang L, Zhou X, Zou Z, Hu X (2014) The photoprotective role of spermidine in tomato seedlings under salinity-alkalinity stress. PLoS ONE 9:e110855. https://doi.org/10.1371/journal.pone.0110855
Jiang YP, Huang LF, Cheng F, Zhou YH, Xia XJ, Mao WH, Shi K, Yu JQ (2013) Brassinosteroids accelerate recovery of photosynthetic apparatus from cold stress by balancing the electron partitioning, carboxylation and redox homeostasis in cucumber. Physiol Plant 148:133–145. https://doi.org/10.1111/j.1399-3054.2012.01696.x
Kalaji HM, Govindjee, Bosa K, Kościelniak J, Żuk-Gołaszewska K (2011) Effects of salt stress on photosystem II efficiency and CO2 assimilation of two Syrian barley landraces. Environ Exp Bot 73:64–72. https://doi.org/10.1016/j.envexpbot.2010.10.009
Kemmerling B, Schwedt A, Rodriguez P, Mazzotta S, Frank M, Qamar SA, Mengiste T, Betsuyaku S, Parker JE, Müssig C, Thomma BPHJ, Albrecht C, de Vries SC, Hirt H, Nürnberger T (2007) The BRI1-associated kinase 1, BAK1, has a brassinolide-independent role in plant cell-death control. Curr Biol 17:1116–1122. https://doi.org/10.1016/j.cub.2007.05.046
Li YH, Liu YJ, Xu XL, Jin M, An LZ, Zhang H (2012) Effect of 24-epibrassinolide on drought stress-induced changes in Chorispora bungeana. Biol Plant 56:192–196. https://doi.org/10.1007/s10535-012-0041-2
Li JY, Zhao CY, Li J, Yan YY, Yu B, Han M (2013) Growth and leaf gas exchange in Populus euphratica across soil water and salinity gradients. Photosynthetica 51:321–329. https://doi.org/10.1007/s11099-013-0028-z
Li J, Pu L, Han M, Zhu M, Zhang R, Xiang Y (2014) Soil salinization research in China: advances and prospects. J Geogr Sci 24:943–960. https://doi.org/10.1007/s11442-014-1130-2
Li X, Ahammed GJ, Li ZX, Zhang L, Wei JP, Shen C, Yan P, Zhang LP, Han WY (2016) Brassinosteroids improve quality of summer tea (Camellia sinensis L.) by balancing biosynthesis of polyphenols and amino acids. Front Plant Sci 7:1304. https://doi.org/10.3389/fpls.2016.01304
Liu WN, Jiang HY, Yang MS (2010) Selection of salt-tolerance on Robinia pseudoacacia seedings and impact of salt stress on seedlings’ physiological characteristics. J Agric Univ Hebei 3:62–66. (in Chinese)
Luo Z, Li K, Gai Y, Göbel C, Wildhagen H, Jiang X, Feußner I, Rennenberg H, Polle A (2011) The ectomycorrhizal fungus (Paxillus involutus) modulates leaf physiology of poplar towards improved salt tolerance. Environ Exp Bot 72:304–311. https://doi.org/10.1016/j.envexpbot.2011.04.008
Milla-Moreno EA, McKown AD, Guy RD, Soolanayakanahally RY (2016) Leaf mass per area predicts palisade structural properties linked to mesophyll conductance in balsam poplar (Populus balsamifera L.). Botany 94:225–239. https://doi.org/10.1139/cjb-2015-0219
Mitchell JW, Mandava N, Worley JF, Plimmer JR, Smith MV (1970) Brassins—a new family of plant hormones from rape pollen. Nature 225:563–564
Mittler R (2002) Oxidative stress, antioxidants and stress tolerance. Trends Plant Sci 7:405–410. https://doi.org/10.1016/S1360-1385(02)02312-9
Nakano Y, Asada K (1981) Hydrogen peroxide is scavenged by ascorbate-specific peroxidase in spinach chloroplasts. Plant Cell Physiol 22:867–880. https://doi.org/10.1093/oxfordjournals.pcp.a076232
Oxborough K (2004) Imaging of chlorophyll a fluorescence: theoretical and practical aspects of an emerging technique for the monitoring of photosynthetic performance. J Exp Bot 55:1195–1205. https://doi.org/10.1093/jxb/erh145
Park CH, Kim TW, Son SH, Hwang JY, Lee SC, Chang SC, Kim SH, Kim SW, Kim SK (2010) Brassinosteroids control AtEXPA5 gene expression in Arabidopsis thaliana. Phytochemistry 71:380–387. https://doi.org/10.1016/j.phytochem.2009.11.003
Patterson BD, MacRae EA, Ferguson IB (1984) Estimation of hydrogen peroxide in plant extracts using titanium (IV). Anal Biochem 139:487–492. https://doi.org/10.1016/0003-2697(84)90039-3
Qadir M, Oster JD (2004) Crop and irrigation management strategies for saline-sodic soils and waters aimed at environmentally sustainable agriculture. Sci Tot Environ 323:1–19. https://doi.org/10.1016/j.scitotenv.2003.10.012
Rahmonov O (2009) The chemical composition of plant litter of black locust (Robinia pseudoacacia L.) and its ecological role in sandy ecosystems. Shengtai Xuebao/Acta Ecol Sin 29:237–243. https://doi.org/10.1016/j.chnaes.2009.08.006
Ramakrishna B, Rao SSR (2012) 24-Epibrassinolide alleviated zinc-induced oxidative stress in radish (Raphanus sativus L.) seedlings by enhancing antioxidative system. Plant Growth Regul 68:249–259. https://doi.org/10.1007/s10725-012-9713-3
Roháček K (2002) Chlorophyll fluorescence parameters: the definitions, photosynthetic meaning, and mutual relationships. Photosynthetica 40:13–29. https://doi.org/10.1023/A:1020125719386
Sairam RK (1994) Effects of homobrassinolide application on plant metabolism and grain yield under irrigated and moisture-stress conditions of two wheat varieties. Plant Growth Regul 306:173–181. https://doi.org/10.1007/BF00025220
Sayed OH (2003) Chlorophyll fluorescence as a tool in cereal crop research. Photosynthetica 41:321–330. https://doi.org/10.1023/B:PHOT.0000015454.36367.e2
Shahid SA (2013) Developments in soil salinity assessment, modeling, mapping and monitoring from regional to submicroscopic scales. In: Shahid SA, Abdelfattah MA, Taha FK (eds) Developments in soil salinity assessment and reclamation. Springer, Dordrecht, pp 3–43. https://doi.org/10.1007/978-94-007-5684-7
Sharma I, Kaur N, Pati PK (2017) Brassinosteroids: a promising option in deciphering remedial strategies for abiotic stress tolerance in rice. Front Plant Sci 8:2151. https://doi.org/10.3389/fpls.2017.02151
Shu S, Yuan LY, Guo SR, Sun J, Yuan YH (2013) Effects of exogenous spermine on chlorophyll fluorescence, antioxidant system and ultrastructure of chloroplasts in Cucumis sativus L. under salt stress. Plant Physiol Biochem 63:209–216. https://doi.org/10.1016/j.plaphy.2012.11.028
Sitzia T, Campagnaro T, Dainese M, Cierjacks A (2012) Plant species diversity in alien black locust stands: a paired comparison with native stands across a north-Mediterranean range expansion. For Ecol Manag 285:85–91. https://doi.org/10.1016/j.foreco.2012.08.016
Song XS, Tiao CL, Shi K, Mao WH, Ogweno JO, Zhou YH, Yu JQ (2006) The response of antioxidant enzymes in cellular organelles in cucumber (Cucumis sativus L.) leaves to methyl viologen-induced photo-oxidative stress. Plant Growth Regul 49:85–93. https://doi.org/10.1007/s10725-006-0023-5
Sudhir P, Murthy SDS (2004) Effects of salt stress on basic processes of photosynthesis. Photosynthetica 42:481–486. https://doi.org/10.1007/S11099-005-0001-6
Talaat NB, Shawky BT (2012) 24-Epibrassinolide ameliorates the saline stress and improves the productivity of wheat (Triticum aestivum L.). Environ Exp Bot 82:80–88. https://doi.org/10.1016/j.envexpbot.2012.03.009
Tao Y, Yu QX, Zhou YH, Shi K, Zhou J, Yu JQ, Xia XJ (2015) Application of 24-epibrassinolide decreases the susceptibility to cucumber mosaic virus in zucchini (Cucurbita pepo L). Sci Hortic 195:116–123. https://doi.org/10.1016/j.scienta.2015.09.005
Tateno R, Tokuchi N, Yamanaka N, Du S, Otsuki K, Shimamura T, Xue Z, Wang S, Hou Q (2007) Comparison of litterfall production and leaf litter decomposition between an exotic black locust plantation and an indigenous oak forest near Yan’an on the Loess Plateau, China. For Ecol Manag 241:84–90. https://doi.org/10.1016/j.foreco.2006.12.026
Thakur P, Kumar S, Malik JA, Berger JD, Nayyar H (2010) Cold stress effects on reproductive development in grain crops: an overview. Environ Exp Bot 67:429–443. https://doi.org/10.1016/j.envexpbot.2009.09.004
Thussagunpanit J, Jutamanee K, Sonjaroon W, Kaveeta L, Chai-Arree W, Pankean P, Suksamrarn A (2015) Effects of brassinosteroid and brassinosteroid mimic on photosynthetic efficiency and rice yield under heat stress. Photosynthetica 53:312–320. https://doi.org/10.1007/s11099-015-0106-5
Wang W, Vinocur B, Altman A (2003) Plant responses to drought, salinity and extreme temperatures: towards genetic engineering for stress tolerance. Planta 218:1–14. https://doi.org/10.1007/s00425-003-1105-5
Wang R, Chen S, Deng L, Fritz E, Hüttermann A, Polle A (2007) Leaf photosynthesis, fluorescence response to salinity and the relevance to chloroplast salt compartmentation and anti-oxidative stress in two poplars. Trees Struct Funct 21:581–591. https://doi.org/10.1007/s00468-007-0154-y
Wang Z, Wang M, Liu L, Meng F (2013) Physiological and proteomic responses of diploid and tetraploid black locust (Robinia pseudoacacia L.) subjected to salt stress. Int J Mol Sci 14:20299–20325. https://doi.org/10.3390/ijms141020299
Wang Z, Zheng P, Meng J, Xi Z (2015) Effect of exogenous 24-epibrassinolide on chlorophyll fluorescence, leaf surface morphology and cellular ultrastructure of grape seedlings (Vitis vinifera L.) under water stress. Acta Physiol Plant 37:1729. https://doi.org/10.1007/s11738-014-1729-z
Wei LJ, Deng XG, Zhu T, Zheng T, Li PX, Wu JQ, Zhang DW, Lin HH (2015) Ethylene is involved in brassinosteroids induced alternative respiratory pathway in cucumber (Cucumis sativus L.) seedlings response to abiotic stress. Front Plant Sci 6:982. https://doi.org/10.3389/fpls.2015.00982
Wu X, Zhu Z, Li X, Zha D (2012) Effects of cytokinin on photosynthetic gas exchange, chlorophyll fluorescence parameters and antioxidative system in seedlings of eggplant (Solanum melongena L.) under salinity stress. Acta Physiol Plant 34:2105–2114. https://doi.org/10.1007/s11738-012-1010-2
Wu X, Yao X, Chen J, Zhu Z, Zha D (2014) Brassinosteroids protect photosynthesis and antioxidant system of eggplant seedlings from high-temperature stress. Acta Physiol Plant 36:251–261. https://doi.org/10.1007/s11738-013-1406-7
Wu W, Zhang Q, Ervin EH, Yang Z, Zhang X (2017) Physiological mechanism of enhancing salt stress tolerance of perennial ryegrass by 24-epibrassinolide. Front Plant Sci 8:1–11. https://doi.org/10.3389/fpls.2017.01017
Xi Z, Wang Z, Fang Y, Hu Z, Hu Y, Deng M, Zhang Z (2013) Effects of 24-epibrassinolide on antioxidation defense and osmoregulation systems of young grapevines (V. vinifera L.) under chilling stress. Plant Growth Regul 71:57–65. https://doi.org/10.1007/s10725-013-9809-4
Xu G, Huang TF, Zhang XL, Duan BL (2013) Significance of mesophyll conductance for photosynthetic capacity and water-use efficiency in response to alkaline stress in Populus cathayana seedlings. Photosynthetica 51:438–444. https://doi.org/10.1007/s11099-013-0043-0
Yamane K, Kawasaki M, Taniguchi M, Miyake H (2008) Correlation between chloroplast ultrastructure and chlorophyll fluorescence characteristics in the leaves of rice (Oryza sativa L.) grown under salinity. Plant Prod Sci 11:139–145. https://doi.org/10.1626/pps.11.139
Zhang S, Chen L, Duan BL, Korpelainen H, Li C (2012) Populus cathayana males exhibit more efficient protective mechanisms than females under drought stress. For Ecol Manag 275:68–78. https://doi.org/10.1016/j.foreco.2012.03.014
Zhang YP, Zhu XH, Ding HD, Yang SJ, Chen YY (2013) Foliar application of 24-epibrassinolide alleviates high-temperature-induced inhibition of photosynthesis in seedlings of two melon cultivars. Photosynthetica 51:341–349. https://doi.org/10.1007/s11099-013-0031-4
Zhu T, Deng XG, Tan WR, Zhou X, Luo SS, Han XY, Zhang DW, Lin HH (2015) Nitric oxide is involved in brassinosteroid-induced alternative respiratory pathway in Nicotiana benthamiana seedlings’ response to salt stress. Physiol Plant 156:150–163. https://doi.org/10.1111/ppl.12392
Acknowledgements
We would like to thank Fangyuan Yu and Dawei Shi for their constructive suggestions on experimental design. Financial support for this study was provided by the National Special Fund for Forestry Scientific Research in the Public Interest (Grant No. 201504406), the New Project for Forestry Research of Jiangsu Province, China (Grant No. LY-SX[2014]05), Science and Technology Project of Jiangsu Province, China (Grant No. BE2012344), Major Projects of Natural Science Research in Universities in Jiangsu Province, China (Grant No. 15KJA220004), the Priority Academic Program Development of Jiangsu Higher Education Institutions (PAPD), and the Doctorate Fellowship Foundation of Nanjing Forestry University.
Author information
Authors and Affiliations
Corresponding authors
Ethics declarations
Conflict of interest
The authors declare that they have no conflicts of interest.
Rights and permissions
About this article
Cite this article
Yue, J., You, Y., Zhang, L. et al. Exogenous 24-Epibrassinolide Alleviates Effects of Salt Stress on Chloroplasts and Photosynthesis in Robinia pseudoacacia L. Seedlings. J Plant Growth Regul 38, 669–682 (2019). https://doi.org/10.1007/s00344-018-9881-0
Received:
Accepted:
Published:
Issue Date:
DOI: https://doi.org/10.1007/s00344-018-9881-0