Abstract
Inter-individual variation in lifetime reproduction is the key target for natural selection, and it is influenced by many factors. Yet, we lack an understanding of how abiotic and biotic factors interact to influence lifetime reproductive output (number of offspring) and reproductive effort (total biomass invested into reproduction). Thus, we used a factorial design to manipulate variability in food availability and temperature while also accounting for mate quality. We tested hypotheses related to estimates of lifetime reproductive output and effort in females of the wing-dimorphic sand field cricket (Gryllus firmus). Environmental variability influenced a temporal tradeoff of reproduction because females experiencing fluctuating temperatures had a particular bias toward reproductive output during early adulthood. Also, complex environmental variability (i.e., multiple and co-varying environmental factors) influenced differential allocation, which is when individuals adjust their reproductive efforts according to mate quality. Females mated to higher quality males laid more eggs only in environments that were highly stable (constant temperature and ad libitum food access). Reproductive effort was affected by a food–temperature interaction—fluctuating temperatures promoted egg production when food was limited, while constant temperature promoted egg production when food was abundant. Although a wing dimorphism mediates a well-established flight–fecundity tradeoff during early adulthood in G. firmus, short- and long-winged morphs exhibited similar lifetime reproduction and responded similarly to complex environmental variability. Given the natural co-variation of many environmental factors (e.g., water limitation often accompanies heat waves), we encourage continued work examining the role of complex environmental variability in tradeoffs related to reproductive decision-making and allocation.
Significance statement
It is unclear how abiotic and biotic factors interact to influence lifetime reproductive output (number of offspring) and reproductive effort (total biomass invested into reproduction). Thus, we examined the effects of mate quality and complex environmental variability (variable food and temperature) on several metrics of lifetime reproduction. Female crickets mated to high-quality males had greater reproductive output, but only in highly stable or environmental conditions. Fluctuating temperatures biased reproductive output toward early adulthood, particularly in short-winged females. Reproductive effort was affected by a food–temperature interaction—fluctuating temperatures promoted egg production when food was limited, while constant temperature promoted egg production when food was abundant. Females can also retain unfertilized eggs, and egg retention (i.e., unrealized fitness potential) was influenced by a combination of mate quality and complex environments. Thus, it is important to carefully consider the interplay between biotic and abiotic factors in aspects of lifetime reproduction and reproductive decision-making.
Similar content being viewed by others
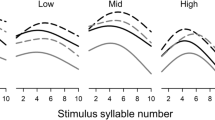
Avoid common mistakes on your manuscript.
Introduction
Reproduction is an essential component of fitness, and the dynamics of reproductive output (i.e., reproductive success, such as the number of eggs or offspring produced) form the foundation for life-history theory (Stearns 1992; Roff 2002; Flatt and Heyland 2011). However, reproductive effort obligates costs associated with time (e.g., time spent guarding nest or provisioning offspring), physiology (e.g., energy expenditure or growth of reproductive tissues at expense of somatic tissues), and ecology (e.g., increased predation risk) (van Noordwijk and de Jong 1986; Magnhagen 1991; Harshman and Zera 2007; Royle et al. 2012). Therefore, selection should favor individuals that mitigate reproductive costs by adaptively allocating limited resources toward reproduction over their lifetimes (Cody 1966; Williams 1966; Brommer 2000). For example, sub-optimal environments characterized by increased risk of mortality or reduced food availability can lead to increased reproductive investment during early adulthood reproduction at the expense of reproduction during late adulthood (sensu the “terminal investment hypothesis”: Clutton-Brock 1984; Lemaitre et al. 2015; reviewed in Duffield et al. 2017). Because they spend their lives in complex environments that change with time and space, animals must integrate multiple environmental inputs to optimize reproductive decisions throughout adulthood.
Sexual selection is based on the assumption that mate quality informs reproductive decisions (e.g., mate choice: Andersson 1994). Reproductive decision-making is linked to life-history strategy via the concept of differential allocation (DA), which predicts that an individual adaptively adjusts its reproductive effort according to the quality of its mate (reviewed in Haaland et al. 2017). For example, an individual with a low-quality mate may compensate by increasing its reproductive effort (i.e., reproductive compensation or negative DA: reviewed in Gowaty 2008; Harris and Uller 2009; Ratikainen and Kokko 2010). Alternatively, an individual with a high-quality mate may choose to capitalize by increasing its reproductive effort (i.e., positive DA: Burley 1986; Sheldon 2000). Thus, DA can result in either a negative or positive relationship between mate quality and reproductive effort. However, support for DA is mixed potentially because the role of DA may depend on context, such as age, body condition, or mating system (reviewed in Harris and Uller 2009; Ratikainen and Kokko 2010; Horvathova et al. 2012; Haaland et al. 2017). For example, maternal body size is positively associated with reproductive output across taxa (e.g., reviewed in Gotthard et al. 2007)—thus, female body size (or quality) may be an important context because larger females may be “choosier” or more sensitive to variation in male quality.
In addition to biotic factors, such as mate quality, abiotic environmental factors can exert strong effects on reproductive effort and output. For example, abundant food and ideal temperatures are often considered aspects of high-quality environments as they typically promote reproduction across taxa (Boggs and Ross 1993; Kirk 1997; Both and Visser 2005; Anderson et al. 2011; Kleinteich et al. 2015; reviewed in Angilletta 2009). However, environments exhibit spatiotemporal variation, such as the temperature gradient between shaded and sunny microhabitats, or the shift in food abundance over the course of a breeding season (e.g., Korpimaki and Wiehn 1998; Sears et al. 2016). Thus, in addition to mean environmental values (e.g., constant low temperature vs. constant high temperature: reviewed in Dillon et al. 2016), environmental quality may be determined via environmental variability, which can influence reproductive patterns. For example, fluctuations in a single environmental factor (temperature) during development can improve performance in a range of life-history traits (e.g., reproduction) in insects (Hervé et al. 2015). However, environments are complex and characterized by multiple, co-varying factors. Thus, it is important to understand how animal life histories respond to complex environmental change (Hastings and Caswell 1979; Zammuto and Millar 1985; Charmantier et al. 2008; Todgham and Stillman 2013; Kaunisto et al. 2016). Yet, to our knowledge, the role of complex environmental variability (e.g., combined manipulations of temperature variability and food availability) in animal life histories, generally, or in DA, specifically, is unexplored.
Further, life-history tradeoffs may influence reproductive decisions or strategies. Dispersal is an important aspect of many life histories because it allows individuals to move to higher quality environments (Roff 1994). Yet, investment into dispersal-related traits can obligate tradeoffs with other life-history traits (reviewed in Zera and Harshman 2001; Guerra 2011). Given these benefits and costs, dispersal polymorphisms are common among insects (reviewed in Zera and Denno 1997). For example, each discrete wing morph of Gryllus crickets adopts a different strategy to a flight–fecundity tradeoff. During early adulthood, long-winged (LW) females specialize in dispersal (invest in functional flight musculature) at a cost to reproduction while short-winged (SW) females lack the ability to fly in return for greater reproductive investment (heavier ovaries) (Zera et al. 1997; reviewed in Zera and Harshman 2001; Zera 2005). Investigations using both SW and LW crickets would allow for the examination of the effects of complex environmental variability and DA in animals with different life-history allocation strategies (e.g., individuals investing into flight muscles may respond differently than those that do not invest into flight muscles).
Thus, we examined several estimates of lifetime reproductive output (number of eggs oviposited) and effort (total biomass invested into reproduction) in females of the wing-dimorphic sand field cricket (Gryllus firmus). We accounted for female body size and mate (male) quality because increased reproductive output or effort is associated with larger females and higher male quality (i.e., positive DA) in Gryllus crickets (Carriere and Roff 1995; Bretman et al. 2006). We used a factorial design to manipulate variability in food availability (ad libitum vs. intermittent access) and temperature (constant vs. ecologically relevant fluctuations) in female G. firmus, each of which was mated to a single male of known quality. We used this design to test three hypotheses.
- (1)
Environmental variability influences a tradeoff between investment into early and late reproduction (Williams 1966; Brommer 2000). We predict that variable environmental conditions (e.g., intermittent food availability and/or fluctuating temperatures) will increase reproductive output during early adulthood relative to late adulthood based on other work reviewing the independent role of a single environmental factor in this dynamic. Specifically, resource limitation tends to favor investment into reproduction during early adulthood in many animals (Lemaitre et al. 2015).
- (2)
Environmental variability influences DA, the importance of an individual’s mate quality to its reproductive output or effort. Here, we test two opposing predictions. First, we predict that positive DA will be favored in stable (high-quality) environments. We expect variable environments to be perceived as lower quality (e.g., because animals in our study were adapted to constant temperature conditions: see below) and, thus, incentivize females to fertilize and oviposit as many eggs as possible (regardless of mate quality). That is, a terminal investment strategy (Clutton-Brock 1984; Lemaitre et al. 2015; reviewed in Duffield et al. 2017) may mask or eliminate DA in variable environments. Second, we alternatively predict that variable (low-quality) environments will favor positive DA. Sexual selection may be stronger in more stressful environmental conditions because it will eliminate mutations from a population in which adaptation is particularly critical (Lorch et al. 2003; Whitlock and Agrawal 2009; Long et al. 2012; Connallon and Hall 2016).
- (3)
A wing dimorphism influences lifetime reproduction. During early adulthood (i.e., < 1 week into adulthood), SW G. firmus females exhibit greater reproductive effort relative to flight-capable LW females because flight investment by LW females delays heavy investment into egg production prior to mating (reviewed in Zera and Harshman 2001; Zera 2005). However, the role of this wing dimorphism in lifetime reproductive dynamics is unclear. Given SW females’ head-start in egg production, we predict that SW females will produce and oviposit more eggs over their lifetimes relative to LW females.
Methods
Study animals
The wing dimorphic sand field cricket (Gryllus firmus) is endemic to the south and eastern USA, ranging from Connecticut to Texas (Capinera et al. 2004). Wing morphology is influenced by genetic and environmental factors, but artificial selection has created nearly true-breeding SW and LW lab populations (reviewed in Zera 2005; see below). Each discrete wing morph adopts a different strategy to a flight–fecundity tradeoff. During early adulthood, LW females specialize in dispersal by investing in functional flight musculature, which comes at a cost to reproduction. Short-winged females lack the ability to fly during early adulthood but have greater reproductive investment (ovaries that are 100–400% heavier) than LW females (reviewed in Zera and Harshman 2001; Zera 2005). Cricket stock originated from populations near Gainesville, FL that exhibit morph frequencies of approximately 0.4 SW and 0.6 LW (Zera et al. 2007). Given the putative fecundity advantage of SW females (reviewed in Zera 2005) and the greater attractiveness of SW males (Crnokrak and Roff 1995), it is difficult to rear mixed-morph populations that produce similar frequencies of SW and LW crickets. Therefore, crickets were raised in outbred populations (blocks) that were artificially selected for several decades to produce either SW or LW morphs that have been previously described (Zera 2005). Due to limited within-block genetic variation, three replicate blocks of crickets were used in this study. Both wing morphs were used in the study to address Hypothesis 3 (a wing dimorphism influences lifetime reproduction), and crickets were reared in standard conditions (16 h photoperiod, 28 ± 1 °C, and ad libitum access to water and commercial dry cat food) until adulthood.
Experimental design
At approx. 1 day of adulthood, virgin LW and SW G. firmus of both sexes were isolated and individually housed in translucent plastic containers (473 ml) with ad libitum access to water. Experimental males (n = 210 total) were maintained in standard conditions (see above). To address Hypotheses 1 and 2 (environmental variability influences a tradeoff between investment into early and late reproduction and influences DA, respectively), a 2 × 2 factorial design was used with females of both wing morphs (LW: n = 107; SW: 103) to manipulate food availability and temperature variability throughout adulthood—that is, eight treatment group combinations (n = 210 total). Each female’s access to food (dry cat food) was manipulated: ad libitum access (n = 98) or intermittent access (n = 112; cat food was only available for 3 h twice per week: sensu Adamo et al. 2012; Stahlschmidt et al. 2013, 2015). In addition, each female was maintained in an incubator (model I-36, Percival Scientific, Inc., Perry, IA, U.S.) set at one of two temperature regimes: constant 28 °C (n = 99) or a fluctuating cycle (n = 111), in which temperature ranged from 23 to 33 °C and changed each hour (i.e., a 28 ± 5 °C diel cycle: Fig. S1). These temperature fluctuations approximate the average diel (daily) temperature variation in Gainesville, FL, USA, which is where the founders of the stock were collected during the crickets’ active season (May–September; mean daily minimum and maximum: 21 and 33 °C, respectively: National Weather Service). Therefore, our manipulations created a gradient of environmental conditions from highly stable (constant temperature combined with ad libitum food access) to highly variable (both fluctuating temperature and intermittent food access).
At approx. 8 days of adulthood (i.e., 7 days after isolation), each female was randomly paired with a male from the same block in a 1.9-L translucent plastic container to facilitate mating. Males were virgin, the same age as their female partners, and individually housed throughout adulthood in standard conditions (see above) prior to mating. To avoid cannibalism, intermittently fed females were allowed access to food throughout this period. After 24 h, females were returned to individual translucent plastic containers in their treatment conditions (see above), and males were removed, weighed, and frozen at − 20 °C. Later, males were measured for the following fitness-related traits: head width (a proxy for the size of mandibles, which are sexually dimorphic and can be used during agonistic contests: e.g., Alexander 1961; Adamo and Hoy 1995) and femur length (a proxy for body size: Simmons 1986; Glass and Stahlschmidt 2019). Male body mass, femur length, and head width were highly correlated with one another, and the first principal component (PC1) extracted from a principal components analysis explained 84% of the total variance in these three traits. A male with a high PC1 value was relatively heavy, large, and had a wide head.
To address Hypothesis 2 (environmental variability influences DA), PC1 was subsequently used as a proxy for male quality. In G. firmus, PC1 strongly predicts dominance among males (Nguyen and Stahlschmidt 2019), and larger G. firmus males sire more offspring than smaller males (Saleh et al. 2014). In other Gryllus crickets, larger males achieve more matings than smaller males (Simmons 1986), and male dominance is associated with greater mating success (Rantala and Kortet 2004), which can be facilitated by mate guarding (Wynn and Vahed 2004). The advantage of dominance in the mating success of male Gryllus can be eliminated if males are quickly introduced to females after male–male agonistic contests (Vedenina and Shestakov 2018)—however, we avoided this potential issue by eliminating male-male interactions throughout the study period.
After males were removed, each female was left in its 1.9-L container and given egg-laying substrate (cotton-plugged water bottle) for three weeks. Although G. firmus can live longer in laboratory conditions (ZRS unpublished), they live only up to 25 days post-adult molt in their natural environment (Zera et al. 2007). Therefore, our reproductive measurements (see below) refer to estimates of lifetime reproduction in the context of an ecologically relevant lifespan. Water bottles were replaced twice each week at which points oviposited eggs were counted to determine weekly egg-laying (e.g., to determine whether mate quality or the environment influenced females to bias their reproductive output toward early or late adulthood sensu Hypotheses 1 and 2 above). The total number of eggs oviposited over the course of the study was used to estimate females’ lifetime reproductive output, which allowed us to address all three hypotheses, because nearly 95% of oviposited eggs are fertilized in unhandled Gryllus (Shoemaker and Adamo 2007). At the end of the study (three weeks post-mating), females were removed, weighed, and frozen at − 20 °C.
Later, the lengths of females’ femurs were measured to determine body size, and females were dissected to determine the number of eggs retained in females’ lateral oviducts to estimate females’ lifetime reproductive effort (i.e., the sum of oviposited eggs and retained, unfertilized eggs). Given the rapid rate of body decomposition after death, the number of eggs retained by females that did not survive the study could not be determined. In addition to its utility in estimating lifetime reproductive effort, the number of eggs retained at the end of adulthood was determined to estimate adaptive decision-making (e.g., a female in a low-risk environment and mated to a low-quality male may delay fertilizing and ovipositing her eggs as she waits for a higher quality male: see Hypotheses 1 and 2 above). Similar to other studies in crickets (e.g., Lierheimer and Tinghitella 2017), nearly all females in our study were mated (i.e., 95% of surviving females had sperm-filled spermathecae) regardless of mate quality or environmental conditions. Due to the small number of unmated females and because mating strongly promotes reproductive effort (ZRS unpublished), unmated females were excluded from analyses. To minimize observer bias, blinded methods were used when data were recorded and/or analyzed.
Statistical analyses
Several linear models were performed in SPSS (v.22 IBM Corp., Armonk, NY), data were log-transformed as necessary (e.g., to meet the assumption of normally distributed residuals), and two-tailed significance was determined at α = 0.05. To determine effects on reproductive output and effort (i.e., number of eggs oviposited and produced, respectively), analyses of covariance (ANCOVAs) were performed. Fixed effects included food treatment, temperature treatment, and wing morphology, and covariates included female femur length (to control for body size) and male quality (PC1, see above). To determine effects on retained eggs, a similar ANCOVA model was used that also included the total number of eggs oviposited as a covariate. To determine effects on weekly reproductive output (number of eggs laid), a linear mixed effects model was performed. For this model, fixed effects included food treatment, temperature treatment, wing morphology, and age (1, 2, or 3 weeks post-mating). Covariates included female femur length and male quality, while female ID number was included as a random effect (to control for repeated [weekly] sampling). Although not explicitly incorporated into our hypotheses, survival is an important life-history metric (e.g., lifetime reproduction can be tightly linked to lifespan) that was determined using a binary logistic generalized linear model, which allowed for examining the independent and interactive fixed effects of food treatment, temperature treatment, and wing morphology. For all models, interactions between fixed effects and covariates were initially included, but non-significant interactions were removed in final (reported) model results.
Results
For the females in our study (n = 210 total), survival to the end of the study period (three weeks post-mating) was affected by food treatment (χ2 = 6.8, df = 1, P = 0.0091) and temperature treatment (χ2 = 5.2, df = 1, P = 0.022), but not by wing morphology (herein, “morph”; χ2 = 0.14, df = 1, P = 0.71). More females with ad libitum access to food survived (mean ± s.e.m.: 86 ± 4% vs. 70 ± 4%), as well as those kept at a constant temperature (85 ± 4% vs. 71 ± 4% survival).
Estimated lifetime reproductive output (number of eggs oviposited) was not independently affected by food treatment (F1,196 = 0.81, P = 0.37), temperature treatment (F1,196 = 0.15, P = 0.70), female body size (F1,196 = 0.86, P = 0.36), morph (F1,196 = 1.3, P = 0.26), or male quality (F1,196 = 3.2, P = 0.075). However, it was affected by interactions between mate quality and environmental conditions (temperature × PC1: F1,196 = 4.1, P = 0.044)—females laid more eggs when mated to higher quality males and kept at a constant temperature (Pearson correlation: n = 94, R = 0.31, P = 0.0023), but not in the fluctuating temperature treatment (n = 106, R = 0.12, P = 0.23). There was also an interactive effect of mate quality, food treatment, and temperature treatment on lifetime egg-laying (F2,196 = 4.9, P = 0.0084; Fig. 1) where females mated to higher quality males laid more eggs only in highly stable (constant temperature and ad libitum access to food; Pearson correlation: n = 46, R = 0.48, P < 0.001) conditions.
Effect of male quality (principal component explaining 84% of the total variance in body mass, femur length, and head width: see text for details) on estimated lifetime reproductive output (number of eggs oviposited) in female G. firmus. Throughout adulthood, females experienced either a constant (28 °C) or a fluctuating (28 ± 5 °C) daily temperature regime (gray and white symbols, respectively) and either intermittent or ad libitum food availability (square and diamond symbols, respectively). A line of best fit is included for the significant relationship (P < 0.05) for females with ad libitum food availability and at a constant temperature. For clarity, wing morphology (short-winged [SW] vs. long-winged [LW]) is not shown because it did not have a significant effect on estimated lifetime reproductive output (see text for details)
The number of eggs laid each week (e.g., the number of eggs laid the week after mating vs. the number laid 2 wks after mating) was affected by female age (F2,570 = 39, P < 0.0001; Fig. 2), and mate quality (F1,570 = 7.2, P = 0.0074) where weekly egg-laying was higher during early adulthood and in females mated to larger (presumably, higher quality) males. Week-to-week egg-laying was not affected by food treatment (F1,570 = 2.1, P = 0.15), temperature treatment (F1,570 = 0.51, P = 0.48), morph (F1,570 = 2.6, P = 0.11), or female body size (F1,570 = 2.3, P = 0.13). Females exhibited a greater age-related decline in oviposition in fluctuating temperature conditions (Fig. 2; age × temperature: F2,570 = 3.0, P = 0.049). However, the effect of temperature variability on temporal egg-laying dynamics was influenced by wing morphology—SW females, in particular, exhibited a blunted age-related decline in oviposition in constant temperature conditions (Fig. 2; age × temperature × morph: F5,570 = 2.9, P = 0.013). There was also an interactive effect of mate quality, food treatment, and temperature treatment on week-to-week egg-laying where females mated to higher quality males laid more eggs each week only in highly stable (constant temperature and ad libitum access to food) conditions (F3,570 = 6.8, P < 0.001).
Effects of temperature treatment (constant vs. fluctuating) and wing morphology (short-winged [SW] vs. long-winged [LW]) on weekly reproductive output of G. firmus. Females were mated 1 week into adulthood, and eggs were counted for three weeks thereafter (i.e., 2nd, 3rd, and 4th weeks of adulthood). For clarity, food treatment (ad libitum vs. intermittent access) is not shown because it did not have a significant effect (see text for details). Values are displayed as mean ± s.e.m.
Estimated lifetime reproductive effort (sum of oviposited and retained eggs) was independently affected by food treatment (F1,180 = 38, P < 0.001; Fig. 3a) and female body size (F1,180 = 13, P < 0.001; Fig. S2) where larger females and those with ad libitum access to food produced more eggs over their lifetimes. Estimated lifetime reproductive effort was not influenced by temperature treatment (F1,180 = 0.043, P = 0.84), morph (F1,180 = 3.5, P = 0.064), or male quality (F1,180 = 1.0, P = 0.31). Yet, it was affected by an interaction between food and temperature treatments—fluctuating temperatures promoted egg production when food was limited while constant temperature promoted egg production when food was abundant (Fig. 3a: F4,180 = 3.9, P = 0.0045).
Effects of temperature treatment (constant vs. fluctuating) and food treatment (ad libitum vs. intermittent availability) on (a) estimated lifetime reproductive effort and (b) retained, unfertilized eggs in female G. firmus. Significant effects, including covariates (not shown), are described in each panel. For clarity, wing morphology (short- vs. long-winged morph) is not shown because it did not have a significant effect (see text for details). Values are displayed as mean ± s.e.m.
The number of retained, unfertilized eggs was affected by food treatment (F1,161 = 45, P < 0.001; Fig. 3b), and it was associated with lifetime reproductive output (F1,161 = 20, P < 0.001), female body size (Fig. S2; F1,161 = 17, P < 0.001), and mate quality (F1,161 = 4.1, P = 0.045; Fig. 4). Females retained more eggs when they (1) laid fewer eggs, (2) had ad libitum access to food (Fig. 3b), (3) were larger, and (4) were mated to lower quality males (Fig. 4). Neither temperature treatment alone nor morph affected egg retention (temperature: F1,161 = 0.002, P = 0.97; morph: F1,180 = 2.9, P = 0.093). There was an interactive effect of food and temperature treatment—fluctuating temperatures promoted egg retention when food was limited, while constant temperature promoted egg retention when food was abundant (Fig. 3b: F1,161 = 4.8, P = 0.030).
Effect of male quality (principal component explaining 84% of the total variance in body mass, femur length, and head width: see text for details) on the number of retained, unfertilized eggs in female G. firmus. Throughout adulthood, females experienced either a constant (28 °C) or a fluctuating (28 ± 5 °C) daily temperature regime and either intermittent or ad libitum food availability. Data displayed were pooled across all treatment groups for clarity, and a line of best fit is included for the significant relationship (P < 0.05)
Discussion
We demonstrate strong effects of biotic and abiotic factors on estimates of lifetime reproductive effort, output, and allocation (e.g., DA and the balance between egg retention and oviposition; Figs. 1, 2, and 3), as well as on survival. Larger female G. firmus and those putatively acquiring more resource (i.e., those with ad libitum access to food) invested more into lifetime reproductive effort (estimated reproductive biomass: Fig. 3a; Fig. S1) as in other taxa (Wootton 1979; Peters 1983; Shine 1988; Honek 1993; Wheeler 1996; Kjesbu et al. 1998). Yet, lifetime reproductive output (estimated number of offspring) responded differently than reproductive effort because reproductive output was influenced by interactions between temperature variability and mate quality (Fig. 1). Therefore, lifetime reproductive effort and output were uncoupled due to variation in reproductive allocation because not all eggs produced were fertilized (Fig. 3). Further, survival over an ecologically relevant timeframe (28 days, which approximates the maximum lifespan of G. firmus in nature: Zera et al. 2007) was affected by a different set of factors (variation in food availability and temperature). Thus, it is important to carefully consider the costs and benefits of lifetime reproduction (i.e., energetic investment of reproductive effort and offspring number of reproductive output, respectively), as well as survival, when determining the effects of complex environmental shifts on animal life histories.
We found partial support for our first hypothesis that environmental variability influences a tradeoff between investment into early and late reproduction (particularly in SW females: Fig. 2). Female G. firmus experiencing fluctuating temperatures were biased toward early (not late) reproductive output because they oviposited more during early adulthood (temperature × age effect with a steep decline from Week 2 to Week 3: Fig. 2) relative to those experiencing constant temperature. However, this effect was influenced by an interaction with wing morphology (Fig. 2), and we did not detect a similar effect due to variability in food availability. Nonetheless, our results suggest that decision-making related to reproductive allocation (temporal dynamics of oviposition) was influenced by variability in temperature, a biologically important environmental factor that naturally exhibits spatiotemporal variation (reviewed in Angilletta 2009). This result agrees with the effects of resource (food) limitation on the current-future (or early-late) tradeoff in reproductive allocation in vertebrates where reproductive investment is biased toward early adulthood when resources are limited (Lemaitre et al. 2015). Developmental plasticity was not examined in our study, and it may also play a role in early-late allocation decisions because an animal born and reared in poor conditions (e.g., those in which food availability is low) may likewise invest more heavily into early reproduction (reviewed in Douhard et al. 2016). However, continued work is required to understand how life histories are influenced by environmental variability or fluctuation, rather than mean environmental values (e.g., consistent low- vs. high-food availability)—particularly, with regard to the effects of multiple, co-varying environmental factors (Todgham and Stillman 2013; Kaunisto et al. 2016) across developmental stages.
We found support for only the first prediction of our second hypothesis that environmental stability promotes positive DA. In support, female G. firmus mated to higher quality males laid more eggs only in highly stable conditions (constant temperature and ad libitum access to food; Fig. 1), which is indicative of positive DA (as in other taxa, including Gryllus: Burley 1986; Sheldon 2000; Bretman et al. 2006). In our study, stable environments were considered higher quality than unstable ones given constant temperature, food availability were associated with increased survival, and ad libitum access to food was further associated with increased lifetime egg production (Fig. 3a). Thus, a female in stable conditions that was mated to a low-quality male presumably had the luxury to forego oviposition in the short-term given the reduced risk of mortality (sensu the “terminal investment hypothesis”: Clutton-Brock 1984; Lemaitre et al. 2015; reviewed in Duffield et al. 2017). She could then “trade up” by mating with a higher quality male in the future because multiple mating, including polyandry, is common in Gryllus (Gershman 2010; Worthington and Kelly 2016). Although females in our study exerted choice after mating, others have explored this phenomenon in mate choice. As examples, food limitation strengthened the preference for mate quality in some studies (fish: Fisher and Rosenthal 2006; spider: Moskalik and Uetz 2011), but opposing results have also been demonstrated (spider: Eraly et al. 2009; beetle: Fox and Moya-Larano 2009). Understanding the tradeoff between the number and size of offspring (Smith and Fretwell 1974; Brockelman 1975) may clarify contrasting results. For example, food-limited Gryllus favor offspring size (not measured in our study) over offspring number (Stahlschmidt and Adamo 2015). Further, these studies did not examine effects of temperature variation. Thus, we encourage continued investigations of combined manipulations of temperature and food availability on pre- and post-copulatory decision-making given the natural co-variation of these two environmental factors (Stamp 1993).
We found no support for our third hypothesis that the wing dimorphism exhibited by G. firmus influences lifetime reproduction. Gryllus crickets and G. firmus, in particular, have been powerful models used to examine the genetics, physiology, and ecology of life-history tradeoffs (reviewed in Zera and Harshman 2001; Zera 2005; King et al. 2011). These studies have generally shown that investment into flight musculature by LW females obligates reduced investment into ovarian tissue relative to SW females during early adulthood (i.e., < 1 week into adulthood). This fecundity advantage by young SW females is even robust to food limitation (Roff and Gelinas 2003), but it disappears after one week into adulthood (Mole and Zera 1994; Zera et al. 1997). Multiple years of fieldwork on G. firmus indicates that the average age of adults in the field is 11–14 days post-adult molt, with a maximum age of 25 days post-adult molt (Zera et al. 2007). We show that the total number of eggs oviposited and produced over 21 days in a 28-day lifespan was not independently affected by wing morphology. Thus, LW females were able to “catch up” with SW females over their lifetimes, and SWs did not exhibit an oviposition advantage during any age we examined (Fig. 2). By estimating lifetime reproductive effort (biomass invested into reproduction) and output (number of offspring produced) in both G. firmus morphs, our study indicates that the costs of dispersal (flight) capacity to lifetime reproduction can be negligible in some environmental contexts.
Oviposition decisions in Gryllus crickets exhibit adaptive plasticity in response to biotic and abiotic factors (e.g., food availability, temperature, and predation risk: Stahlschmidt and Adamo 2013; Stahlschmidt et al. 2014). Similarly, our results demonstrate an uncoupling of reproductive effort and output where many eggs produced were not oviposited (Fig. 3), and the decision-making process to retain or oviposit eggs was sensitive to widespread biotic and abiotic factors (Figs. 1 and 3). For example, females mated to higher quality males exhibited reduced egg retention (i.e., these females fertilized a large proportion of their eggs; Fig. 4), which indicates adaptive post-copulatory decision-making. Females (particularly, larger females) mated to lower quality males may have foregone egg fertilization with the intention of mating with higher quality males in the future (see above). Indeed, there tend to be indirect (genetic) benefits of polyandry across taxa (Slatyer et al. 2012), and there are also direct (non-genetic) benefits of multiple mating in Gryllus (e.g., increased oviposition: Gershman 2010; Worthington and Kelly 2016). In addition, aspects of the abiotic environment interacted to influence egg retention (Fig. 3b) where, for example, the average female at a constant temperature and with ad libitum access to food retained over 45% of the eggs she produced over her lifetime. Thus, stable (benign) environmental conditions may promote post-copulatory choosiness, which agrees with our results indicating positive DA in stable environmental conditions. Because females in our study were given only a single mating opportunity, future work is required to understand the effects of environmental variability on the benefits of multiple mating, the temporal dynamics of mate choice, and post-copulatory decision-making (e.g., cryptic female choice or sperm competition) (Evans and Garcia-Gonzalez 2016). We also welcome investigations into the physiological mechanisms underlying DA and post-copulatory decisions in insects. For example, oviposition is regulated by juvenile hormone (JH) in crickets (Strambi et al. 1997), and JH titers are plastic in response to circadian rhythms and wing morphology in G. firmus (Zera and Cisper 2001).
In sum, our study used an experimental approach to continue to clarify the importance of environmental variability in three themes of behavioral ecology and life history—the tradeoff between early and late reproduction, the role of mate quality in reproductive effort and output (i.e., differential allocation), and a polymorphism mediating a reproduction-related tradeoff. Complex environmental variability has been integrated into ecology (ecological synergies: reviewed in Darling and Cote 2008) and physiology (multiple environmental stressors: reviewed in Todgham and Stillman 2013). Although theoretical models are important (e.g., Hastings and Caswell 1979; Orzack and Tuljapurkar 2001), we encourage future empirical efforts by behavioral ecologists because, in general, studies manipulating single environmental variables can generate misguided results and interpretations (McBryan et al. 2013). Many environmental variables co-vary to influence aspects of behavioral ecology and life history (e.g., Stahlschmidt and DeNardo 2010; Stahlschmidt et al. 2011; Kaunisto et al. 2016; Nguyen and Stahlschmidt 2019; Cronin et al. 2019), and we welcome continued work on the effects of other environmental combinations (e.g., pH and salinity in aquatic systems) on tradeoffs and reproductive decision-making.
Data availability statement
The datasets generated and analyzed during the current study are available from the corresponding author on reasonable request.
References
Adamo SA, Hoy RR (1995) Agonistic behavior in male and female field crickets, GRYLLUS-BIMACULATUS, and how behavioral context influences its expression. Anim Behav 49:1491–1501
Adamo SA, Baker JL, Lovett MME, Wilson G (2012) Climate change and temperate zone insects: the tyranny of thermodynamics meets the world of limited resources. Environ Entomol 41:1644–1652
Alexander RD (1961) Aggressiveness, territoriality, and sexual behavior in field crickets (Orthoptera: Gryllidae). Behaviour 17:130–223
Anderson JL, Albergotti L, Ellebracht B, Huey RB, Phillips PC (2011) Does thermoregulatory behavior maximize reproductive fitness of natural isolates of Caenorhabditis elegans? BMC Evol Biol 11:157
Andersson M (1994) Sexual selection. Princeton University Press, Princeton
Angilletta MJ (2009) Thermal adaptation: a theoretical and empirical synthesis. University Press, Oxford
Boggs CL, Ross CL (1993) The effect of adult food limitation on life history traits in Speyeria mormonia (Lepidoptera: Nymphalidae). Ecology 74:433–441
Both C, Visser ME (2005) The effect of climate change on the correlation between avian life-history traits. Glob Chang Biol 11:1606–1613
Bretman A, Rodriguez-Munoz R, Tregenza T (2006) Male dominance determines female egg laying rate in crickets. Biol Lett 2:409–411
Brockelman WY (1975) Competition, the fitness of offspring, and optimal clutch size. Am Nat 109:677–699
Brommer JE (2000) The evolution of fitness in life-history theory. Biol Rev 75:377–404
Burley N (1986) Sexual selection for aesthetic traits in species with biparental care. Am Nat 127:415–445
Capinera JL, Scott RD, Walker TJ (2004) Field guide to grasshoppers, crickets, and katydids of the United States. Cornell University Press, Ithaca
Carriere Y, Roff DA (1995) The evolution of offspring size and number - a test of the Smith-Fretwell model in three species of crickets. Oecologia 102:389–396
Charmantier A, McCleery RH, Cole LR, Perrins C, Kruuk LEB, Sheldon BC (2008) Adaptive phenotypic plasticity in response to climate change in a wild bird population. Science 320:800–803
Clutton-Brock TH (1984) Reproductive effort and terminal investment in iteroparous animals. Am Nat 123:212–229
Cody ML (1966) A general theory of clutch size. Evolution 20:174–184
Connallon T, Hall MD (2016) Genetic correlations and sex-specific adaptation in changing environments. Evolution 70:2186–2198
Crnokrak P, Roff DA (1995) Fitness differences associated with calling behaviour in the two wing morphs of male sand crickets, Gryllus firmus. Anim Behav 50:1475–1481
Cronin AD, Ryan MJ, Page RA et al (2019) Environmental heterogeneity alters mate choice behavior for multimodal signals. Behav Ecol Sociobiol 73:43
Darling ES, Cote IM (2008) Quantifying the evidence for ecological synergies. Ecol Lett 11:1278–1286
Dillon ME, Woods HA, Wang G, Fey SB, Vasseur DA, Telemeco RS, Marshall K, Pincebourde S (2016) Life in the frequency domain: the biological impacts of changes in climate variability at multiple time scales. Integr Comp Biol 56:14–30
Douhard M, Loe LE, Stien A, Bonenfant C, Irvine RJ, Veiberg V, Ropstad E, Albon S (2016) The influence of weather conditions during gestation on life histories in a wild Arctic ungulate. Proc R Soc Lond B 283:20161760
Duffield KR, Bowers EK, Sakaluk SK, Sadd BM (2017) A dynamic threshold model for terminal investment. Behav Ecol Sociobiol 71:185
Eraly D, Hendrickx F, Lens L (2009) Condition-dependent mate choice and its implications for population differentiation in the wolf spider Pirata piraticus. Behav Ecol 20:856–863
Evans JP, Garcia-Gonzalez F (2016) The total opportunity for sexual selection and the integration of pre- and post-mating episodes of sexual selection in a complex world. J Evol Biol 29:2338–2361
Fisher HS, Rosenthal GG (2006) Hungry females show stronger mating preferences. Behav Ecol 17:979–981
Flatt T, Heyland A (2011) Mechanisms of life history evolution the genetics and physiology of life history traits and trade-offs. Oxford University Press, Oxford
Fox CW, Moya-Larano J (2009) Diet affects female mating behaviour in a seed-feeding beetle. Physiol Entomol 34:370–378
Gershman SN (2010) Large numbers of matings give female field crickets a direct benefit but not a genetic benefit. J Insect Behav 23:59–68
Glass JR, Stahlschmidt ZR (2019) Should I stay or should I go? Complex environments influence the developmental plasticity of flight capacity and flight-related trade-offs. Biol J Linn Soc blz073. https://doi.org/10.1093/biolinnean/blz073
Gotthard K, Berger D, Walters R (2007) What keeps insects small? Time limitation during oviposition reduces the fecundity benefit of female size in a butterfly. Am Nat 169:768–779
Gowaty PA (2008) Reproductive compensation. J Evol Biol 21:1189–1200
Guerra P (2011) Evaluating the life-history trade-off between dispersal capability and reproduction in wing dimorphic insects: a meta-analysis. Biol Rev 86:813–835
Haaland TR, Wright J, Kuijper B, Ratikainen II (2017) Differential allocation revisited: when should mate quality affect parental investment? Am Nat 190:534–546
Harris WE, Uller T (2009) Reproductive investment when mate quality varies: differential allocation versus reproductive compensation. Philos Trans R Soc B 364:1039–1048
Harshman LG, Zera AJ (2007) The cost of reproduction: the devil in the details. Trends Ecol Evol 22:80–86
Hastings A, Caswell H (1979) Role of environmental variability in the evolution of life history strategies. Proc NatI Acad Sci U S A 76:4700–4703
Hervé C, Brent JS, Philippe V, David R (2015) Insects in Fluctuating Thermal Environments. Annual Review of Entomology 60:1, 123–140
Honek A (1993) Intraspecific variation in body size and fecundity in insects – a general relationship. Oikos 66:483–492
Horvathova T, Nakagawa S, Uller T (2012) Strategic female reproductive investment in response to male attractiveness in birds. Proc R Soc Lond B 279:163–170
Kaunisto S, Ferguson LV, Sinclair BJ (2016) Can we predict the effects of multiple stressors on insects in a changing climate? Curr Opin Insect Sci 17:55–61
King EG, Roff DA, Fairbairn DJ (2011) Trade-off acquisition and allocation in Gryllus firmus: a test of the Y model. J Evol Biol 24:256–264
Kirk KL (1997) Life-history responses to variable environments: starvation and reproduction in planktonic rotifers. Ecology 78:434–441
Kjesbu OS, Witthames PR, Solemdal P, Walker MG (1998) Temporal variations in the fecundity of Arcto-Norwegian cod (Gadus morhua) in response to natural changes in food and temperature. J Sea Res 40:303–321
Kleinteich A, Wilder SM, Schneider JM (2015) Contributions of juvenile and adult diet to the lifetime reproductive success and lifespan of a spider. Oikos 124:130138
Korpimaki E, Wiehn J (1998) Clutch size of kestrels: seasonal decline and experimental evidence for food limitation under fluctuating food conditions. Oikos 83:259–272
Lemaitre JF, Berger V, Bonenfant C, Douhard M, Gamelon M, Plard F, Gaillard JM (2015) Early-late life trade-offs and the evolution of ageing in the wild. Proc R Soc Lond B 282:20150209
Lierheimer VF, Tinghitella RM (2017) Quantity and quality of available mates alters female responsiveness but not investment in the Pacific field cricket, Teleogryllus oceanicus. Behav Ecol Sociobiol 71:80
Long TAF, Agrawal AF, Rowe L (2012) The effect of sexual selection on offspring fitness depends on the nature of genetic variation. Curr Biol 22:204–208
Lorch PD, Proulx S, Rowe L, Day T (2003) Condition-dependent sexual selection can accelerate adaptation. Evol Ecol Res 5:867–881
Magnhagen C (1991) Predation risk as a cost of reproduction. Trends Ecol Evol 6:183–185
McBryan TL, Anttila K, Healy TM, Schulte PM (2013) Responses to temperature and hypoxia as interacting stressors in fish: implications for adaptation to environmental change. Integr Comp Biol 53:648–659
Mole S, Zera AJ (1994) Differential resource consumption obviates a potential flight fecundity trade-off in the sand cricket (GRYLLUS-FIRMUS). Funct Ecol 8:573–580
Moskalik B, Uetz GW (2011) Female hunger state affects mate choice of a sexually selected trait in a wolf spider. Anim Behav 81:715–722
Nguyen K, Stahlschmidt ZR (2019) When to fight? Disentangling temperature and circadian effects on aggression and agonistic contests. Anim Behav 148:1–8
Orzack SH, Tuljapurkar S (2001) Reproductive effort in variable environments, or environmental variation IS for the birds. Ecology 82:2659–2665
Peters RH (1983) The ecological implications of body size. Cambridge University Press, Cambridge
Rantala MJ, Kortet R (2004) Male dominance and immunocompetence in a field cricket. Behav Ecol 15:187–191
Ratikainen II, Kokko H (2010) Differential allocation and compensation: who deserves the silver spoon? Behav Ecol 21:195–200
Roff DA (1994) Habitat persistence and the evolution of wing dimorphism in insects. Am Nat 144:772–798
Roff DA (2002) Life history evolution. Sinauer Press, Sunderland
Roff DA, Gelinas MB (2003) Phenotypic plasticity and the evolution of trade-offs: the quantitative genetics of resource allocation in the wing dimorphic cricket, Gryllus firmus. J Evol Biol 16:55–63
Royle NJ, Smiseth PT, Kolliker M (2012) The evolution of parental care. Oxford University Press, Oxford
Saleh NW, Larson EL, Harrison RG (2014) Reproductive success and body size in the cricket Gryllus firmus. J Insect Behav 27:346–356
Sears MW, Angilletta MJ, Schuler MS, Borchert J, Dilliplane KF, Stegman M, Rusch TW, Mitchell WA (2016) Configuration of the thermal landscape determines thermoregulatory performance of ectotherms. Proc Natl Acad Sci U S A 113:10595–10600
Sheldon BC (2000) Differential allocation: tests, mechanisms and implications. Trends Ecol Evol 15:397–402
Shine R (1988) The evolution of large body size in females: a critique of Darwin's ‘fecundity advantage’ model. Am Nat 131:124–131
Shoemaker KL, Adamo SA (2007) Adult female crickets, Gryllus texensis, maintain reproductive output after repeated immune challenges. Physiol Entomol 32:113–120
Simmons LW (1986) Inter-male competition and mating success in the field cricket, Gryllus bimaculatus (De Geer). Anim Behav 34:567–579
Slatyer RA, Mautz BS, Backwell PRY, Jennions MD (2012) Estimating genetic benefits of polyandry from experimental studies: a meta-analysis. Biol Rev 87:1–33
Smith CC, Fretwell SD (1974) Optimal balance between size and number of offspring. Am Nat 108:499–506
Stahlschmidt ZR, Adamo SA (2013) Warm and cozy: temperature and predation risk interactively affect oviposition-site selection. Anim Behav 86:553–558
Stahlschmidt ZR, Adamo SA (2015) Food-limited mothers favour offspring quality over offspring number: a principal components approach. Funct Ecol 29:88–95
Stahlschmidt ZR, DeNardo DF (2010) Parental behavior in pythons is responsive to both the hydric and thermal dynamics of the nest. J Exp Biol 213:1691–1696
Stahlschmidt ZR, Brashears J, DeNardo DF (2011) The role of temperature and humidity in python nest site selection. Anim Behav 81:1077–1081
Stahlschmidt ZR, Rollinson N, Acker M, Adamo SA (2013) Are all eggs created equal? Food availability and the fitness trade-off between reproduction and immunity. Funct Ecol 27:800–806
Stahlschmidt ZR, O'Leary ME, Adamo SA (2014) Food limitation leads to risky decision-making and to tradeoffs with oviposition. Behav Ecol 25:23–27
Stahlschmidt ZR, Acker M, Kovalko I, Adamo SA (2015) The double-edged sword of immune defence and damage control: Do food availability and immune challenge alter the balance? Functional Ecology. 29, 1445–1452
Stamp NE (1993) A temperate region view of the interaction of temperature, food quality, and predators on caterpillar foraging. In: Stamp NE, Casey TM (eds) Caterpillars: ecological and evolutionary constraints on foraging. Chapman and Hall, New York
Stearns SC (1992) Evolution of life histories. Oxford University Press, New York
Strambi A, Strambi C, Cayre M (1997) Hormonal control of reproduction and reproductive behavior in crickets. Arch Insect Biochem Physiol 35:393–404
Todgham AE, Stillman JH (2013) Physiological responses to shifts in multiple environmental stressors: relevance in a changing world. Integr Comp Biol 53:539–544
van Noordwijk AJ, de Jong G (1986) Acquisition and allocation of resources: their influence on variation in life history tactics. Am Nat 128:137–142
Vedenina VY, Shestakov LS (2018) Loser in fight but winner in love: how does inter-male competition determine the pattern and outcome of courtship in cricket Gryllus bimaculatus? Front Ecol Evol 6:197
Wheeler D (1996) The role of nourishment in oogenesis. Annu Rev Entomol 41:407–431
Whitlock MC, Agrawal AF (2009) Purging the genome with sexual selection: reducing mutation load through selection on males. Evolution 63:569–582
Williams GC (1966) Natural selection, the costs of reproduction, and a refinement of Lack's principle. Am Nat 100:687–690
Wootton RJ (1979) Energy cost of egg reproduction and environmental determinants of fecundity in teleost fishes. Symp Zool Soc Lond 44:133–159
Worthington AM, Kelly CD (2016) Direct costs and benefits of multiple mating: are high female mating rates due to ejaculate replenishment? Behav Process 124:115–122
Wynn H, Vahed K (2004) Male Gryllus bimaculatus guard females to delay them from mating with rival males and to obtain repeated copulations. J Insect Behav 17:53–66
Zammuto RM, Millar JS (1985) Environmental predictability, variability, and Spermophilus columbianus life history over an elevational gradient. Ecology 66:1784–1794
Zera AJ (2005) Intermediary metabolism and life history trade-offs: lipid metabolism in lines of the wing-polymorphic cricket, Gryllus firmus, selected for flight capability vs. early age reproduction. Integr Comp Biol 45:511–524
Zera AJ, Cisper G (2001) Genetic and diurnal variation in the juvenile hormone titer in a wing-polymorphic cricket: implications for the evolution of life histories and dispersal. Physiol Biochem Zool 74:293–306
Zera AJ, Denno RF (1997) Physiology and ecology of dispersal polymorphism in insects. Annu Rev Entomol 42:207–230
Zera AJ, Harshman LG (2001) The physiology of life history trade-offs in animals. Annu Rev Ecol Syst 32:95–126
Zera AJ, Sall J, Grudzinski K (1997) Flight-muscle polymorphism in the cricket Gryllus firmus: muscle characteristics and their influence on the evolution of flightlessness. Physiol Zool 70:519–529
Zera AJ, Zhao Z, Kaliseck K (2007) Hormones in the field: evolutionary endocrinology of juvenile hormone and ecdysteroids in field populations of the wing-dimorphic cricket Gryllus firmus. Physiol Biochem Zool 80:592–606
Acknowledgments
We thank Andy Byeon, Grace Cho, Jordan Glass, Stephanie Ha, Narin Jeong, David Luc, Garrett Masuda, Katherine Nguyen, and Carolyn Pak for animal care and/or experimental assistance. We also appreciate feedback on the manuscript from Jordan Glass, Dustin Johnson, and an anonymous reviewer.
Funding
This study was financially supported by the National Science Foundation (IOS-1565695 to ZRS) and University of the Pacific (to ZRS).
Author information
Authors and Affiliations
Corresponding author
Additional information
Communicated by K. Shaw
Publisher’s note
Springer Nature remains neutral with regard to jurisdictional claims in published maps and institutional affiliations.
Electronic supplementary material
ESM 1
(DOCX 23 kb)
Rights and permissions
About this article
Cite this article
Stahlschmidt, Z.R., Chu, I. & Koh, C. When do looks matter? Effects of mate quality and environmental variability on lifetime reproduction. Behav Ecol Sociobiol 74, 11 (2020). https://doi.org/10.1007/s00265-019-2790-9
Received:
Revised:
Accepted:
Published:
DOI: https://doi.org/10.1007/s00265-019-2790-9