Abstract
There is genetic variation in the female mating rate in the green-veined white butterfly (Pieris napi), and females benefit from male ejaculates that contain both sperm and accessory gland substances. Although polyandry corresponds to higher lifetime fecundity than monandry, some females abstain from remating irrespective of the number of available mates. Explaining genetic variation in mating rates requires that monandrous females perform better than polyandrous ones under some conditions. We experimentally explored the reproductive performance of females either with a low or high intrinsic mating rate by allowing them to mate, feed, and lay eggs freely in a laboratory. Individual females followed different life histories: during the early days of reproduction, females with a low mating rate produced more eggs than females with a high mating rate. Hence, refraining from the benefits of multiple mating may be beneficial, if the time for reproduction is limited, or other female traits associated with polyandry are traded off against longevity. Given the day length of 10 h, a model shows that even if polyandrous females enjoy higher lifetime reproductive success, changeable and unpredictable weather will favor monandry if each period of suitable weather lasts, on average, less than 5 days. Thus, a combination of life history cost and unpredictability of fitness may explain the maintenance of monandry in the wild. Our results are also consistent with the observation that frequency of monandry increases with latitude.
Similar content being viewed by others
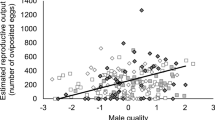
Avoid common mistakes on your manuscript.
Introduction
The traditional belief of monogamy as a prevailing female mating tactic in nature has undergone a prominent shift. Females may mate more than once, for example to avoid male harassment, even if mating is costly (Arnqvist 1989). However, in the meta-analysis of 122 experimental studies across ten insect orders, Arnqvist and Nilsson (2000) reported nearly ubiquitous positive effects of multiple mating on female reproduction. Females may mate more than once to ensure high fitness for their offspring by selecting good or compatible genes (Brown 1997; Jennions and Petrie 2000; Tregenza and Wedell 1998; Zeh and Zeh 1997) or to replenish their sperm storages (Gromko et al. 1984). Also other aspects of mating besides sperm transfer may enhance the female’s egg production (Opp and Prokopy 1986). In some insects, males provide females with large ejaculates that contain both sperm and nutritious accessory gland substances referred to as nuptial gifts. In these cases, it is particularly easy to understand why multiple mating bring benefits to the female (e.g., Boggs and Gilbert 1979; Boggs and Watt 1981; Kaitala and Wiklund 1994; Karlsson 1998; Oberhauser 1989; Reinhardt et al. 1999; Stjernholm and Karlsson 2000).
In butterflies, females in the genera Colias (Boggs and Gilbert 1979), Papilio (Watanabe 1988), and Pieris (Wiklund et al. 1993) are known to benefit from male derived substances. The green-veined white butterfly (Pieris napi) is a polyandrous species, in which male derived substances are partly incorporated into eggs (Wiklund et al. 1993). The positive effects of polyandry are dose-dependent, so that females receiving large spermatophores by mating multiply with recently unmated males, have higher lifetime fecundity, are able to maintain high egg weight for longer durations, and have a longer life span, compared to females that mate only once (Bergström and Wiklund 2002; Kaitala and Wiklund 1994; Karlsson 1998; Wiklund et al. 1993, 1998). In their influential study, Wedell et al. (2002) proved that female mating frequency and fecundity are under genetic control and show high heritability. Since male-derived nutrients enhance egg production and because not all studies have found covariation between female size and lifetime fecundity (e.g., Wiklund and Kaitala 1995), it seems that female egg production is largely determined by genetic variation in mating frequency and fecundity independent of female size variation in P. napi.
In good agreement with the idea of direct benefits of multiple mating, most females of P. napi are polyandrous and they may mate up to five times in the wild (Bergström et al. 2002; Wiklund and Forsberg 1991). Nevertheless, 12% of females mate only once and another 35–40% mate twice (Bergström et al. 2002). Moreover, the proportion of monandrous females varies among populations from 22 up to 35% in northern Scandinavia (Välimäki and Kaitala 2006). The high proportion of singly mated females in the north is not simply due to some females lacking a chance to mate again, since we were not able to reveal differences in mating frequency between wild-caught females and laboratory females that were introduced to an abundance of males. Traits that have direct effects on reproductive success are predicted to show only a minor additive genetic variance (Falconer 1989). Thus, the maintenance of low mating rates in the wild is a puzzle.
Generally, female lifetime reproductive output depends on fertility and life span. Females of some insect species suffer from reduced life span due to the cost of mating, including, for example, toxic properties of male ejaculates (Chapman et al. 1995; Kemp and Rutowski 2004) or an increased risk of physical injury (Crudgington and Siva-Jothy 2000). Since reduced longevity implies a shorter egg-laying period, these costs of mating could result in a reproductive depression of polyandrous females, and thus explain the maintenance of genetic variation in the female mating rate. However, these explanations do not apply to P. napi, because the female life span is actually reduced if they cannot realize their intrinsic mating frequency (Wedell et al. 2002). Thus, the benefits of polyandry may be lost if mating opportunities are scarce (Kokko and Mappes 2005) or if males can suppress the female mating rate below their optimum (Sauter et al. 2001). This does not seem likely in P. napi either, both because the males’ ability to control female mating rate is evidently low (Bergström and Wiklund 2005), and the operational sex ratio is highly male-biased (Wiklund et al. 1998).
Genetic variation in the female mating frequency and the surprisingly high prevalence of monandry may be explained if polyandry bears some features that result in diminishing rewards of multiple mating under some conditions that occur in the wild. Variation in the temporal distribution of the reproductive effort among females, mediated by mating tactics, can be such a factor, especially given unpredictable weather conditions that cause annual variation in the effective life span of butterflies. First, we explored temporal variation in the reproductive effort between females with varying mating tactics. We used both wild females and females that derived from artificially selected low and high mating rate lines. Secondly, we related the reproductive success of selected females to a model that predicts fitness in changing weather conditions.
Materials and methods
Selection procedure
Selection lines that allow comparison of life history traits between mating tactics were created in the University of Stockholm (Bergström 2004). Both lines derived from 51 wild-caught P. napi females that were netted from three populations—one in southern Sweden and two in the vicinity of Stockholm. Five eggs laid by each female were chosen to create the founder population for the selection lines. Larvae were reared on fresh leaves of Alliaria petiolata in a laboratory, in common circumstances, and only middle-sized butterflies were included in the founder population to avoid uncontrollable maternal effects and to minimize accidental selection on female size.
After a sufficient number of butterflies had hatched, 57 females and 76 males were divided evenly into three flight cages (0.8×0.8×0.5 m3) and were provided with adult food resources and leaves of A. petiolata for egg-laying. Females were allowed to mate freely for their whole life span, and all matings were recorded by inspecting the cages every 15 min. Mating couples were transferred into a cage of their own and mated females were allowed to lay eggs for 2 days after copula termination. Females were then released again into a randomly selected flight cage with males, and their mating rate was recorded during the following 10 days. Copulation with a virgin male lasts on average for ca. 2 h, and it is prolonged if a male has recently mated (Wiklund et al. 1998). Females that have not remated after 10 days usually do not remate at all (Wiklund et al. 1993). Hence, it is unlikely that any matings could have been left undetected during the selection procedure.
After 10 days, females were ranked by their mating rate, and the eggs laid by eight females that remated first and last (or did not remate) were included in founder populations of high (HMR) and low (LMR) mating rate lines, respectively. Ten offspring per female were reared under similar conditions. After eclosion, the selection procedure was repeated, but now butterflies from different selection lines were held in separate cages all the time and only the eight fastest HMR females to remate were selected to continue the HMR line, while the eight slowest LMR females were selected to continue the LMR line. After four generations of artificial selection, HMR and LMR lines were found to differ significantly in the female mating rate (Bergström and Wiklund, unpublished).
Mating experiment I
To study temporal variation in the reproductive effort with respect to mating tactics, we let females with varying mating rates lay eggs in a laboratory. Experimental animals were reared in a laboratory on A. petiolata. On the day of eclosion, butterflies were marked individually on the forewing with a marker pen and then kept in a refrigerator room (6°C) until a sufficient number of individuals had hatched. At first, we released 12 females from the LMR and HMR lines into two separate flight cages (0.65×0.65×0.65 m3) with twice as many randomly selected virgin males. Mating couples were transferred into a cage of their own.
Altogether, 24 cages with either one LMR or HMR female and at least two males (range: two to three) were placed in a laboratory with large windows so that the butterflies were exposed to quantitatively natural sunlight during daytime. The cages were provided with fresh Arabis alpina and Erysimum strictum shoots for egg-laying and flowering Viola sp. shoots with 20% sugar solution drops added twice a day on the petals for adult feeding. Butterflies faced a light regime of 10:14 h and a temperature regime of 30:20°C (light:dark). We inspected the cages every 20 min to detect all matings.
To avoid systematic effects on female performance that might arise from the exact location of the egg-laying cage, females were placed in the rows of cages so that an HMR female was always put in a cage next to an LMR female and vice versa. Females were constantly accompanied by at least two males to avoid reproductive depression due to suboptimal mating rate. Two males per female should be enough since males can mate at a far higher rate than females (Wiklund et al. 1998), and females are incapable of evaluating male quality before mating (Kaitala and Wiklund 1994). Any biases on the mating or egg production rate between HMR and LMR females caused by male origin or size were minimized by dividing males randomly among the cages. To ensure that males could deliver a full-sized spermatophore every time they mated, we replaced each male immediately after copulation or alternatively every third day if mating had not been detected by then. We used either virgin males or males that had not mated during three preceding days. The use of mated males is justifiable, because a male that had not mated for 2–3 days can deliver a new spermatophore that is as large as the first one (Bissoondath and Wiklund 1996; Svärd and Wiklund 1989).
Under these circumstances females were allowed to mate, feed, and lay eggs freely for 2 weeks. Every evening, host plants were checked for eggs and these were counted and removed. Finally, we determined the mating frequency of each female by dissecting the abdomen and counting the number of spermatophore (one is delivered at each mating) residues that remain within the female’s bursa copulatrix throughout her lifetime (see Drummond 1984).
Mating experiment II
Due to conceptual weaknesses of the selection procedure (see “Methodological aspects”), temporal variation in the reproductive effort between females with varying mating rates was also studied with wild butterflies. We used females that derived from 47 wild-caught females, which were netted from the northernmost part of Scandinavia, Kilpisjärvi (69°03′N: 20°50′E), because the probability of obtaining monandrous females into a random sample appears to increase with latitude. Larvae were reared in a laboratory on their natural host, A. alpina. On the day of eclosion, 30 randomly selected females were divided evenly into three flight cages (0.65×0.65×0.65 m3) and were provided with twice as many virgin males. Of these females, 21 mated for the first time on the same day and were selected for the experiment and placed into a cage of their own. The second experiment was otherwise conducted like the first one, but the females were offered Rorippa palustris shoots for egg laying and flowering Leontodon autumnalis shoots (plus sugar solution) for adult feeding, and they faced a light regime of 8:16 h and a temperature regime of 20:15°C (light:dark).
Data analysis
To test if there is temporal variation in the reproductive effort between females with high and low mating rates, we used a bootstrap technique (see Sokal and Rohlf 1995). We resampled the observed cumulative number of eggs laid by LMR and HMR females each day with replacement using 1,000 iterations. Resampling was carried out with a Resampling stats add-in for Microsoft Excel™ and was conducted separately for artificially selected (mating experiment I) and wild (mating experiment II) females. The sample size for both artificially selected LMR and HMR females was 12. With wild butterflies, to obtain a mating frequency variation of similar magnitude between LMR and HMR females as between the artificially selected lines, the six slowest females to remate and nine females that mated three or four times were included in the LMR and HMR groups, respectively. One female assigned into the LMR group died accidentally on the sixth day after the first mating, and was thus excluded from the fecundity analyses from that day on.
Given that the mean of estimated statistics from bootstrapped samples approximates the mean of the population, as if we had repeatedly sampled from the population without replacement, the method permits us to calculate 95% confidence intervals for the cumulative number of eggs for each day after the first mating. If the bootstrapped 95% CI of certain females does not overlap with the observed average of another female group, the difference in realized fecundity between HMR and LMR females will be statistically significant (α=0.05). We also tested variation in the average lifetime fecundity and mating frequency between the LMR and HMR groups with t tests.
In further analyses, we used only wild females (N=21). First, we explored temporal variation in reproductive effort between monandrous [mating frequency=1.16±0.17 (SE); range=1–2; N=6] and polyandrous [mating frequency=3.27±0.27 (SE);range=2–5; N=15) females by comparing the timing when an average offspring was produced using a t test. One female was considered monandrous although she mated twice, since the second mating did not take place until 8 days after the first and only three days before she died. We also explored the effects of the mating tactic and the mating act on the female egg-laying rate, measured as daily fecundity on the days when most of the polyandrous females remated (2, 4, and 6 days after the first mating). Females were divided into three groups based on their behavior those days. The first group consisted of monandrous females, while the second and the third groups included polyandrous females that mated or did not mate on that particular day, respectively.
Differences in average daily fecundity among groups were explored with one-way ANOVA and pairwise comparisons with LSD a posteriori tests. Each group of females consists of some repeated measures on a single individual; thus, days are not independent of each other. The widely used Bonferroni procedure would have reduced statistical power to the point where the risk of a type II error increased to unacceptable levels (since there will be temporal autocorrelation between egg numbers on consecutive days, the Bonferroni assumption of independent tests is incorrect; see Nakagawa 2004). Thus, instead of using the Bonferroni procedure to control the risk of type I errors, we controlled the proportion of false positives at the α=0.05 level among all significant tests, i.e., false discovery rate (FDR; see Benjamini and Hochberg 1995; García 2004). In practice, null hypotheses can be safely rejected if k×(α/n)≥p k,, where k is the ascending rank order of p k and n is the number of tests.
T tests and ANOVA were conducted with SPSS for Windows 12.0.1 statistical software. Tested variables were log-transformed if they deviated from normal distribution according to Kolmogorov–Smirnov’s tests. Equalities of variances were tested with Levene’s tests.
Methodological aspects
Given that females with a low mating rate are scarce in natural populations and they cannot be identified by their external appearance, it is very hard to obtain wild LMR females in sufficient numbers for statistical analyses. Thus, we decided to use artificially selected butterfly lines in the first experiment. Because selection lines were not replicated, we agree that some caution toward our results is well-grounded. The obvious problem with the selection procedure is that original females were netted from different locations and the number of females that gave rise to LMR and HMR lines was quite low. Thus, the results of the first experiment may, to some extent, be affected by geographic effects and/or genetic drift. However, the low number of generations involved in the selection procedure and the concordance of results between the first and the second experiment conducted with wild butterflies implies that variation in egg-laying patterns between selected LMR and HMR lines resembles natural variation as well. Similarly, the possible confounding effects that might have risen from uncontrollable variation in male origin in the first experiment can be considered negligible. Thus, conceptual weaknesses are unlikely to compromise our conclusions.
Determination of suitable weather conditions for butterfly activity
The effects of weather conditions on lepidopteran activity in Kilpisjärvi were determined by linking behavioral data on diurnal macrolepidopteran (DML; families Hesperiidae–Noctuidae) species with weather data provided by the Finnish Meteorological Institute. Since we are basically dealing with butterflies that are active only in daytime and obligatorily dependent on sunshine, the estimation of the length of suitable weather conditions was restricted to a period of 9:00 a.m.–7:00 p.m. with no rain.
In addition to rainfall, weather data included average values of ambient temperature and wind speed, which were measured hourly. We used the number of DML individuals observed during a certain period on a standardized area as an estimation of butterfly activity. In practice, three observers spent 20 min on an area of 50×50 m and calculated all DMLs that were identifiable. Activity measurements were done on five squares daily and repeated five times on each square in days with slightly different weather conditions in 2003. According to the regression analysis, the variation in the number of observed DMLs with respect to ambient temperature fits best to the logistic equation \(y = 150.11\ln x - 350.49\) (R 2=0.704; df=23; F=54.8; p<0.001), which indicates that butterfly activity decreases rapidly when the temperature goes below 12°C. Average wind speed on those same days was 3.6 m/s with the upper limit of 95% confidence intervals being 4.6 m/s. This was rounded up to the nearest integer to set the upper level of permissible wind speed. Although all aspects of butterfly thermoregulation were not taken into account, the given frames fit well into our own observations of butterfly activity.
Results
The lifetime number of matings by females from the artificially selected LMR line (1.17±0.11, SE) was lower than that of the females from the HMR line (3.00±0.17, SE; t=−8.848; df=22; p<0.001). HMR females remated for the first and second time 3.08±0.08 (N=12; Mo=3) and 5.18±0.12 (N=10; Mo=5) days after the first mating, respectively. Wild females that we used to compare the cumulative number of eggs between mating tactics assigned into the HMR group (3.44±0.18) had a higher mating frequency than the wild LMR females [1.16±0.17 (t=−1.406; df=12.58; p=0.000]. Those HMR females remated for the first, second, and third time 3.00±0.44 (N=9; Mo=2), 5.38±0.92 (N=8; Mo=4), and 7.25±1.60 (N=4; Mo=6) days after the first mating, respectively. The incoherence in the number of observations between the first two remating days is due to one unnoticed remating.
During the first 5 days after the first mating, artificially selected LMR females produced more eggs than HMR females (Fig. 1). Since the result is practically identical if we resample LMR females, only 95% CI for HMR females is shown. A similar pattern with respect to the female mating rate was also observed with wild butterflies (Fig. 2). Although LMR females performed better during the early days, lifetime fecundity of the HMR females in the consecutive experiments was 1.4 and 1.6 times higher than that of LMR females, respectively (t=−2. 206, df=15.893, p=0.041; t=−4.489, df=12, p=0.001; Figs. 1 and 2).
The difference in the timing of egg production is also seen when comparing the time (in days) from the first mating until egg-laying, averaged over all eggs. This averages 4.10±0.11 days for monandrous butterflies and 7.36±0.27 days for polyandrous ones (t=−9.042; df=19; p<0.001). Average daily fecundity varied between female groups on the days when most of the polyandrous females remated, even after FDR was controlled for (Table 1). Mating seemed to decrease the reproduction rate of P. napi females, as indicated by the low number of eggs laid by polyandrous females on the days of remating compared to polyandrous females that refused to mate on those particular days. A similar pattern was also observed between monandrous and mating polyandrous females on the second and fourth day after the first mating. However, that difference diminished later on. The reproductive rate of monandrous and nonmating polyandrous females was equal until the sixth day after the first mating, when the daily fecundity of polyandrous females evidently exceeded that of monandrous ones.
In P. napi, discrete populations face widely varying weather conditions. Sunny weather, which is obligatory to butterfly reproduction, is frequently interrupted by cold and rainy periods in northern Scandinavia. By making the reasonable assumption that the date of a butterfly female’s first mating falls within a suitable weather period, we find out that her realized fitness will depend on the number of suitable days afterwards. From a butterfly perspective, the number of such days is highly unpredictable. Assuming that each day carries with it a constant risk (probability p) that the weather turns unsuitable, the fitness W of a female, whose mating tactic leads it to lay a cumulative sum of F(i) eggs by the end of the ith day, equals
When the previous equation is applied to the data of the first experiment, we can assume that F(i) stays constant (no more eggs) after day 13, which means that the Eq. 1 can now be written as
A given value of p corresponds to a mean of
suitable consecutive days for reproductive activities. The average success of LMR and HMR females depends on the mean length of each suitable weather period, such that LMR females have higher expected reproductive success if effective life spans are, on average, short (Fig. 3). Given the day length of 10 h, the predicted fitness of the two lineages is equal if the effective life spans of females are, on average, 5 days.
Expected reproductive success W as a function of the mean length of the flight window (i.e., effective life span) Eq. 3 for LMR and HMR females, and assuming unpredictable weather (a constant probability of weather change, regardless of the number of suitable days experienced so far)
The threshold length of the effective life span depends on how accurately the samples replicate true reproductive success: it shifts between 3 and 4 days if we use the lower limit of the 95% confidence interval for reproductive success, and 9 days if we use the higher limit (not shown; fitness curves qualitatively similar to Fig. 3). However, we stress that it is highly unlikely (0.05×0.05=0.0025) that estimates of both LMR and HMR females’ reproductive success are at the boundaries of their confidence intervals. Thus, our result is quite robust.
Discussion
P. napi females benefit from male-derived, nutrient-rich ejaculates in terms of increased longevity and/or lifetime fecundity (Bergström and Wiklund 2002; Stjernholm and Karlsson 2000; Wiklund et al. 1993; 1998). Hence, it is not surprising that P. napi females are generally polyandrous. Yet, our study shows that, even if there is no doubt that a polyandrous lifestyle corresponds to higher lifetime fecundity, high mating rates can be associated with a cost, which may explain the maintenance of variation in female mating frequency.
Surprisingly, both artificially selected and wild P. napi females with a low mating rate produced more eggs during the early days of reproduction than comparable females with a high mating rate. Therefore, foregoing the chance of obtaining nutrients through multiple mating may be beneficial for a female in terms of egg quantity, if the time for reproduction is limited or other female traits associated with a high degree of polyandry are traded off against longevity. The benefits of multiple mating arose about 1 week after the first mating, by which time the reproductive rate of females with a high mating rate had exceeded that of females with a low mating rate. Consequently, females with a high mating rate had ca. 1.5 times higher lifetime fecundity than females with low mating rate. A notable discrepancy in lifetime fecundity between this and some earlier studies (Bergström and Wiklund 2002; Stjernholm and Karlsson 2000; Wiklund et al. 1993, 1998) is most probably due to high temperature and long-lasting dark periods during our experiments, which enforced females to allocate their body reserves for somatic maintenance at the expense of reproduction. Yet, LMR and HMR females faced similar conditions, such that the relative difference in lifetime fecundity with respect to mating tactic is in concordance with the prevailing conception (reviewed by Arnqvist and Nilsson 2000).
In general, mating is time-consuming and mutually exclusive with egg laying (see Daly 1978). The low daily fecundity of polyandrous females that mated compared to that of nonmating polyandrous females indicates that the time cost of mating is biologically significant in P. napi. Given that monandrous females also laid more eggs than mating polyandrous females early in the reproductive period, this time cost is likely to constitute a great deal of observed temporal variation in realized fecundity between LMR and HMR females. Similarly, the offspring of polyandrous females will be laid, on average, later than the offspring of monandrous ones. As a consequence, the larvae of polyandrous females have less time for development than the larvae that derive from monandrous females. An additional cost related to polyandry may arise from the females’ need to mate according to their intrinsic mating rate, in order to realize their potential fecundity (see Wedell et al. 2002). However, our results do not support this scenario, since there was no difference in daily fecundity during the early days of reproduction between monandrous and nonmating polyandrous females. Here, our indirect approach is not totally conclusive; a more direct evaluation of the time costs would have involved a comparison of the number of eggs laid by a given female on the day of remating with her hypothetical daily fecundity if she had not mated on that day, which unfortunately is impossible.
P. napi females, which mate with a virgin male, have to remain in copula for an average of ca. 2 h, while copulation with a recently mated male may be prolonged up to 7 h of suitable weather (Wiklund et al. 1998). Assuming relatively short effective life spans for butterflies (Chew 1981), prolonged copulation points to the obvious time costs of remating. Since P. napi females are unable to discriminate between mated and unmated males (Kaitala and Wiklund 1995) and virgin males are no longer readily available late in the season, we may have underestimated the cost of remating for wild females. The cost of mating may also include post-mating processes such as spermatophore absorption and sperm migration to the spermatheca (see Tschudi-Rein and Benz 1990).
Potential costs of remating have previously been considered negligible in P. napi (Wiklund et al. 1993). The heritability of mating rates was not clear at that time; thus, the categorization of females into monandrous and polyandrous ones beforehand was impossible. Consequently, most of the randomly selected females considered monandrous were actually polyandrous, but were forced to mate only once (Wiklund et al. 1993). Hence, the reproductive performance of true monandrous and polyandrous females could not be explored properly in Wiklund et al. (1993) study. In spite of the conceptual problem, their experiment suggests that the observed cost of polyandry is not due to a trade off between egg quality and early fecundity because, otherwise, the first eggs laid by monandrous and polyandrous females assigned to monandry treatment should have been slightly lighter than eggs laid by true polyandrous females alone. Yet, we have to stress that we did not explicitly take the possible variation in either female mass or egg mass into account, both of which could possibly contribute to offspring quality.
In the wild, there is a risk of early grave and unfavorable weather conditions that are inappropriate for reproduction. Thus, female reproductive output is sometimes more dependent on early egg-laying rate than on hypothetical performance in ideal conditions. The model shows that if the effective life span of a female lasts less than 5 days, on average, at a time, monandry becomes the favored tactic. Interestingly, 5 days after the first mating LMR females still performed better in the experimental setting, yet the model predicts them equal fitness with HMR females. This highlights the importance of unpredictability: even if average flight periods are fairly short, weather patterns may stay stable for a number of days, allowing HMR females to reap their late-life benefits.
At least in the northern edge-or-range populations, individuals frequently face periods of weather that render reproduction impossible. In Kilpisjärvi, suitable weather conditions (9:00 a.m.–7:00 p.m.; temperature ≥12°;wind ≤5 m/s; no rain) lasted only for 46 h during the flight period of P. napi in 2000 (20th June–15th July). According to our model, the selection for polyandry should be relaxed in such conditions. In accordance, the proportion of monandrous females appears to increase along with latitude, being almost three times higher in northern than southern Finland (Välimäki and Kaitala 2006).
The prevalence of genetic variation in each population and the annual variation in mating frequencies among wild-caught females in Kilpisjärvi raise a possibility that the variation may be related to weather unpredictability: fitness, as described in Fig. 3, is accumulated through all possible lengths of suitable weather conditions and their associated probabilities. Although there are years when the selection pressure for polyandry is expected to be low, summer weather in Fennoscandia shows strong annual variation. As an indicator of this, the number of rainy days in July in Kilpisjärvi has varied between 4 and 25 in the last few decades (1952–2003), and the average July temperature has varied between 4.2 and 14.0°C during the same period. Moreover, the length of suitable weather between 20th June and 15th July during 2000–2004 has varied between 46 and 142 h. An annual variation in weather conditions creates obvious possibilities for a temporally fluctuating selection, although it is currently unclear if these fluctuations are indefinitely sufficient for maintaining variation in mating rates.
Temporal variation in the reproductive effort results in the asynchronous hatching of offspring, but at the same time the larvae of polyandrous females develop faster than the larvae of monandrous ones (Wedell et al. 2002). This could create a counter-selection pressure to the one we have described, if larvae were time-limited in the north. However, thermal summer has lasted during the last 5-year period, on average, for 48 days past the peak flight season, which is equivalent to time between spring and summer generations in southern Finland (Huldén et al. 2000). Hence, the life history change from bi- to univoltine is likely to relax selection for fast larval development in the north. On the other hand, high larval growth rate is coupled with poor performance under food stress (Gotthard et al. 1994) and an increased predation risk (Gotthard 2000) in another lepidopteran species. If this holds true in P. napi as well, additional costs of polyandry may arise from increased larval mortality. Hence, even if females have relatively long effective life spans, difference in female fitness between mating tactics may not be as pronounced as expected, based on the number of eggs laid only.
Either way, life history considerations will help understand and give rise to novel hypotheses that explain why genetic variation and seemingly suboptimal mating rates are maintained in butterflies and why monandry is more common in the northernmost parts of the distribution of P. napi. Our modeling exercise indicates that a low early egg-laying rate alone can, in principle, form a serious enough cost, which can outweigh the well-documented benefits of multiple mating under unpredictable and frequently changeable weather conditions.
References
Arnqvist G (1989) Multiple mating in a water strider: mutual benefits or intersexual conflict? Anim Behav 38:749–756
Arnqvist G, Nilsson T (2000) The evolution of polyandry: multiple mating and female fitness in insects. Anim Behav 60:145–164
Benjamini Y, Hochberg Y (1995) Controlling the false discovery rate: a practical and powerful approach to multiple testing. J R Stat Soc B 57:289–300
Bergström J (2004) The evolution of mating rates in Pieris napi. Dissertation, Department of Zoology, Stockholm University
Bergström J, Wiklund C (2002) Effects of size and nuptial gifts on butterfly reproduction: can females compensate for a smaller size through male-derived nutrients? Behav Ecol Sociobiol 52:296–302
Bergström J, Wiklund C (2005) No effect of male courtship intensity of female remating in the buttefly Pieris napi. J Insect Behav 18:479–489
Bergström J, Wiklund C, Kaitala A (2002) Natural variation in female mating rate in a polyandrous butterfly: effects of size and age. Anim Behav 64:49–54
Bissoondath CJ, Wiklund C (1996) Effect of male history and body size on ejaculate size and quality in two polyandrous butterflies, Pieris napi and Pieris rapae (Lepidoptera: Pieridae). Funct Ecol 10:457–464
Boggs CL, Gilbert LE (1979) Male contribution to egg production in butterflies: evidence for transfer of nutrients at mating. Science 206:83–84
Boggs CL, Watt WB (1981) Population structure of pierid butterflies. IV. Genetic and physiological investment in offspring by male Colias. Oecologia 50:320–324
Brown WD (1997) Female remating and the intensity of female choice in black-horned tree crickets, Oecanthus nigricornis. Behav Ecol 8:66–74
Chapman T, Liddle LF, Kalb JM, Wolfner MF, Partridge L (1995) Cost of mating in Drosophila melanogaster females is mediated by male accessory gland products. Nature 373:241–244
Chew FS (1981) Coexistence and local extinction in two pierid butterflies. Am Nat 118:655–672
Crudgington HS, Siva-Jothy MT (2000) Genital damage, kicking and early death: the battle of the sexes takes a sinister turn in the bean weevil. Nature 407:855–856
Daly M (1978) The cost of mating. Am Nat 112:771–774
Drummond BB (1984) Multiple mating and sperm competition in the Lepidoptera In: Smith RL (ed) Sperm competition and the evolution of animal mating systems. Academic, London, pp 547–572
Falconer DS (1989) Introduction to quantitative genetics. Longman, Harlow
García LV (2004) Escaping the Bonferroni iron claw in ecological studies. Oikos 105:657–663
Gotthard K (2000) Increased risk of predation as a cost of high growth rate: an experimental test in a butterfly. J Anim Ecol 69:896–902
Gotthard K, Nylin S, Wiklund C (1994) Adaptive variation in growth-rate—lifehistory costs and consequences in the speckled wood butterfly, Pararge aegeria. Oecologia 99:281–289
Gromko MH, Newport MEA, Kortier MG (1984) Sperm dependence of female receptivity to remating in Drosophila melanogaster. Evolution 38:1273–1282
Huldén L, Albrecht A, Itämies J, Malinen P, Wettenhovi J (eds) (2000) Atlas of Finnish Macrolepidoptera. Suomen Perhostutkijain Seura. Finnish Museum of Natural History, Helsinki
Jennions MD, Petrie M (2000) Why do females mate multiply? A review of the genetic benefits. Biol Rev 75:21–64
Kaitala A, Wiklund C (1994) Polyandrous female butterflies forage for matings. Behav Ecol Sociobiol 35:385–388
Kaitala A, Wiklund C (1995) Female mate choice and mating costs in the polyandrous butterfly Pieris napi. J Insect Behav 8:355–363
Karlsson B (1998) Nuptial gifts, resource budgets, and reproductive output in a polyandrous butterfly. Ecology 79:2931–2940
Kemp DJ, Rutowski RL (2004) A survival cost to mating in a polyandrous butterfly, Colias eurytheme. Oikos 105:65–70
Kokko H, Mappes J (2005) Sexual selection when fertilization is not guaranteed. Evolution 59:1876–1885
Nakagawa S (2004) A farewell to Bonferroni: the problems of low statistical power and publication bias. Behav Ecol 15:1044–1045
Oberhauser K (1989) Effects of spermatophores on male and female monarch butterfly reproductive success. Behav Ecol Sociobiol 25:237–246
Opp SB, Prokopy RJ (1986) Variation in laboratory oviposition by Rhagoletis pomonella (Diptera: Tephrididae) in relation to mating status. Ann Entomol Soc Am 79:705–710
Reinhardt K, Köhler G, Schumacher J (1999) Females of the grasshopper Chorthippus parallelus (Zett.) do not remate for fresh sperm. Proc R Soc Lond B 266:2003–2009
Sauter A, Brown MJF, Baer B, Schmid-Hempel P (2001) Males of social insects can prevent queens from multiple mating. Proc R Soc Lond B 268:1449–1454
Sokal RR, Rohlf FJ (1995) Biometry—The principles and practice of statistics in biological research. Freeman, New York
Stjernholm F, Karlsson B (2000) Nuptial gifts and the use of body resources for reproduction in the green-veined white butterfly Pieris napi. Proc R Soc Lond B 267:807–811
Svärd L, Wiklund C (1989) Mass and production rate of ejaculates in relation to monandry/polyandry in butterflies. Behav Ecol Sociobiol 24:395–402
Tregenza T, Wedell N (1998) Benefits of multiple mates in the cricket Gryllus bimaculatus. Evolution 52:1726–1730
Tschudi-Rein K, Benz G (1990) Mechanisms of sperm transfer in female Pieris brassicae (Lepidoptera: Pieridae). Ann Entomol Soc Am 83:1158–1164
Välimäki P, Kaitala A (2006) Does a lack of mating opportunities explain monandry in the green-veined white butterfly (Pieris napi)? Oikos (published online 18-May-2006, http://www.blackwell-synergy.com/doi/pdf/10.1111/j.2006.0030-1299.14947.x)
Watanabe M (1988) Multiple matings increase the fecundity of the yellow swallowtail butterfly, Papilio xuthus L., in summer generations. J Insect Behav 1:17–27
Wedell N, Wiklund C, Cook PA (2002) Monandry and polyandry as alternative lifestyles in a butterfly. Behav Ecol 13:450–455
Wiklund C, Forsberg J (1991) Sexual size dimorphism in relation to female polygamy and protandry in butterflies: a comparative study of Swedish Pieridae and Satyridae. Oikos 60:373–381
Wiklund C, Kaitala A (1995) Sexual selection for large male size in a polyandrous butterfly: the effect of body size on male versus female reproductive success in Pieris napi. Behav Ecol 6:6–13
Wiklund C, Kaitala A, Lindfors V, Abenius J (1993) Polyandry and its effect on female reproduction in the green-veined white butterfly (Pieris napi L.). Behav Ecol Sociobiol 33:25–33
Wiklund C, Kaitala A, Wedell N (1998) Decoupling of reproductive rates and parental expenditure in a polyandrous butterfly. Behav Ecol 9:20–25
Zeh JA, Zeh DW (1997) The evolution of polyandry. I. Intragenomic conflict and genetic and genetic incompatibility. Proc R Soc Lond B 263:1711–1717
Acknowledgements
We thank Christer Wiklund for providing artificially selected butterflies and for his valuable comments on the manuscript. We are also grateful to the anonymous referees for their contribution to the manuscript, and to Nora Weckström for linguistic advice. Sanni Jørgensen, Sami Kivelä, Tomi Mutanen, and Petri Hirvonen participated in larval rearing and fieldwork. This study was supported by the Academy of Finland (grant to HK) and Ella och Georg Ehrnroots stiftelse (grant to PV). Weather data was provided by the Finnish Meteorological Institute (IL drno: 1/410/05). Permission for butterfly sampling was granted by the Finnish Forest Research Institute. All Finland’s guidelines and legal requirements for the use of animals in research were followed.
Author information
Authors and Affiliations
Corresponding author
Additional information
Communicated by N. Wedell
Rights and permissions
About this article
Cite this article
Välimäki, P., Kaitala, A. & Kokko, H. Temporal patterns in reproduction may explain variationin mating frequencies in the green-veined white butterfly Pieris napi . Behav Ecol Sociobiol 61, 99–107 (2006). https://doi.org/10.1007/s00265-006-0240-y
Received:
Revised:
Accepted:
Published:
Issue Date:
DOI: https://doi.org/10.1007/s00265-006-0240-y