Abstract
In this paper, 1D single-crystalline MnO2 nanowires have been successfully synthesized by hydrothermal method using KMnO4 and (NH4)2S2O8 as raw materials. X-ray diffraction patterns and high-resolution TEM images reveal pure tetragonal MnO2 phase with diameters of 15–20 nm. Photoluminescence studies exhibited a strong ultraviolet (UV) emission band at 380 nm, blue emission at 452 nm and an extra weak defect-related green emission at 542 nm. UV–visible spectrophotometery was used to determine the absorption behavior of nanostructured MnO2 and a direct optical band gap of 2.5 eV was acquired by Davis–Mott model. The magnetic properties of the products have been evaluated using vibrating sample magnetometer, which showed that MnO2 nanowires exhibited a superparamagnetic behavior at room temperature. The magnetization versus temperature curve of the as-obtained MnO2 nanowires shows that antiferromagnetic transition temperature is 99 K.
Similar content being viewed by others
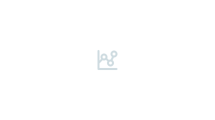
Avoid common mistakes on your manuscript.
1 Introduction
Synthesis of nanostructured materials with controlled size, architecture and shape is of importance to ensure their property performance in various device applications such as optics, magnetism, mechanics and electronics [1]. Low-dimensional nanostructures exhibit distinct properties that are different from those of bulk materials due to their small size and large surface-to-volume ratios [2], because the size of nanoparticles plays a crucial role while tailoring the properties of semiconductor nanomaterials [3].
One-dimensional (1D) nanoarchitectures such as nanowires, nanorods and nanotubes have attracted exceptional interest due to their potential applications as conducting interconnectors and nanoscale electronic, optoelectronic and sensing devices [4], but for optoelectronics applications, it is highly desirable to fabricate luminescent materials that are compatible with the existing developed nanotechnology [5]. For a single absorber system, a band gap of 1.8–2.2 eV is considered optimal for balancing the need to have a semiconductor photoelectrode which absorbs strongly in the visible portion of the solar spectrum. In our previous work, a direct optical band gap of 1.65 eV was calculated for hexagonal MnO2 nanostructures [6]. Furthermore, the evidence of photoreductive dissolution of manganese minerals has led researchers to study the semiconducting nature of manganese oxides [7]. In terms of magnetism, geometrically frustrated systems often exhibit unusual magnetic properties that stem from their enormous ground-state spin degeneracies; therefore, it is important to review the characteristics of conventional antiferromagnets in contrast to geometrically frustrated systems [8].
Manganese dioxide is of considerable importance in advanced technology applications such as catalysis, molecular sieves, as electrodes in rechargeable batteries [9] and soft magnetic materials due to its outstanding structural flexibility combined with novel chemical and physical properties [10]. A tetragonal rutile-type β-MnO2 is the most stable form of MnO2 in nature [11] among several α-, β-, γ- and λ-types MnO2, produced when the basic structure unit [MnO6] octahedron is linked in different ways [12].
Among various successful chemical synthetic routes, hydrothermal method has been facilely used to fabricate manganese oxide nanomaterials because of the choice of solvents, homogeneous reaction conditions and control of reaction time and temperature. At present, although many researchers have focused on the synthesis of 1D MnO2 nanostructures and its polymorphic forms [13–25], however, the exploration of the mechanism of MnO2 nanostructural magnetic behaviors, characteristic photoluminescence (PL) and ultraviolet–visible absorption properties in detail still remains a major challenge.
Herein we report a facile hydrothermal route to synthesize 1D single-crystalline high-quality MnO2 nanowires at a reaction temperature of 180 °C for 15 h in which (NH4)2S2O8 was used as an oxidizing agent for KMnO4. A direct optical band gap of 2.5 eV was calculated for the as-prepared MnO2 nanowires. The PL spectrum exhibited ultraviolet (UV) and blue emission bands at 380 and 452 nm and a weak defect-related green emission band at 542 nm. These optical properties indicate that the as-prepared MnO2 nanowires have promise for application in optoelectronic UV and visible emission devices. Furthermore, field-dependent and temperature-dependent magnetic properties of the product have been evaluated and discussed in detail.
2 Experimental details
2.1 Sample preparation
All reagents were commercially available and used without further purification. In a typical procedure, 2 g of KMnO4 was added to 40 ml of deionized water and magnetically stirred for 20 min. While stirring, 1 g of (NH4)2S2O8 and 0.5 ml of HNO3 were added and stirred ultrasonically for another 15 min. The resultant solution was calcined in a stainless autoclave at 180 °C for 15 h under constant pressure. The autoclave was allowed to cool down at room temperature. After completion of the reaction the prepared product was washed with distilled water several times, filtered, centrifuged and dried at 90 °C for 5 h in an oven. Finally, the dried product was grounded and mixed thoroughly in mortar and pestle.
2.2 Sample characterizations
The initial morphology and composition of the resultant product were imaged by field emission scanning electron microscope (Hitachi S-3500-SEM) operated at 15 kV and energy dispersive X-ray spectrometer (Hitachi S-3500-EDX) using 32 eV beam resolution from 100 to 500 cps. Crystallinity, phase and diameter of the as-prepared nanostructures were investigated by X-ray diffractometer (X’Pert MPD-XRD) using Cu K α radiation (λ = 0.154 nm), transmission electron microscope (JEM 2010-TEM), high-resolution TEM (JEM 2010-HRTEM), and selected area electron diffraction (SAED JEM 2010). PL (F-4500 FL spectrophotometer) and UV–visible spectroscopy (TU-1901) were performed to study the optical properties of the synthesized material. Magnetic measurements were carried out using vibrating sample magnetometer (VSM) option of Quantum Design Versa Lab.
3 Results and discussion
The XRD pattern of MnO2 nanowires is depicted in Fig. 1a. All the diffraction peaks are well indexed to the tetragonal structure (JCPDS file No. 44-0141) with lattice constants of a = b = 9.785 A, c = 2.863 A and α = β = γ = 90o. A sharp [211] strong diffraction peak depicted in Fig. 1a demonstrates that the MnO2 nanowires are well crystallized in the [211] lattice planes. No impurity peak is observed which indicates the high purity of the as-prepared products. The average crystalline size was estimated using Debye–Scherrer formula:
where K = 0.9 is the shape factor for tetragon, λ is the X-ray wavelength (1.5406°A for Cu K α ), β is the full-width at half maximum (FWHM) in radians and θ is the position of the maximum diffraction peak. Using the above equation, the average grain size was calculated to be about 10 nm. The EDS analysis shown in Fig. 1b (right upper inset of Fig. 1) manifests that the final product contains only Mn and O suggesting that the nanowires were made up of pure MnO2.
The morphology of the as-prepared MnO2 nanowires was primarily investigated by FESEM. The low- and high-magnification FESEM images shown in Fig. 2a–c reveal that the as-prepared tetragonal MnO2 consists of uniform, smooth and high-quality nanowires with diameters of 15–20 nm and lengths up to several micrometers. The TEM and HRTEM were used to get further information regarding the structural aspects of tetragonal MnO2 nanowires. Figure 2d, e illustrates the low- and high-magnification TEM images of MnO2 nanowires, which are in agreement with the FESEM observations. The HRTEM image taken from a single nanowire given in Fig. 2f demonstrated the single set of parallel planes with inter-planer spacing of 0.21 nm which is in good agreement with the [211] plane in the XRD spectrum. The single-crystalline structure of as-prepared tetragonal MnO2 nanowires is also confirmed by the SAED pattern shown in the lower inset of Fig. 2f.
3.1 Optical properties
To determine the optical properties, we have investigated the PL emission and UV–visible absorption spectra of hydrothermally grown MnO2 nanowires. Figure 3a displays the PL spectra of MnO2 nanowires measured at room temperature using a Xe excitation source with an excitation wavelength of 300 nm. As indicated in the figure, the spectrum exhibits prominent emission bands located in green–violet spectral region. Through the Gaussian curve fitting, it was clear that three signal peaks were located at 380, 452 and 542 nm. The PL spectrum exhibits a very strong UV emission band at 380 nm, a strong blue emission band at 452 nm and an extra weak green peak at 542 nm. The strong UV emission corresponds to the band edge emission signals of MnO2 [26] of the well-crystallized crystals. The blue emission at 452 nm may be assigned to the oxygen vacancy-related defects and an additional broad and weak green emission at 542 nm can be attributed to surface dangling bonds or surface defects [27]. Kannan et al. [28] reported two PL emission bands located at 417 and 437 nm and for CdS-MnO2. Recently, the PL emissions located in the blue–violet spectral region have been reported for β-MnO2 [26]. Hence, in addition to the reported violet–blue band emissions in MnO2 nanostructures observed by many researchers, an extra green peak at around 542 nm has been reported in our work. These PL emission characteristics demonstrate the promise of as-synthesized MnO2 nanowires for practical applications such as for UV and visible light emission devices.
It is well-known that optical absorption behavior significantly affects the photocatalyic activity of photocatalysts. In general, semiconductors with higher energy gaps tend to show better photostability but relatively lower solar energy-use efficiency, whereas narrow band gap semiconductors tend to have high solar energy efficiency but their photostability is relatively lower [26]. Therefore, to better understand the optical properties, UV–visible absorption spectroscopy was investigated for the as-prepared MnO2 nanowires and the absorption was found maximum in the visible region as shown in Fig. 3b. From the spectra, it can be seen that the nanostructured MnO2 has an absorption band in the wavelength range of 210–400 nm and a broad absorption band in the region of 410–800 nm, respectively. The absorption band in UV region may be attributed to the electron transition from valence bands to conduction bands [29] and the optical absorption of MnO2 nanostructures in the visible light range originated mainly from d–d transitions of Mn ions [3].
The band gap of tetragonal MnO2 nanowires was probed optically via UV–visible absorption spectroscopy plotted in Fig. 3b using a well-known Tauc and Davis–Mott relation [6]:
where A is a constant, hv is the photon energy and α is the absorption coefficient, while n = 2 for direct interband transition and n = ½ for indirect transition. The best fit of the experimental curve to a band gap semiconductor function was obtained for n = 2 to calculate the direct band gap energy values. The straight line part of the spectrum in (αhv)2 versus hv graph is plotted in Fig. 3c in which the value of direct band gap was calculated by extrapolating the linear part of curve to energy axis at α = 0 and found to be 2.5 eV for the synthesized nanostructured MnO2. The calculated band gap (2.5 eV) is higher than the reported values for nanostructured MnO2 [30, 31]. This high value may be attributed to the small crystallite size and better quantum confinement effect of MnO2 crystals reported here. Based on the above experimental results, we can say that the observed UV–visible absorption properties and a moderate band gap value of 2.5 eV make this photostable material potentially interesting for application in optoelectronic UV and visible emission devices.
3.2 Magnetic properties
We investigated the magnetic properties of as-obtained tetragonal MnO2 nanowires using a VSM. Figure 4a shows the zero-field cooling (ZFC) and field cooling (FC) magnetization curves of the as-prepared MnO2 nanowires under 1,000 Oe, from which it can be seen that the observed Néel magnetic transition temperature (T N) is 99 K which is slightly higher than the reported value for 1D nanostructured MnO2 [32] which may be due to the small crystal size and the effect of surface spins. The FC curve shows a larger increase of the magnetization than the ZFC curve. This may be due to the large surface-to-volume ratio of the as-obtained product, which leads to a much larger proportion of noncompensated surface spins on the antiferromagnetic core and thus reveals the difference between the ZFC curve and FC curve. Figure 4b shows magnetization (M) as a function of applied magnetic field (H) of the nanowires at room temperature, which reveals that a superparamagnetic state exists at this temperature, as the coercivity (H c) and remnant magnetization are zero. A domain transformation mechanism occurs from multidomain to single domain when the size of magnetic particles decreases and if the single-domain particles become small enough, the magnetic moment in the domain fluctuates in direction due to thermal agitation, which leads to superparamagnetism [10]. For most magnetic materials, the single-domain particles lie in the range of 10–100 nm [33] which indicates that our synthesized MnO2 particles fall apparently in single domain due to which they exhibit superparamagnetism. The thermal activation energy overcomes cohesive energy of fluctuating magnetic domains which provides sufficient energy for the alignment of particle moments in an applied magnetic field resulting in the suppression of the hysteric behavior in the as-prepared MnO2 nanowires exhibiting superparamagnetism above the Neel temperature T N. The magnetic properties are strongly influenced by the shape of nanostructures because of the dominating role of crystallographic and shape anisotropy in magnetism. The shape of magnetic materials is unequivocally one of the key factors governing their magnetic behavior. Ying et al. [34] reported that the magnetization of elongated particles with a larger length-to-width ratio is more sensitive to the applied field which leads to the decreasing tendency of coercive field for elongated arrays. Hence, the extrinsic property of a magnetic material such as coercivity not only depends on the spin carrier but also on the size or shape of the magnets. The coercivity of MnO2 nanowires approaches zero which is considered to be due to the decrease in magnetic anisotropy. The total magnetic anisotropy of the system is K = K xtal + K s, where K xtal is the bulk magneto-crystalline anisotropy and K s represents the shape anisotropy [35]. The superparamagnetic behavior observed in the as-prepared MnO2 nanowires can be assigned to the decrease in shape anisotropy of a system, because the shape anisotropy in (elongated) wire-shaped morphology makes it easier to induce magnetization along the long direction of material. This is due to the fact that demagnetizing field is less in the long direction because the induced poles at the surface of material are further apart. At 55 K, which is below the Néel temperature, the M–H curve shows no hysteresis, indicating the antiferromagnetic nature of MnO2 nanowires as shown in Fig. 4c. The magnetic properties of manganese dioxide are determined by interactions between the Mn ions, which in turn depend on the Mn valence distribution in the [Mn2] O4 network [36]. It is also to note that the K+ cations are possibly introduced to the tunnel structure of MnO2, which may have influence on the magnetic properties [37–39]. There have been reports on the paramagnetic characteristics at room temperature and a ferromagnetic behavior of MnO2 nanowires below the Neel transition temperature [16, 24]. The ferromagnetic behavior of MnO2 nanowires at room temperature has also been reported [19]. In our previous work, we have reported the weak-ferromagnetic behavior of MnO2 nanorods at room temperature [32]. This conspicuous magnetic behavior of the as-prepared MnO2 nanowires shows the promise of the as-obtained product to be used in magnetic resonance imaging (MRI) and biomedical applications.
4 Conclusion
In summary, 1D single-crystalline tetragonal MnO2 nanowires with smooth and uniform surface morphology were successfully synthesized by a facile hydrothermal procedure. The nanowires have large surface-to-volume ratio because of small diameters (15–20 nm). The PL spectra of MnO2 nanowires exhibit a strong UV excitonic emission band at 380 nm, blue emission band at 452 nm and a weak defect-related green emission band at 542 nm. The PL studies and a moderate band gap value (2.5 eV) calculated from UV–visible absorption spectroscopy make this material potentially interesting for application in optoelectronic UV and visible emission devices and solar cells. The magnetic measurements indicate that the antiferromagnetic transition temperature (T N) is about 99 K.
References
Y. Li, J. Wang, Y. Zhang, M.N. Banis, J. Liu, D. Geng, X. Sun, J. Colloid Interface Sci. 369, 123–128 (2012)
G. Zou, H. Li, Y. Zhang, K. Xiong, Y. Qian, Nanotechnology 17, 313 (2006)
S. Jana, S. Pande, A.K. Sinha, S. Sarkar, M. Pradhan, M. Basu, T. Pal, J. Phys. Chem. C 113, 1386–1392 (2009)
S.C. Pang, S.F. Chin, C.Y. Ling, J. Nano Mater. 2, 607870 (2012)
Z. Pan, Li Xinyong, Z. Qidong, Li Shaomin, Nanoscale Res. Lett. 6, 323 (2011)
A.M. Toufiq, F.P. Wang, Q.U.A. Javed, J. Nanosci. Nanotechnol. 13, 2948 (2013)
B.A. Pinaud, Z. Chen, D.N. Abram, T.F. Jaramillo, J. Phys. Chem. C 115(23), 11830–11838 (2011)
J.E. Greedan, N.P. Raju, A.S. Wills, C. Morin, S.M. Shaw, J.N. Reimers, Chem. Mater. 10(10), 3058–3067 (1998)
H.J. Kim, J.B. Lee, Y.M. Kim, M.H. Jung, Z. Jaglicic, P. Umek, J. Dolinsek, Nanoscale Res. Lett. 2(2), 81–86 (2007)
S. Jana, S. Basu, S. Pande, S.K. Ghosh, T. Pal, J. Phys. Chem. C 111, 16272–16277 (2007)
C.M. Julien, M. Massot, C. Poinsignon, Spectrochimica Acta, Part A 60, 689–700 (2004)
F. Cheng, J. Zhao, W. Song, C. Li, H. Ma, J. Chen, P. Shen, Inorg. Chem. 45, 2038–2044 (2006)
Y. Yang, L. Xiao, Y. Zhao, F. Wang, Int. J. Electrochem. Sci. 3, 67–74 (2008)
X. Wang, Y. Li, J. Am. Chem. Soc. 124, 2880–2881 (2002)
X. Wang, Y. Li, Chem. Eur. J. 9, 300–306 (2003)
J.B. Yang, X.D. Zhou, W.J. James, S.K. Malik, C.S. Wang, Appl. Phys. Lett. 85, 3160–3162 (2004)
B. Li, G. Rong, Y. Xie, L. Huang, C. Feng, Inorg. Chem. 45, 6404–6410 (2006)
Y. Chen, C. Liu, F. Li, H.M. Cheng, J. Alloy Compd. 397, 282–285 (2005)
G.H. Yue, P.X. Yan, D. Yan, D.M. Qu, X.Y. Fan, M.X. Wang, H.T. Shang, J. Cryst. Growth 294, 385–388 (2006)
J.T. Sampanthar, J. Dou, G.G. Joo, E. Widjaja, L.Q.H. Eunice, Nanotechnology 18, 025601 (2007)
H. Wang, Z. Lu, D. Qian, Y. Li, W. Zhang, Nanotechnology 18, 115616 (2007)
X. Chen, X. Li, Y. Jiang, C. Shi, X. Li, Solid State Commun. 136, 94–96 (2005)
J. Luo, H.T. Zhu, H.M. Fan, J.K. Liang, H.L. Shi, G.H. Rao, Z.X. Shen, J. Phys. Chem. C 112, 12594–12598 (2008)
J.G. Zhao, J.Z. Yin, S.G. Yang, Mater. Res. Bull. 47, 896–900 (2012)
M. Zhou, X. Zhang, J. Wei, S. Zhao, L. Wang, B. Feng, J. Phys. Chem. C 115, 1398–1402 (2010)
Y.H. Pai, C.T. Tsai, Int. J. Hydrogen Energy 38, 4342–4350 (2013)
L. Song, S. Zhang, X. Wu, Q. Wei, Chem. Eng. J. 187, 385–390 (2012)
R. Kannan, K. Karunakaran, S. Vasanthkumar, Appl. Nanosci. 1, 197–203 (2011)
S. Li, Z. Ma, L. Wang, J. Liu, Sci. China, Ser. B: Chem. 51(2), 179–185 (2008)
P. Asogwa, J. Optoelectron. Biomed. Mater. 2, 109–117 (2010)
K.A.M. Ahmed, H. Peng, K. Wu, K. Huang, Chem. Eng. J. 172, 531–539 (2011)
A.M. Toufiq, F.P. Wang, Q.U.A. Javed, Y. Li, Nanotechnology 24, 415703 (2013)
N.A. Frey, S. Peng, K. Cheng, S. Sun, Chem. Soc. Rev. 38(9), 2532–2542 (2009)
L. Ying, L. Yongfeng, W. Tianxing, J. Nanomater. 1-6 (2012)
S. Hao-Ling, S. Hongtao, Z. Fei, Q. Limin, G. Song, Chem. Commun. 34, 4339–4341 (2005)
X.M. Liu, S.Y. Fu, C. Huang, J. Powder Technol. 154(2), 120–124 (2005)
H.T. Zhu, J. Luo, H.X. Yang, J.K. Liang, G.H. Rao, J.B. Li, Z.M. Du, J. Phys. Chem. C 112, 17089–17094 (2008)
J. Luo, H.T. Zhu, F. Zhang, J.K. Liang, G.H. Rao, J.B. Li, Z.M. Du, J. Appl. Phys. 105, 093925 (2009)
J. Luo, H.T. Zhu, J.K. Liang, G.H. Rao, J.B. Li, Z.M. Du, J. Phys. Chem. C 114, 8782–8786 (2010)
Acknowledgments
We appreciate the financial supports of National Key Scientific Instruments and Equipment Development Special Fund (2011YQ14014506 and 2011YQ14014507), the Oriented Award Foundation for Science and Technological Innovation, Inner Mongolia Autonomous Region, China (2012), University of Science and Technology Beijing (fundamental development fund and Chinese government scholarship program) and the Fundamental Research Funds for the Central Universities: FRF-BR-09-007A.
Author information
Authors and Affiliations
Corresponding author
Rights and permissions
About this article
Cite this article
Toufiq, A.M., Wang, F., Javed, Qua. et al. Hydrothermal synthesis of MnO2 nanowires: structural characterizations, optical and magnetic properties. Appl. Phys. A 116, 1127–1132 (2014). https://doi.org/10.1007/s00339-013-8195-0
Received:
Accepted:
Published:
Issue Date:
DOI: https://doi.org/10.1007/s00339-013-8195-0