Abstract
Bacterial opportunistic pathogens are defined as microorganisms causing disease in hosts experiencing atypical environmental stressors or having impaired immune function. In intensive aquacultural rearing, stress factors (such as hypoxia, abnormal pH, and high population density) generate an optimal setting for such pathogens to thrive. The status of these organisms—either as natural components of a healthy microbiome, or a latent step in disease establishment, or both—is still not entirely clear. In this chapter, we outline the current understanding (i.e., taxonomy, biology, disease impact, and current treatment options) of major opportunist bacterial genera of special interest in aquaculture: Aeromonas, Flavobacterium, and Vibrio. On a broader scale, we consider the importance of host/microbiota/environment interactions in opportunistic infections of teleost fish. Not only does this cross talk play a crucial role in defining disease, but their importance also reveals novel strategies to prevent and cure opportunistic diseases. As such, preventive measures to reduce host stress, along with active interventions to enhance (or restore) the protective effect of the microbiome (i.e., prebiotics, probiotics, synbiotics), can mitigate bacterial opportunistic diseases.
Access provided by Autonomous University of Puebla. Download chapter PDF
Similar content being viewed by others
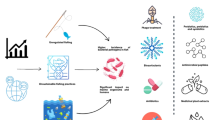
Keywords
- Bacteria
- Opportunistic pathogens
- Aeromonas
- Flavobacter
- Vibrio
- Fish
- Teleosts
- Host–microbiota interactions
- Novel treatment strategies
- Prebiotics
- Probiotics
- Synbiotics
1 Introduction
In recent decades, we have developed a better understanding of the role of microorganisms in beneficial long-term interactions with their host. Fish mucosae (skin, gills, and gut) and associated microbiota are an important primary defense barrier against pathogens (Trivedi 2012). Endogenous fish bacteria contribute to host defense in several ways:
-
1.
Colonization resistance (CR) which prevents pathogen growth with competitive use of resources (Dillon and Charnley 2002)
-
2.
Friction-preventing polymers
-
3.
Stimulation of the innate immune response
-
4.
Production of inhibitory compounds (Austin 2006; Ramsey and Whiteley 2009)
Disturbance of the relative taxonomic abundance of commensal microbiota (i.e., dysbiosis, often representing broken integrity of the microbiome (Dillon and Charnley 2002, see Sect. 4.2.3) is linked to specific human diseases (Frank et al. 2011). The decrease of commensal nonpathogenic bacteria in fish mucosae is correlated to increased abundance of potential pathogens (Boutin et al. 2013b). In many cases, pathogens may be isolated from healthy fish (Cahill 1990; Austin 2006). It is not clear whether asymptomatic carriers are merely a latent step of a disease cycle. Nonetheless, known pathogenic organisms present as a component of healthy fish microbiota are termed “opportunistic pathogens” (Austin and Austin 2007). Here, we review bacterial opportunistic pathogens involved in fish disease.
2 Overview
Opportunistic pathogens are defined as microorganisms associated with disease only in host individuals experiencing atypical environmental stressors or having impaired immune function (Boutin et al. 2013b). Most pathogens that impact aquaculture are deemed opportunistic. Important bacterial pathogens are represented by multiple species and several genera (Austin 2006). In this chapter, we focus on three genera with special reference to aquaculture: Aeromonas, Flavobacterium, and Vibrio.
2.1 The Genus Aeromonas
Aeromonas is a genus belonging to the Gammaproteobacteria that is ubiquitous in freshwater (Cahill 1990) and commonly found in the commensal microbiota of aquatic or terrestrial animals, plants, and natural soils (Abbott et al. 1992). Species among this genus are mainly pathogenic to fish and, to some extent, mammals and reptiles (Janda and Abbott 2010). Aeromonads are separated into two major groups, according to their morphological traits and optimum growth conditions (Janda and Abbott 2010): motile aeromonads, which tend to be mesophilic, and nonmotile aeromonads, which develop in psychrophilic conditions (Janda and Duffey 1988). The main diseases associated with motile aeromonads vary from opportunistic infections of fish to bacterial gastroenteritis in humans leading to bacteremia in immunocompromised patients (Austin and Austin 2012a, b). Due to their low salinity and cold temperature requirements, nonmotile aeromonads are almost exclusively pathogenic to freshwater fish. Especially in industrial aquaculture, their contribution to economic losses is worldwide and substantial (Nielsen et al. 2001). Solely in the province of Quebec, Canada, aeromonads are responsible for 30–60 % of all infection diagnoses in farmed salmonids each year (Morin 2010). The conditions to which farmed fish are exposed have been shown to disturb their natural microbiota (Boutin et al. 2013b), leaving them more vulnerable to opportunistic pathogens (Stecher et al. 2013). Aeromonads apparently exploit dysbiosis in aquaculture to emerge as virulent and damaging.
2.1.1 Aeromonas hydrophila
This bacterium is a ubiquitous and potent opportunistic pathogen of fish and humans. Zoonosis is unlikely in healthy individuals, but may occur via contact with mucus or tissue from a carrier fish (Lowry and Smith 2007). In farmed freshwater fish, A. hydrophila causes motile aeromonas septicemia (MAS), found in two main forms (Cipriano et al. 1984): (1) The acute form induces internal hemorrhages and generalized bacteremia, without apparent external symptoms, except for darkening of the skin and erratic behavior. (2) The chronic form is characterized by skin ulcers and underlying necrosis of the musculature. Inflammatory cells are often absent in necrotized tissue, but abundant in the adjacent epidermis. Aeromonas hydrophila is naturally present in the microflora of healthy fish (Trust et al. 1979). However, it takes advantage of environmental stresses to which fish are exposed. High-nutrient diets, combined with the lack of oxygen-producing plankton, lead to faster oxygen depletion, which facilitates A. hydrophila outbreaks in farmed channel catfish (Plumb et al. 1976).
Aeromonas hydrophila consists of heterotrophic facultative–anaerobic Gram-negative bacteria. They are rod shaped and motile, and their size is from 0.3 to 1.0 μm wide by 1.0–3.5 μm long (Horneman et al. 2007). Layered cell surface proteins (S-layers) facilitate osmotolerance, resistance to bactericidal compounds, and increased adherence (Sara and Sleytr 2000). Other virulence factors include fimbriae for increased adherence to the host tissues, cytotoxic enterotoxin, and hemolysin. The AH-3 strain also expresses effector proteins secreted by a molecular nanosyringe complex known as the type III secretion system (T3SS). The expression of this complex is activated by low extracellular calcium concentrations and allows specific injection of effector proteins in eukaryotic cells of the host (Vilches et al. 2009). As for many Gammaproteobacteria, the T3SS is a key determinant in virulence (Coburn et al. 2007). Secreted effectors cause direct toxicity to infected cells by disrupting actin polymerization and disruption of intracellular signaling.
2.1.2 Aeromonas salmonicida
Aeromonas salmonicida subsp. salmonicida is the etiological agent of furunculosis, a ravaging opportunistic disease affecting salmonids in industrial aquaculture. Its role as the causative agent of salmonid furunculosis was first established near the end of the nineteenth century (Emmerich and Weibel 1890). In the province of Quebec, Canada, where the brook trout accounts for 95 % of farmed salmonids, furunculosis represents 30–60 % of all diagnoses of fish infections each year (Morin 2010). This disease is highly contagious and can decimate an experimental sea trout (Salmo trutta morpha trutta) and induce 75 % mortality in a group of brown trout (Salmo trutta morpha fario) in less than 7 days after challenge (Scott 1968). Zoonoses are not a concern, since the growth temperature range of this bacterium is not compatible with the internal temperature of humans. The concern is, however, not only on the high pathogenic potential of A. salmonicida between fish but on how its proliferation is facilitated in industrial fish culture systems. Elevated temperature makes optimum growth of A. salmonicida easier to achieve, and the high density of fish stocks allows rapid and proximal transmission of the bacteria between fish. Even outside aquaculture basins, high temperature (more than 18 °C) is correlated to increased abundance of A. salmonicida in the wild. Climate change has been shown to accelerate the prevalence of furunculosis in wild fish of the James Bay, Quebec, Canada (Tam et al. 2011).
Salmonid furunculosis manifests itself in three major forms (Cipriano and Bullock 2001): (1) The peracute form of furunculosis mostly affects fingerlings. Clinical indications are not obvious, since the only evident marks are darkening of the skin, slight exophthalmia, and premature death. (2) Acute infections affect mostly juvenile and adult salmonids. This form is notorious for the variety of physiological and behavioral damages induced in the infected fish. First signs include color darkening and hemorrhage at the base of fins and the oral cavity. This condition worsens with hemorrhages in internal and reproductive organs, necroses of the kidney and spleen, and also gross intestine congestion (Scott 1968). Aeromonas salmonicida then gains access to the bloodstream via the multiple internal lesions, which leads to generalized septicemia and, ultimately, death of the infected fish. (3) The chronic form of furunculosis primarily affects older fish or species which have greater innate resistance to infection by A. salmonicida, such as the rainbow trout (Oncorhynchus mykiss) (Scott 1968). It is this form of furunculosis which is characterized by boil-like lesions in varying numbers on the skin of infected fish. The underlying ulcers may extend deep into the musculature.
Aeromonas salmonicida are Gram-negative rod-shaped bacteria of about 1 × 1.5 μm in size, with cells arranged in bunches (Loch and Faisal 2010). They are facultative anaerobes whose optimum growth temperature ranges between 22 and 25 °C. A vast range of virulence factors allow rapid and efficient colonization of eukaryotic cells. These include an extracellular matrix of lipopolysaccharide (LPS) and a surface protein array (A-layer) which, respectively, enhance autoaggregation (Johnson et al. 1985) and adherence to eukaryotic cells (Garduño and Kay 1992). An arsenal of effector products are also secreted specifically to eukaryotic hosts by a molecular nanosyringe complex known as the type III secretion system (T3SS). The T3SS is the main virulence factor of A. salmonicida, and loss-of-function (LOF) mutations can drastically reduce virulence. Compared to the fully virulent wild-type (wt) strain JF5054, 2 × 105 times more CFU per fish of T3SS-negative mutants must be administered in order to achieve similar fish mortality (Vanden Bergh and Frey 2013). Genome sequencing of the reference strain A449 (Reith et al. 2008) has revealed major insights about other secretion routes such as the T2SS or T6SS. However, the T3SS genes are located on a large conjugative plasmid (pAsa5), while the others are on chromosomal loci. Previous studies have revealed that loss of virulence in A. salmonicida is due to thermolability of this plasmid (Stuber et al. 2003). High temperature (more than 25 °C) and stressful growth conditions lead to rearrangement of the T3SS-bearing plasmid by DNA recombination events between insertion sequences (IS) (Tanaka et al. 2012).
2.2 The Genus Flavobacterium
The genus Flavobacterium occurs in most aquatic ecosystems. Some species belonging to this genus are known as pathogenic agents of flavobacteriosis in fish. The four most important etiological agents of flavobacteriosis are F. psychrophilum, F. columnare, F. branchiophilum, and F. johnsoniae (Bernardet and Bowman 2006). Flavobacteriosis is a major problem in Scandinavian aquaculture, but it also affects fisheries worldwide (Decostere et al. 1998; Durborow et al. 1998; Madetoja et al. 2002; Bernardet and Bowman 2006; Mohamed and Ahmed Refat 2011). Flavobacterium sp. are naturally present in the fish microbiota. When the immune defenses of their host weaken, they undergo a positive shift in their virulence (Cahill 1990; Nematollahi et al. 2003; Austin 2006; Bernardet and Bowman 2006; Boutin et al. 2013b). Common symptoms of Flavobacteriosis include the erosion of external tissues of the fish (e.g., gills, fin, jaws, and tails). Evidence shows that Flavobacterium sp. is transmitted vertically as well as horizontally through water and physical contact (Bernardet and Bowman 2006).
2.2.1 Flavobacterium psychrophilum
Flavobacterium psychrophilum is the etiological agent of the (1) peduncle disease, the (2) cold-water disease, and the (3) rainbow trout fry syndrome. Those diseases occur mostly in salmonids, but its prevalence as a pathogen has also been demonstrated in other fish families (Amita et al. 2000; Liu et al. 2001). The symptoms of those diseases are characteristic lesions and necrosis occurring on, or near, the peduncle (Davis 1946); skin lesions located near the dorsal fin are associated in severe cases with a systemic infection causing anorexia, distended abdomen, and darkened pigmentation in the region of the caudal peduncle. In those severe cases, the bacterium may be isolated from internal organs such as the spleen and kidney. In rainbow trout fry syndrome, the several symptoms include lethargy and abnormal spiral swimming behavior. Subsequently, darkening of the gills, swelling of the abdomen, and reddening of the vent may be observed prior to death.
Flavobacterium psychrophilum are strictly aerobic, Gram-negative, slender, flexible rods. Their typical growth temperature ranges from 4 to 23 °C and is supported in water of low salinity but not high salinity. Strains of F. psychrophilum showed genetic heterogeneity with four serotypes identified in Japan, the United States, and Europe and the presence of diverse plasmids (Chakroun et al. 1998) (Wakabayashi et al. 1994; Lorenzen and Olesen 1997; Chakroun et al. 1998; Izumi et al. 2003). As the bacteria can be detected in sexual products from both female and male fish, parental transmission could likewise constitute a route of colonization (Holt 1993; Ekman et al. 1999; Amita et al. 2000; Vatsos et al. 2001, 2006; Cipriano 2005). This transmission route may lead to the development of disease in fry (Rangdale et al. 1996; Brown et al. 1997; Rangdale et al. 1997a, b; Kumagai et al. 2000; Cipriano 2005). Furthermore, F. psychrophilum is part of the natural microbiota, which makes fish a main reservoir for horizontal transmission (Bullock and Snieszko 1981; Holt 1993; Lorenzen 1994; Madetoja et al. 2002). Transmission via water streams is also demonstrated as a possible cross-contamination route—a claim that is supported by the ability of this bacterium to survive in streaming water and sediments for several months (Rangdale 1995; Brown et al. 1997; Madsen and Dalsgaard 1999; Vatsos et al. 2001; Madetoja et al. 2002; Sundell and Wiklund 2011).
However, even if F. psychrophilum is widely transmissible, the disease can occur only in immune-suppressed or stressed fish. Some studies proved that contact of the bacterium even at high concentrations is not sufficient to trigger an outbreak in healthy fish (Iida and Mizokami 1996; Ostland et al. 1997; Decostere et al. 2000). When the fish are stressed, the bacteria can outcompete the immune system and the natural microflora to invade the organisms via the gills, skin injuries, or gut (Lorenzen 1994; Liu et al. 2001).
2.2.2 Flavobacterium columnare
Flavobacterium columnare is an aerobic Gram-negative rod responsible for the columnaris disease. Phylogenetic analysis of the 16S rRNA gene has revealed strong heterogeneity among F. columnare strains. However, the high percentage of DNA–DNA hybridization further leads to a consideration of all those strains as being one species.
The virulence of this bacterium is strain dependent and is effective against a large range of host families, such as the Salmonidae, Cyprinidae, and Anguillidae. As is the case for many pathogens, a phylogenetic relationship between strain genotype and host species range is observed (Darwish and Ismaiel 2005). In fingerlings, death occurs early and no external lesions are observed. In the Salmonidae, specific lesions located near the dorsal fin have led to the naming of this condition as “saddleback disease.” Flavobacterium columnare is also an etiological agent of gill disease, along with F. branchiophilum. Symptoms of this disease include (1) white spots on the side of the head, the gills, and fins, (2) erosion of the mouth and fins, (3) necrosis, and (4) hemorrhagic lesions. In severe cases, the disease becomes systemic, and the bacteria colonize internal organs (Durborow et al. 1998). Stressful conditions have been shown to increase the probability of infections. Once fish are infected, they become a reservoir for the pathogen, which facilitates epizootic transmission of the disease. Presence of F. columnare favors colonization by other secondary pathogens like Saprolegnia. The disease is mostly transmitted via the surrounding waters and via moribund fish. Survival of F. columnare in water was estimated to be (1) 77 h in freshwater at 20 °C and (2) up to 16 days in alkaline water at 25 °C (Fijan 1967). The bacterial agent is able to colonize and infect fish without interindividual contact via the water column (Welker et al. 2005).
2.2.3 Flavobacterium branchiophilum
This bacterial species was first isolated from gills of salmonids in Japan, Ontario, and Oregon, as well as rainbow trout, sheatfish, and silver carp in Hungary. The etiological agent of gill disease is a Gram-negative rod which grows at temperatures ranging from 5 to 30 °C in low salinity (0–0.1 %).
Bacterial gill disease is one of the most important conditions affecting the salmonid aquaculture industry in Ontario (Ferguson et al. 1991; Turnbull 1993; Ostland et al. 1994). This disease is characterized by colonization of the gills at the lamellar surface by F. branchiophilum, which induces lamellar epithelial necrosis (Speare and Ferguson 1989; Speare et al. 1991). All the isolated strains so far are able to attach to the gills; however, only the most virulent ones colonize the gills substantially (Turnbull 1993; Ostland et al. 1995). Virulence of F. branchiophilum is not as pronounced as F. columnare or F. psychrophilum. Even in severe cases, the disease does not become systemic and usually does not invade the inner organs. In fact, the disease causes mortality only in juveniles (Kimura et al. 1978; Wakabayashi et al. 1980). The virulence of F. branchiophilum is attributed to various enzymatic and hemagglutinating activities detected in the extracellular products. Touchon et al. showed that the genome of F. branchiophilum presents the first observation of a cholera-like toxin gene acquired by a non-Proteobacteria (Touchon et al., 2011). Several adhesin-encoding genes are present, which may be linked to the virulence of the pathogen (Touchon et al. 2011). Few studies have focused on the transmission route of F. branchiophilum in aquaculture systems. Evidence suggests, however, that it probably occurs horizontally from the water supply. Indeed, bath exposure experiments reveal the presence of the pathogen in the water during outbreaks (Heo et al. 1990; Ostland et al. 1995).
2.3 The Genus Vibrio
The link between the genus Vibrio and pathogenicity dates from the beginning of modern microbiology. Several marine Vibrio, such as V. anguillarum, V. harveyi, and V. vulnificus, have proven to be potent pathogens of marine fish, migrating freshwater fish, bivalves, and crustaceans (Austin 2010). In an aquacultural context, high stock density facilitates epizootic transmission and augments the incidence of zoonoses affecting human consumers (Ghittino et al. 2003).
2.3.1 Vibrio anguillarum
The 1718’s outbreak of pestis rubra anguillarum (Lat. “red pest of eels”) in Italy was the first reference to a bacterial fish disease in the scientific literature (Bonaveri 1761 (quoted by Drouin de Bouville 1908)). However, it was not until the end of the nineteenth century that the causal agent of this disease was determined. Following an epizootic episode dating back to 1817 in migrating eels, Canestrini isolated the causal agent, which he named Bacterium anguillarum (1893). Sixteen years later, the etiological agent of the “red pest” in the Baltic Sea was described by Bergman (1909), who proposed the name Vibrio anguillarum from his findings. Subsequently, it was established that both researchers had described the same etiological agent, whose latter name has been retained. The disease, now generally referred to as “vibriosis,” causes fin and mouth rot, hemorrhages, generalized septicemia, and superficial skin lesions (Egidius 1987). Currently, it is widely known that vibriosis affects not only eels but also benthopelagic or benthic fish such as cod (Bagge and Bagge 1956), halibut (Hoare et al. 2002), and turbot (Grisez et al. 1996). Migrating freshwater fish, like Pacific and Atlantic salmon, also are susceptible targets of vibriosis (Evelyn 1971; Arkoosh et al. 1998; Frans et al. 2011). To a lesser extent, bivalves and crustaceans are occasionally infected (Paillard et al. 2004; Aguirre-Guzmán et al. 2004).
Vibrio anguillarum are Gram-negative, curved-rod-shaped bacteria. Their motility is guided by chemotaxis and mediated by a monotrichous polar flagellum (Larsen and Boesen 2001). Colonies growing on 2 % NaCl blood agar at 22 °C typically are yellowish, low, convex, and shiny and have unguent consistency and are sized from 3 to 5 mm after 48 h (Myhr et al. 1991).
The first pillar of bacterial virulence is proper recognition of the host. Vibrio anguillarum is not an exception and exhibits motility driven by chemotactic recognition of skin or gut mucus components (O’Toole et al. 1996, 1999). Active motility is required in the early stages of infection. A decrease in virulence and persistence in systemic infections is observed when the flagellin genes flaA, flaD, and flaE undergo deleterious mutations (Milton et al. 1996; McGee et al. 1996). Following movement toward the host, virulent bacteria “grip” to the host epithelial cells. In V. anguillarum, bacterial adherence is facilitated by a myriad of adherence factors (i.e., adhesins) such as fimbriae, outer membrane proteins (OMP), and extracellular polysaccharides (Pizarro-Cerdá and Cossart 2006). Invasion of host tissue is followed by degradation of epithelial mucus due to extracellular metalloprotease EmpA (Han et al. 2011). Once V. anguillarum has reached the blood vessels, the activity of hemolysins causes erythrocyte lysis and release of intracellular heme. Chelated iron ions are then captured by a siderophore-dependent iron acquisition system, which is encoded on pJM1, a ~65 kb plasmid (Stork et al. 2002).
3 Factors Triggering Opportunistic Infections
Examples of pathogenic outbreaks in aquaculture present some common trends:
-
Opportunistic pathogens seem to be present at low prevalence in healthy fish, indicating that under normal conditions, the immune system can maintain these organisms at low abundance.
-
Practices from aquaculture modify the environmental conditions that greatly impact the virulence or diversification of the pathogens (virus or bacteria).
In the following sections, we will explore the three main factors triggering opportunistic infections: (1) environmental factors, (2) relationships between pathogens and commensals, and (3) host–microbe interactions.
3.1 Environmental Factors
Fish welfare is known to be influenced by environmental parameters, as for all animals. There are now strong indications that circadian and seasonal variation of temperature and light can affect health (Zapata et al. 1992; Nelson 2004; Bowden et al. 2007). Such factors can affect fish susceptibility to infectious diseases due to increased prevalence of the pathogen or due to increased susceptibility in the host (Revie et al. 2002; Lillehaug et al. 2003; Hjelm et al. 2004; Ondračková et al. 2004). Temperature is one of the most influential environmental factors as fish are ectotherms and their inability to regulate their internal temperature influences their immune response (Baras 1995). For example, the columnaris disease occurs when temperature rises higher than 14 °C and most infections are observed between 20 and 30 °C (Durborow et al. 1998). Temperature may therefore influence both innate and adaptive immune responses in many different fish species (Bly and Clem 1992; Ellis 2001). Usually, higher temperature enhances the immune response in fish whereas lower temperature exerts an adverse effect (Bly and Clem 1992). Opportunistic diseases that appear at higher temperature are thus either due to (1) autoimmune damage triggering the infections or to (2) a direct effect on pathogen growth.
A second influential environmental parameter triggering opportunistic infections in fish is stress. The growing demand for fish is answered by the industry with extensive production, which often negatively affects the welfare of farmed fish (Ashley 2007). Based on health, physiological, and behavioral indicators, animal stress is a widely used indicator of welfare. Stress is a response reaction to a stimulus that can alter the homeostatic state of an individual (Barton and Iwama 1991). In aquaculture, stress results from handling, sorting, grading, transporting, and stocking. When these stress factors overwhelm the adaptive capacity of the fish, the stress response becomes detrimental to the fish’s health. Adams considered that stress can be divided into two different effects (Adams 1990):
-
Direct effects influence the organisms by altering their physiological, hormonal, or cellular functions.
-
Indirect effects operate at a higher level integrating the population or community effect by affecting the energy resources available for fish.
At the interface between direct and indirect effects, we can now append the effect of stress on the associated microbiome of fishes. Infectious diseases are known to be triggered by stress (Snieszko 1974; Wakabayashi 1991; Freestone et al. 2008; Littman et al. 2010; Boutin et al. 2012; Moloney et al. 2013). It is widely accepted that opportunistic pathogens, among which some are present in healthy fish microbiota, become infectious when hosts are stressed (Durborow et al. 1998; Le Moullac et al. 1998; Starliper 2011). However, it is nearly impossible to draw a global framework of how and when opportunistic diseases occur following stressful conditions. Fortunately, the number of studies on infectious outbreaks in aquaculture is growing. Recent studies highlight some patterns which increase the infectivity and spread of an infection, such as the number of host species cultured together and the intensity of culture.
Population density is the other factor that clearly influences the spread of the infection. In aquaculture settings, density is often a thousand times higher than in nature (Pulkkinen et al. 2010). This increases the probability of horizontal pathogen transmission through fish-to-fish contact. Indeed, host limitation (the frequency of host encounter) is one of the major limiting factors in pathogen population dynamics. The probability of transmission increases greatly when hosts are numerous and homogeneously distributed, especially if hosts are stressed by crowding or other factors (Snieszko 1974; Lipsitch et al. 1995; Ebert 1998; Pulkkinen et al. 2010). This disappearance of host range limitations also changes how the dynamics between hosts and pathogens evolve. The classical trade-off in this dynamics is that pathogens will reduce their virulence in order to avoid depletion of available hosts (Anderson and May 1982; Ebert and Mangin 1997). However, in aquaculture settings, frequency of host contact no longer is a limiting resource. Consequently, the removal of host encounter as a selection pressure may lead to increased virulence and transmission, resulting in increased pathogen fitness (Day 2002). A notable example illustrating this assumption is F. columnare. Virulence assays showed that F. columnare is not only able to survive in water and in carcasses but is also able to increase its dispersal rate. This results in more efficient transmission from dead to living fish, rather than between two living fish (Pulkkinen et al. 2010).
3.2 Microbe–Microbe Interactions
Interactions between microbial organisms including opportunistic pathogens in the environment as part of the host’s commensal microbiome can also influence disease outcome.
3.2.1 Horizontal Gene Transfer
High microbial density correlates to the occurrence of horizontal gene transfer (HGT), especially of resistance and virulence genes. HGT can occur by three different ways:
-
1.
Transformation, which is the acquisition of free DNA from the environment
-
2.
Transduction, involving transfer of genetic material via phage infection
-
3.
Conjugation, which is gene transfer through a type IV pilus connecting two cells (Sorensen et al. 2005)
Acquisition of antimicrobial resistance genes is mostly due to mutations, but the spreading of resistance is linked to HGT. The spread of virulence factors via HGT may potentially explain the emergence of some opportunistic pathogens.
3.2.2 Agonism and Antagonism
Different types of interactions occur between bacterial species. One of them, mutualism, consists of a beneficial relationship between one or more different individuals or species. In bacteria, mutualism can induce a specialization of certain taxa which efficiently produce select gene products consumed by the microbial community. Such mutualism in return favors the loss of genes by inefficient producers. Gene loss, leading to interdependency between taxa, is tentatively explained by the Black Queen Hypothesis (Morris et al. 2012). On the other hand, bacterial species also compete against each other. Indeed, competition is one of the most abundant interactions between not only bacteria but also most of living organisms (Begon et al. 1990). Patterns of agonism and antagonism explain most of the network of interactions that exist in the bacterial meta-community. Changes among these interactions that result in dysbiosis are also likely to trigger an opportunistic pathogen emergence.
3.2.3 Quorum Sensing
Microbes have developed a process to communicate between cells. This process, named quorum sensing (QS), changed the vision that bacteria regulate their biological processes independently. This system was first discovered in Aliivibrio fischeri, which expresses its bioluminescence in mid-exponential growth phase (Nealson et al. 1970). The signal molecule was found to be acyl-homoserine lactone (AHL). However, several other types of cell–cell communication signals have also been characterized in recent years: furanosyl borate (AI-2), cyclic thiolactone (AIP), hydroxyl palmitic acid methyl ester (PAME), methyl dodecanoic acid which acts as a diffusible signal factor (DSF), and farnesoic acid (FA) (Miller and Bassler 2001; Whitehead et al. 2001; Fuqua and Greenberg 2002). Quorum sensing is involved in the regulation of various biological processes, such as bioluminescence, antimicrobial compound production, plasmid conjugation, motility, virulence, and biofilm formation (Whitehead et al. 2001; Federle and Bassler 2003). It may also influence the emergence of opportunistic pathogens.
3.2.4 Quorum Quenching
As a response to this interspecies communication, some bacteria had developed a way to interfere to outcompete their rivals. This process, quorum quenching, consists of inhibiting the QS pathways by two different ways: (1) binding of non-signal molecules to the receptor (noncognate AHLs, intermediates of the AHL biosynthetic pathway, dicyclic peptides) or (2) degrading the QS signal with different enzymes (AHL-lactonases, decarboxylases, AHL-acylase, and deaminase) (Kalia 2013). Many opportunistic pathogens of fish have based their capacities to respond to environmental factors on QS. Indeed, Aeromonas hydrophila, A. salmonicida, Vibrio anguillarum, V. harveyi, and Yersinia ruckeri express their pathogenicity through QS systems (Bruhn et al. 2005).
3.3 Host–Microbe Interactions
Fish are in permanent interaction with microbes. This leads them to develop efficient processes to recognize potential partners. The most evolved system in that field is the immune system, based on molecular recognition of microbes. Recognition induces a signal which activates the effective immune functions. Two components of the immune system have emerged:
-
1.
The innate immune system, which deals with the recognition of conserved patterns, accessible and shared by many pathogens
-
2.
The adaptive immune response, based on receptors targeting specific structures of a given pathogen
3.3.1 Innate Immunity
The innate receptors are present on the periphery of all cells of the immune system (e.g., macrophages, dendritic cells). This process is important for the maintaining of homeostasis (i.e., stability in the structure of the commensal microbiome). To maintain homeostasis, receptors and signals of innate immunity are constitutively expressed by the host (Dixon et al. 2004). Innate immunity receptors are named pattern recognition receptors (PRR) (Akira et al. 2006). There are different types of those receptors: toll-like receptors (TLRs) and their coreceptor CD14, scavenger receptors, mannose receptors, integrins CD11b-c/CD18, and complement receptors CR1,2,3. Those cell surface receptors bind conserved structures of microbes named pathogen-associated molecular patterns (PAMPs) (Medzhitov and Janeway 1999). These surface molecules are specifically produced by bacteria as peptidoglycans (PGN), lipopolysaccharides (LPS), or lipoteichoic acid (LTA) and are not specific to pathogenic bacteria (Delneste et al. 2007).
3.3.2 Adaptive Immunity
The adaptive response will target specific pathogens via the activation of B and T cells. This response is characterized by immunological memory, which allows quicker recognition of the pathogen after a first infection. The B cells can recognize antigens in their native form. However, T cells recognize only those peptides which have been presented by the major histocompatibility complex (MHC), located at the surface of antigen-presenting cells.
Innate and adaptive immunity were considered as separate for a long time. However, in recent decades, studies have shown that both systems are interconnected, with innate immunity shaping the adaptive response (Medzhitov and Janeway 1999). Furthermore, the role of commensal bacteria was unknown. It is now acknowledged that commensal bacteria are involved in the maturation of both immune responses. Such bacteria even interfere with colonization of the host by pathogenic agents (Rakoff-Nahoum et al. 2004; Kelly et al. 2005; Mazmanian and Kasper 2006; O’Mahony et al. 2008). Protection against pathogens is then influenced by both host-mediated immunity and by the microbiome, but also by the dialog between those two components.
When the integrity of the microbiome is broken, this state is called dysbiosis. Stress on the host, changes in the environmental factors, or antibiotic use can disturb the natural microflora of fish (i.e., dysbiosis). This results in a weakening of the primary defense barrier of the host (Boutin et al. 2013b; Stecher et al. 2013). As we previously discussed, endogenous fish bacteria contribute to the immune function by such factors as colonization resistance (CR), immune response stimulation, and production of microbially inhibitory compounds (Dillon and Charnley 2002; Austin 2006; Ramsey and Whiteley 2009; Maslanik et al. 2012; Naik et al. 2012). The importance of commensal bacteria to fish health is starting to be understood. Furthermore, their role in disease prevention explains why probiotics are successful in aquaculture against many of the previously discussed fish pathogens (Moriarty 1998; Nikoskelainen et al. 2003; Farzanfar 2006; Nakayama et al. 2009; Nayak 2010; Burbank et al. 2011; Boutin et al. 2012, 2013a).
4 Prevention and Treatment of Opportunistic Infections
4.1 Antimicrobial Compounds
Aquaculture is a growing industry that encounters many issues with disease. The way to prevent and control those outbreaks was once exclusively the use of antimicrobial agents (Armstrong et al. 2005; Cole et al. 2009; Burridge et al. 2010). These chemicals were not only used to treat diseases but also as prophylaxis against bacterial infections. Beyond disease control, their side effects are exploited by the industry. For example, in the United States, 70 % of the annual production of antibiotics is used to increase the growth of the livestock (SCAN and the European Commission Health and Consumer Protection Directorate-General 2003; Balcazar et al. 2006). The extensive use of those chemicals in aquaculture leads to certain drawbacks for human and animal health (Witte 1998; Phillips et al. 2004; Marshall and Levy 2011). One of these is the persistence of antimicrobials in the environment, which induces a selective pressure on resistant bacteria. The results likely will include an increase in multiresistant microbial strains which could possibly be pathogenic for humans or livestock (Gold and Moellering 1996; Rangdale et al. 1997b; Levy 1998; Waldvogel 1999; Lindsay and Holden 2006; Arias and Murray 2009). In this context, the new methods being considered to prevent bacterial diseases are (1) the research of new antimicrobials acting upon secreted products of bacteria, instead of the cellular machinery itself (Chapra et al. 1997; Stephens and Shapiro 1997), and (2) alternative approaches such as vaccines, phages, probiotics, or genetic selection.
4.2 Vaccines
Vaccines exploit the adaptative immune response of fish (Foott and Hedrick 1987; Kent et al. 1999). Vaccines mimic a first infection by introducing antigens from the pathogens into the organism. Three types of inoculation are available in aquaculture: oral vaccination with antigen included in the food, immersion in diluted solution of the antigens, or intraperitoneal injection in the body cavity. Oral vaccination is seen as the easiest way to vaccinate fish because it does not require many efforts to administer. However, the decrease in fish mortality resulting from exposure to pathogens by this route is also significantly lower than what is traditionally associated with other methods, perhaps due to antigen destruction in the gut (Hart et al. 1988; Nakanishi and Ototake 1997; Sommerset et al. 2005). Vaccinations by immersion and by injection are the two main ways used so far in aquaculture. Vaccines for most viral and bacterial pathogens are available for salmonids and other fish species of significant economical value (Biering et al. 2004; Håstein et al. 2004). Vaccines have proven efficient in preventing many major bacterial diseases. However, for some pathogens, a single exposure to the vaccine is not enough to induce a long-term protection, and a second vaccination is needed during the production period. Most available virus vaccines, based on either inactivated virus or recombinant subunit proteins, are generally not efficient unless delivered by injection. Live viral vaccines have shown interesting results (Benmansour and De Kinkelin 1996) but are not yet considered safe for widespread use (Sommerset et al. 2005). Furthermore, the cost of developing new vaccines for virus and parasites is an important drawback to their commercial usage.
4.3 Phage Therapy
The use of bacteriophage as a therapy was developed as an alternative to antibiotics. As phage particles are very specific to their bacterial hosts, they do not target both pathogens and the normal flora, and thus, their use may minimize the chance of secondary infections following antibiotic-induced dysbiosis. Furthermore, phage particles replicate at the site of infection; thus, curative doses can be fairly small. Moreover, although bacteria can become resistant to phage, these viral organisms can mutate and therefore evolve to counter phage-resistant bacteria (Matsuzaki et al. 2005). In aquaculture, phage therapy is a new field, but the growing number of phage types isolated in the last decades is promising (Matsuzaki et al. 2005; Vinod et al. 2006; Shivu et al. 2007; Stenholm et al. 2008; Crothers‐Stomps et al. 2010; Defoirdt et al. 2011). The most important advantage of phage is that they might kill planktonic pathogens living in the surrounding water in addition to pathogens proliferating in carrier fish. Possible drawbacks of phage therapy include the possible transduction of virulence factors between bacteria, as well as the fact that the vertebrate host may mount an immune response against the phage itself.
4.4 Probiotics
The use of probiotics is probably the most widespread alternative to antibiotics so far. Probiotics are defined as a “live microbial culture added to feed or environment (water) to increase viability (survival) of the host” (Gram and Ringø 2005). This definition was recently modified by Merrifield et al. (2010) who defined a probiotic in aquaculture as:
A live, dead or component of a microbial cell that, when administered via the feed or to the rearing water, benefits the host by improving either disease resistance, health status, growth performance, feed utilisation, stress response, which is achieved at least in part via improving the hosts or the environmental microbial balance.
Although the mechanisms by which probiotics exert their beneficial effects require further investigation, probiotic administration showed promising results on growth performance and general health of teleost fish (Gatesoupe 2010). Despite the aforementioned advantages of probiotics, the viability of live bacteria during large-scale production of food (i.e., commercial diets) and during transition through the gastrointestinal tract is not always reliable (Ringø et al. 2014).
4.5 Prebiotics
To resolve the problem of viability, the prebiotic concept has been suggested and developed (Mahious and Ollevier 2005). A prebiotic is defined by Roberfroid (2007) as:
A non digestible food ingredient that beneficially affects the host by selectively stimulating the growth and/or activity of one or a limited number of bacteria in the colon, that can improve the host health.
To be classified as a prebiotic, a dietary ingredient needs to follow one of several different criteria: (1) resist gastric acidity, hydrolysis by digestive enzymes, and gastrointestinal absorption; (2) be fermented by the intestinal microbiota; and (3) be able to selectively stimulate the growth and activity of beneficial bacteria (Gibson 2004). First used in 1995 by Hanley, the number of prebiotics is increasing and includes many compounds, such as inulin and oligosaccharide compounds (Hanley et al. 1995; Ringø et al. 2014). Prebiotics are used as nutrients by probiotic bacteria (Geraylou et al. 2012). Many prebiotics are fermented by the gut microbiota into short-chain fatty acids (SCFA), which are the main energy source for colonic epithelial cells. Prebiotics are thus associated with maintenance of the epithelium (Cummings and Macfarlane 2002; Maslowski and Mackay 2010). The SCFA also modulate lipid synthesis (Marcil et al. 2002) as well as stimulate the immune system and aid with host resistance against pathogens (Maslowski and Mackay 2010).
4.6 Synbiotics
Synbiotics refer to nutritional complements combining probiotics and prebiotics (Cerezuela et al. 2011). Synbiotics aim to simultaneously seed and maintain probiotic strains as the dominant species in the gut after treatment cessation (Rurangwa et al. 2009). Despite recent progress in the field of synbiotics in aquaculture, there is limited information available on different aspects of synbiotic effects on fish (Cerezuela et al. 2011).
4.7 Selection Genetics
Selection of livestock to improve the growth and resistance is an old method used for centuries. However, selection programs in aquaculture represent a relatively recent field. Historically, the focus has been on fish growth rate. However, with the growing threat of infectious disease, pathogen resistance became a major phenotype for breeding programs. With the substantial advances in genetics, breeding programs are now based on genetic loci, which are quantitatively linked to the resistance (Baerwald et al. 2011; Langevin et al. 2012; Massault et al. 2011; Ozaki et al. 2001; Rodríguez-Ramilo et al. 2011; Verrier et al. 2013). These regions, named quantitative trait loci (QTL), are becoming interesting markers for the breeding program.
A new way assess the genetic basis of resistance is to observe QTL associated with the relationship between host and microbiome. There is evidence that QTL correlate with an influence of host genetic variation on fecal microbiome composition in mice (Benson et al. 2010; McKnite et al. 2012). Those taxa under host genetic control correspond with species and genera thought to interact with host immunity (Benson et al. 2010; McKnite et al. 2012). The QTL analysis of skin microbiome composition has recently been undertaken in the salmonid Salvelinus fontinalis (Boutin et al. 2014). Standing genetic variation among components of the teleost adaptive immune system is increasingly well characterized (Dionne et al. 2009; Pavey et al. 2013). While toll-like receptors (TLRs) are present in multiple teleost species (Palti 2011), there has been no work to date to correlate genetic diversity at these innate immune loci (inter- or intraspecies) with commensal microbiome diversity. Experiments in zebra fish highlight the role that TLRs play in modulating the intestinal microbiota, whereby alkaline phosphatase is produced via a TLR-4-myD88-controlled pathway to inhibit an inflammatory responses to gut microbiota (Bates et al. 2007). Given that desirable microbiome characteristics may exist from an aquaculture perspective (e.g., disease resistance, nutrient absorption, stress resilience), it is encouraging that a host genetic basis may exist for selection of such traits.
5 Conclusion
In this chapter, we have defined opportunistic teleost pathogens as pathogen species whose presence can be detected from apparently healthy teleost hosts. The status of these organisms—either as a natural component of a healthy commensal microbiome, or a latent step in disease establishment, or both—is still not entirely clear. In our discussion, we have limited ourselves to bacterial pathogens. We excluded viruses, which make up a significant proportion of known teleost pathogens, because these cannot safely be classed as opportunists owing to their obligate parasitic lifestyle. We outlined the major opportunist bacterial genera with special emphasis on disease in aquaculture. In doing so, we discussed the current understanding of bacterial pathogen taxonomy, biology, disease impact, and treatment options.
Unlike directly transmitted pathogens, understanding disease evolution and transmission caused by opportunistic pathogens necessitates a holistic view. Thus, in the second section of this chapter, we consider the importance of environment–host, pathogen–microbiome, and host–pathogen interactions in the context of opportunistic pathogens in teleosts. We demonstrate that not only do these factors play a crucial role in defining disease, but their importance opens up exciting new avenues to treat disease. As such, preventive measures to reduce stress, along with active interventions to enhance the protective effect of the microbiome (prebiotic, probiotics), can all mitigate the impact and prevalence of infectious disease.
The global demand for fish protein grows daily. Meanwhile, wild stocks are dwindling. Furthermore, anthropogenic activities have resulted in the epizootic dispersal of disease agents between farmed and wild individuals, as well as between wild populations via the introduction of invasive host species. A clear understanding of the drivers of disease caused by opportunistic pathogens is thus critical, not only to guarantee safe and sustainable aquaculture but also to protect existing wild fish species.
References
Abbott SL, Cheung WK, Kroske-Bystrom S et al (1992) Identification of Aeromonas strains to the genospecies level in the clinical laboratory. J Clin Microbiol 30:1262–1266
Adams SM (1990) Status and use of biological indicators for evaluating the effects of stress on fish. Am Fish Soc Symp 8:1–8
Aguirre-Guzmán G, Ruíz HM, Ascencio F (2004) A review of extracellular virulence product of Vibrio species important in diseases of cultivated shrimp. Aquacult Res 35:1395–1404. doi:10.1111/j.1365-2109.2004.01165.x
Akira S, Uematsu S, Takeuchi O (2006) Pathogen recognition and innate immunity. Cell 124:783–801. doi:10.1016/j.cell.2006.02.015
Amita K, Hoshino M, Honma T, Wakabayashi H (2000) An investigation on the distribution of Flavobacterium psychrophilum in the Umikawa River. Fish Pathol 35:193–197
Anderson RM, May RM (1982) Coevolution of hosts and parasites. Parasitology 85:411–426
Arias CA, Murray BE (2009) Antibiotic-resistant bugs in the 21st century—a clinical super-challenge. N Engl J Med 360:439–443. doi:10.1056/NEJMp0804651
Arkoosh MR, Casillas E, Huffman P et al (1998) Increased susceptibility of juvenile Chinook salmon from a contaminated estuary to Vibrio anguillarum. Trans Am Fish Soc 127:360–374. doi:10.1577/1548-8659(1998)127<0360:ISOJCS>2.0.CO;2
Armstrong SM, Hargrave BT, Haya K (2005) Antibiotic use in finfish aquaculture: modes of action, environmental fate, and microbial resistance. In: Hargrave BT (ed) Environmental effects of marine finfish aquaculture. Springer, Berlin, pp 341–357
Ashley PJ (2007) Fish welfare: current issues in aquaculture. Appl Anim Behav Sci 104:199–235
Austin B (2006) The bacterial microflora of fish, revised. ScientificWorldJournal 6:931–945. doi:10.1100/tsw.2006.181
Austin B (2010) Vibrios as causal agents of zoonoses. Vet Microbiol 140:310–317. doi:10.1016/j.vetmic.2009.03.015
Austin B, Austin DA (2007) Bacterial fish pathogens: diseases of farmed and wild fish. Praxis, Chichester
Austin B, Austin DA (2012a) Aeromonadaceae Representative (Aeromonas salmonicida). In: Austin B, Austin DA (eds) Bacterial fish pathogens. Springer, Dordrecht, pp 147–228
Austin B, Austin DA (2012b) Aeromonadaceae representatives (motile aeromonads). In: Austin B, Austin DA (eds) Bacterial fish pathogens. Springer, Dordrecht, pp 147–228
Baerwald MR, Petersen JL, Hedrick RP, Schisler GJ, May B (2011) A major effect quantitative trait locus for whirling disease resistance identified in rainbow trout (Oncorhynchus mykiss). Heredity 106:920–926
Bagge J, Bagge O (1956) Vibrio anguillarum som årsak til ulcus sykdom hos torsk (Gadus callarias Linné). Nord Vet Med 8:481–492
Balcazar J, Blas I, Ruizzarzuela I et al (2006) The role of probiotics in aquaculture. Vet Microbiol 114:173–186. doi:10.1016/j.vetmic.2006.01.009
Baras E (1995) Seasonal activities of Barbus barbus: effect of temperature on time-budgeting. J Fish Biol 46:806–818
Barton BA, Iwama GK (1991) Physiological changes in fish from stress in aquaculture with emphasis on the response and effects of corticosteroids. Annu Rev Fish Dis 1:3–26. doi:10.1016/0959-8030(91)90019-g
Bates JM, Akerlund J, Mittge E, Guillemin K (2007) Intestinal alkaline phosphatase detoxifies lipopolysaccharide and prevents inflammation in zebrafish in response to the gut microbiota. Cell Host Microbe 2:371–382. doi:10.1016/j.chom.2007.10.010
Begon M, Harper JL, Townsend CR (1990) Ecology: individuals populations and communities, 2nd edn. Blackwell, Oxford
Benmansour A, De Kinkelin P (1996) Live fish vaccines: history and perspectives. Dev Biol Stand 90:279–289
Benson AK, Kelly SA, Legge R et al (2010) Individuality in gut microbiota composition is a complex polygenic trait shaped by multiple environmental and host genetic factors. Proc Natl Acad Sci U S A 107:18933–18938. doi:10.1073/pnas.1007028107
Bergman AM (1909) Die rote Beulenkrankheit des Aals. Ber Aus K Bayer Vers 2:10–54
Bernardet J-F, Bowman J (2006) The genus Flavobacterium. In: Dworkin M, Falkow S, Rosenberg E et al (eds) The prokaryotes. Springer, New York, pp 481–531
Biering E, Villoing S, Sommerset I, Christie KE (2004) Update on viral vaccines for fish. Dev Biol 121:97–113
Bly JE, Clem LW (1992) Temperature and teleost immune functions. Fish Shellfish Immunol 2:159–171
Boutin S, Bernatchez L, Audet C, Derôme N (2012) Antagonistic effect of indigenous skin bacteria of brook charr (Salvelinus fontinalis) against Flavobacterium columnare and F. psychrophilum. Vet Microbiol 155:355–361. doi:10.1016/j.vetmic.2011.09.002
Boutin S, Audet C, Derôme N (2013a) Probiotic treatment by indigenous bacteria decreases mortality without disturbing the natural microbiota of Salvelinus fontinalis. Can J Microbiol 59:662–670
Boutin S, Bernatchez L, Audet C, Derôme N (2013b) Network analysis highlights complex interactions between pathogen, host and commensal microbiota. PLoS One 8:e84772. doi:10.1371/journal.pone.0084772
Boutin S, Sauvage C, Bernatchez L et al (2014) Inter individual variations of the fish skin microbiota: host genetics basis of mutualism? PLoS One 9:e102649. doi:10.1371/journal.pone.0102649
Bowden TJ, Thompson KD, Morgan AL et al (2007) Seasonal variation and the immune response: a fish perspective. Fish Shellfish Immunol 22:695–706
Brown LL, Cox WT, Levine RP (1997) Evidence that the causal agent of bacterial cold-water disease Flavobacterium psychrophilum is transmitted within salmonid eggs. Dis Aquat Organ 29:213–218
Bruhn JB, Dalsgaard I, Nielsen KF et al (2005) Quorum sensing signal molecules (acylated homoserine lactones) in gram-negative fish pathogenic bacteria. Dis Aquat Organ 65:43–52
Bullock GL, Snieszko SF (1981) Fin rot, coldwater disease, and peduncle disease of salmonid fishes. U.S. Fish and Wildlife Service, Fish Disease Leaflet No. 25
Burbank DR, Shah DH, LaPatra SE et al (2011) Enhanced resistance to coldwater disease following feeding of probiotic bacterial strains to rainbow trout (Oncorhynchus mykiss). Aquaculture 321:185–190. doi:10.1016/j.aquaculture.2011.09.004
Burridge L, Weis JS, Cabello F et al (2010) Chemical use in salmon aquaculture: a review of current practices and possible environmental effects. Aquaculture 306:7–23
Cahill MM (1990) Bacterial flora of fishes: a review. Microb Ecol 19:21–41. doi:10.1007/bf02015051
Canestrini G (1893) La malattia dominante delle anguille: richerche batteriologiche. Atti R Ist Veneto Sci Lett Ed Arti 7:809–814
Cerezuela R, Meseguer J, Esteban M (2011) Current knowledge in synbiotic use for fish aquaculture: a review. J Aquacult Res Dev 1:1–7
Chakroun C, Grimont F, Urdaci MC, Bernardet J-F (1998) Fingerprinting of Flavobacterium psychrophilum isolates by ribotyping and plasmid profiling. Dis Aquat Organ 33:167–177
Chapra I, Hodgson J, Metcalf B, Poste G (1997) The search for antimicrobial agents effective against bacteria resistant to multiple antibiotics. Antimicrob Agents Chemother 41:497–503
Cipriano RC (2005) Intraovum infection caused by Flavobacterium psychrophilum among eggs from captive Atlantic salmon broodfish. J Aquat Anim Health 17:275–283
Cipriano RC, Bullock GL (2001) Furunculosis and other diseases caused by Aeromonas salmonicida. United States Geological Survey
Cipriano R, Bullock G, Pyle S (1984) Aeromonas hydrophila and other septicemias of fish. U.S. Fish and Wildlife Service Publications
Coburn B, Sekirov I, Finlay BB (2007) Type III secretion systems and disease. Clin Microbiol Rev 20:535–549. doi:10.1128/CMR.00013-07
Cole DW, Cole R, Gaydos SJ et al (2009) Aquaculture: environmental, toxicological, and health issues. Int J Hyg Environ Health 212:369–377
Crothers‐Stomps C, Høj L, Bourne DG et al (2010) Isolation of lytic bacteriophage against Vibrio harveyi. J Appl Microbiol 108:1744–1750
Cummings JH, Macfarlane GT (2002) Gastrointestinal effects of prebiotics. Br J Nutr 87:S145–S151
Darwish AM, Ismaiel AA (2005) Genetic diversity of Flavobacterium columnare examined by restriction fragment length polymorphism and sequencing of the 16S ribosomal RNA gene and the 16S–23S rDNA spacer. Mol Cell Probes 19:267–274
Davis HS (1946) Care and diseases of trout. US Department of Interior Research Report No. 12
Day T (2002) Virulence evolution via host exploitation and toxin production in spore-producing pathogens. Ecol Lett 5:471–476
Decostere A, Haesebrouck F, Devriese LA (1998) Characterization of four Flavobacterium columnare (Flexibacter columnaris) strains isolated from tropical fish. Vet Microbiol 62:35–45. doi:10.1016/s0378-1135(98)00196-5
Decostere A, Lammens M, Haesebrouck F (2000) Difficulties in experimental infection studies with Flavobacterium psychrophilum in rainbow trout (Oncorhynchus mykiss) using immersion, oral and anal challenges. Res Vet Sci 69:165–169
Defoirdt T, Sorgeloos P, Bossier P (2011) Alternatives to antibiotics for the control of bacterial disease in aquaculture. Curr Opin Microbiol 14:251–258, http://dx.doi.org/10.1016/j.mib.2011.03.004
Delneste Y, Beauvillain C, Jeannin P (2007) Immunité naturelle: Structure et fonction des toll-like receptors. Med Paris 23:67–74
Dillon R, Charnley K (2002) Mutualism between the desert locust Schistocerca gregaria and its gut microbiota. Res Microbiol 153:503–509, http://dx.doi.org/10.1016/S0923-2508(02)01361-X
Dionne M, Miller KM, Dodson JJ, Bernatchez L (2009) MHC standing genetic variation and pathogen resistance in wild Atlantic salmon. Philos Trans R Soc Lond B Biol Sci 364:1555–1565. doi:10.1098/rstb.2009.0011
Dixon DR, Bainbridge BW, Darveau RP (2004) Modulation of the innate immune response within the periodontium. Periodontol 2000 35:53–74. doi:10.1111/j.0906-6713.2004.003556.x
Drouin de Bouville R de (1908) Les maladies des poissons d’eau douce d’Europe: d’après les travaux des divers icthyo-pathologistes et le traité du professeur Hofer (Deuxième édition, revue et augmentée)/par R. de Drouin de Bouville,… Berger-Levrault (Paris)
Durborow RM, Thune RL, Hawke JP, Camus AC (1998) Columnaris disease: a bacterial infection caused by Flavobacterium columnare. Southern Regional Aquaculture Center (SRAC) Publication No. 479
Ebert D (1998) Experimental evolution of parasites. Science 282:1432–1436
Ebert D, Mangin KL (1997) The influence of host demography on the evolution of virulence of a microsporidian gut parasite. Evolution 51:1828–1837
Egidius E (1987) Vibriosis: pathogenicity and pathology. A review. Aquaculture 67:15–28. doi:10.1016/0044-8486(87)90004-4
Ekman E, Börjeson H, Johansson N (1999) Flavobacterium psychrophilum in Baltic salmon Salmo salar brood fish and their offspring. Dis Aquat Organ 37:159–163
Ellis AE (2001) Innate host defense mechanisms of fish against viruses and bacteria. Dev Comp Immunol 25:827–839. doi:10.1016/s0145-305x(01)00038-6
Emmerich R, Weibel F (1890) Über eine durch Bakterien verursachte Infektionskrankheit der Forellen. Allg Fisch-Ztg 15:73–77, 85–92
Evelyn TPT (1971) First records of vibriosis in pacific salmon cultured in Canada, and taxonomic status of the responsible bacterium, Vibrio anguillarum. J Fish Res Board Can 28:517–525. doi:10.1139/f71-073
Farzanfar A (2006) The use of probiotics in shrimp aquaculture. FEMS Immunol Med Microbiol 48:149–158. doi:10.1111/j.1574-695X.2006.00116.x
Federle MJ, Bassler BL (2003) Interspecies communication in bacteria. J Clin Invest 112:1291–1299
Ferguson HW, Ostland VE, Byrne P, Lumsdsen JS (1991) Experimental production of bacterial gill disease in trout by horizontal transmission and by bath challenge. J Aquat Anim Health 3:118–123
Fijan N (1967) The survival of Chondrococcus columnaris in waters of different quality. Bull Off Int Epizoot 69:1159–1166
Foott JS, Hedrick RP (1987) Seasonal occurrence of the infectious stage of proliferative kidney disease (PKD) and resistance of rainbow trout, Salmo gairdneri Richardson, to reinfection. J Fish Biol 30:477–483
Frank DN, Zhu W, Sartor RB, Li E (2011) Investigating the biological and clinical significance of human dysbioses. Trends Microbiol 19:427–434. doi:10.1016/j.tim.2011.06.005
Frans I, Michiels CW, Bossier P et al (2011) Vibrio anguillarum as a fish pathogen: virulence factors, diagnosis and prevention. J Fish Dis 34:643–661. doi:10.1111/j.1365-2761.2011.01279.x
Freestone PP, Sandrini SM, Haigh RD, Lyte M (2008) Microbial endocrinology: how stress influences susceptibility to infection. Trends Microbiol 16:55–64
Fuqua C, Greenberg EP (2002) Listening in on bacteria: acyl-homoserine lactone signalling. Nat Rev Mol Cell Biol 3:685–695
Garduño RA, Kay WW (1992) Interaction of the fish pathogen Aeromonas salmonicida with rainbow trout macrophages. Infect Immun 60:4612–4620
Gatesoupe FJ (2010) Probiotics and other microbial manipulations in fish feeds: prospective health benefits. In: Watson RR, Preedy VR (eds) Bioactive foods in promoting health. Probiotics and prebiotics. Academic, San Diego, CA, pp 541–552
Geraylou Z, Souffreau C, Rurangwa E et al (2012) Effects of arabinoxylan-oligosaccharides (AXOS) on juvenile Siberian sturgeon (Acipenser baerii) performance, immune responses and gastrointestinal microbial community. Fish Shellfish Immunol 33:718–724. doi:10.1016/j.fsi.2012.06.010
Ghittino C, Latini M, Agnetti F et al (2003) Emerging pathologies in aquaculture: effects on production and food safety. Vet Res Commun 27:471–479. doi:10.1023/B:VERC.0000014204.37722.b6
Gibson GR (2004) Fibre and effects on probiotics (the prebiotic concept). Clin Nutr Suppl 1:25–31
Gold HS, Moellering RC (1996) Antimicrobial-drug resistance. N Engl J Med 335:144–1453
Gram L, Ringø E (2005) Prospects of fish probiotics. In: Holzapfel WH, Naughton PJ (eds) Microbial ecology of the growing animal. Elsevier, Amsterdam, pp 379–417
Grisez L, Chair M, Sorgeloos P, Ollevier F (1996) Mode of infection and spread of Vibrio anguillarum in turbot Scophthalmus maximus larvae after oral challenge through live feed. Dis Aquat Organ 26:181–187
Han Y, Mo Z, Xiao P et al (2011) Characterization of EmpA protease in Vibrio anguillarum M3. J Ocean Univ Chin 10:379–384. doi:10.1007/s11802-011-1781-x
Hanley F, Brown H, Carberry J (1995) First observations on the effects of mannan oligosaccharide added to the hatchery diets for warmwater Hybrid Red Tilapia. In: Nutritional biotechnology in the feed and food industries. Proceedings of Alltech’s 11th annual symposium, Lexington, KY
Hart S, Wrathmell AB, Harris JE, Grayson TH (1988) Gut immunology in fish: a review. Dev Comp Immunol 12:453–480
Håstein T, Gudding R, Evensen O (2004) Bacterial vaccines for fish—an update of the current situation worldwide. Dev Biol 121:55–74
Heo G-J, Kasai K, Wakabayashi H (1990) Occurrence of Flavobacterium branchiophila associated with bacterial gill disease at a trout hatchery. Fish Pathol 25:99–105
Hjelm M, Riaza A, Formoso F et al (2004) Seasonal incidence of autochthonous antagonistic Roseobacter spp. and Vibrionaceae strains in a turbot larva (Scophthalmus maximus) rearing system. Appl Environ Microbiol 70:7288–7294. doi:10.1128/aem.70.12.7288-7294.2004
Hoare R, Hovland H, Langston AL et al (2002) Susceptibility of three different strains of juvenile Atlantic halibut (Hippoglossus hippoglossus L.) cultured at two different temperatures to Vibrio anguillarum and temperature effect on antibody response. Fish Shellfish Immunol 13:111–123
Holt RA, Rohovec JS, Fryer JL (1993) Bacterial coldwater disease. In: Inglis V, Roberts RJ, Bromage NR (eds) Bacterial disease of fish. Blackwell, Oxford, pp 3–22
Horneman AJ, Ali A, Abbott SL (2007) Aeromonas. In: Murray PR, Baron EJ, Landry ML, Jorgensen JH, Pfaller MA (eds) Manual of clinical microbiology, 9th edn. ASM, Washington, DC, pp 715–722
Iida Y, Mizokami A (1996) Outbreaks of coldwater disease in wild Ayu and Pale Chub. Fish Pathol 31:157–164. doi:10.3147/jsfp.31.157
Izumi S, Liu H, Aranishi F, Wakabayashi H (2003) A novel serotype of Flavobacterium psychrophilum detected using antiserum against an isolate from amago, Oncorhynchus masou rhodurus Jordan & Gilbert, in Japan. J Fish Dis 26:677–680. doi:10.1046/j.1365-2761.2003.00502.x
Janda JM, Abbott SL (2010) The genus Aeromonas: taxonomy, pathogenicity, and infection. Clin Microbiol Rev 23:35–73. doi:10.1128/CMR.00039-09
Janda JM, Duffey PS (1988) Mesophilic aeromonads in human disease: current taxonomy, laboratory identification, and infectious disease spectrum. Rev Infect Dis 10:980–997
Johnson CM, Tatner MF, Horne MT (1985) Autoaggregation and extracellular A-layer protein in Aeromonas salmonicida. Aquaculture 46:163–166. doi:10.1016/0044-8486(85)90200-5
Kalia VC (2013) Quorum sensing inhibitors: an overview. Biotechnol Adv 31:224–245
Kelly D, Conway S, Aminov R (2005) Commensal gut bacteria: mechanisms of immune modulation. Trends Immunol 26:326–333. doi:10.1016/j.it.2005.04.008
Kent ML, Dawe SC, Speare DJ (1999) Resistance to reinfection in chinook salmon Oncorhynchus tshawytscha to Loma salmonae (Microsporidia). Dis Aquat Organ 37:205
Kimura N, Wakabayashi H, Kudo S (1978) Studies on bacterial gill disease in salmonids, 1: Selection of bacterium transmitting gill disease. Fish Pathol 12:233–242
Kumagai A, Yamaoka S, Takahashi K et al (2000) Waterborne transmission of Flavobacterium psychrophilum in Coho salmon eggs. Gyobyo Kenkyu Fish Pathol 35:25–28
Langevin C, Blanco M, Martin SAM, Jouneau L, Bernardet J-F, Houel A, Lunazzi A, Duchaud E, Michel C, Quillet E, Boudinot P (2012) Transcriptional responses of resistant and susceptible fish clones to the bacterial pathogen Flavobacterium psychrophilum. PLoS One 7, e39126. doi:10.1371/journal.pone.0039126
Larsen MH, Boesen HT (2001) Role of flagellum and chemotactic motility of Vibrio anguillarum for phagocytosis by and intracellular survival in fish macrophages. FEMS Microbiol Lett 203:149–152. doi:10.1111/j.1574-6968.2001.tb10833.x
Le Moullac G, Soyez C, Saulnier D, Ansquer D, Avarre JC, Levy P (1998) Effect of hypoxic stress on the immune response and the resistance to vibriosis of the shrimp Penaeus stylirostris. Fish Shellfish Immunol 8:621–629. doi:10.1006/fsim.1998.0166
Levy SB (1998) Multidrug resistance—a sign of the times. N Engl J Med 338:1376–1378
Lillehaug A, Lunestad BT, Grave K (2003) Epidemiology of bacterial diseases in Norwegian aquaculture—a description based on antibiotic prescription data for the ten-year period 1991 to 2000. Dis Aquat Organ 53:115–125
Lindsay J, Holden M (2006) Understanding the rise of the superbug: investigation of the evolution and genomic variation of Staphylococcus aureus. Funct Integr Genomics 6:186–201. doi:10.1007/s10142-005-0019-7
Lipsitch M, Nowak MA, Ebert D, May RM (1995) The population dynamics of vertically and horizontally-transmitted parasites. Proc Biol Sci 260:321–327. doi:10.1098/rspb.1995.0099
Littman RA, Bourne DG, Willis BL (2010) Responses of coral-associated bacterial communities to heat stress differ with Symbiodinium type on the same coral host. Mol Ecol 19:1978–1990. doi:10.1111/j.1365-294X.2010.04620.x
Liu H, Izumi S, Wakabayashi H (2001) Detection of Flavobacterium psychrophilum in various organs of ayu Plecoglossus altivelis by in situ hybridization. Fish Pathol 36:7–11
Loch TP, Faisal M (2010) Isolation of Aeromonas salmonicida subspecies salmonicida from Lake Whitefish (Coregonus clupeaformis) inhabiting Lakes Michigan and Huron. J Great Lakes Res 36(Suppl 1):13–17. doi:10.1016/j.jglr.2009.07.002
Lorenzen E (1994) Studies on Flexibacter psychrophilus in relation to rainbow trout fry syndrome (RTFS). Royal Veterinary and Agricultural University, Copenhagen
Lorenzen E, Olesen NJ (1997) Characterization of isolates of Flavobacterium psychrophilum associated with coldwater disease or rainbow trout fry syndrome II: serological studies. Dis Aquat Organ 31:209–220. doi:10.3354/dao031209
Lowry T, Smith SA (2007) Aquatic zoonoses associated with food, bait, ornamental, and tropical fish. J Am Vet Med Assoc 231:876–880. doi:10.2460/javma.231.6.876
Madetoja J, Dalsgaard I, Wiklund T (2002) Occurrence of Flavobacterium psychrophilum in fish-farming environments. Dis Aquat Organ 52:109–118
Madsen L, Dalsgaard I (1999) Reproducible methods for experimental infection with Flavobacterium psychrophilum in rainbow trout Oncorhynchus mykiss. Dis Aquat Organ 36:169
Mahious A, Ollevier F (2005) Probiotics and prebiotics in aquaculture. In: 1st Regional workshop on techniques for enrichment for use in larviculture. Urmia, Iran
Marcil V, Delvin E, Seidman E et al (2002) Modulation of lipid synthesis, apolipoprotein biogenesis, and lipoprotein assembly by butyrate. Am J Physiol Gastrointest Liver Physiol 283:G340–G346
Marshall BM, Levy SB (2011) Food animals and antimicrobials: impacts on human health. Clin Microbiol Rev 24:718–733
Maslanik T, Tannura K, Mahaffey L et al (2012) Commensal bacteria and MAMPs are necessary for stress-induced increases in IL-1β and IL-18 but not IL-6, IL-10 or MCP-1. PLoS One 7:e50636
Maslowski KM, Mackay CR (2010) Diet, gut microbiota and immune responses. Nat Immunol 12:5–9
Massault C, Franch R, Haley C, De Koning DJ, Bovenhuis H, Pellizzari C, Patarnello T, Bargelloni L (2011) Quantitative trait loci for resistance to fish pasteurellosis in gilthead sea bream (Sparus aurata). Anim Genet 42:191–203
Matsuzaki S, Rashel M, Uchiyama J et al (2005) Bacteriophage therapy: a revitalized therapy against bacterial infectious diseases. J Infect Chemother 11:211–219
Mazmanian SK, Kasper DL (2006) The love-hate relationship between bacterial polysaccharides and the host immune system. Nat Rev Immunol 6:849–858
McGee K, Horstedt P, Milton DL (1996) Identification and characterization of additional flagellin genes from Vibrio anguillarum. J Bacteriol 178:5188–5198
McKnite AM, Perez-Munoz ME, Lu L et al (2012) Murine gut microbiota is defined by host genetics and modulates variation of metabolic traits. PLoS One 7:e39191. doi:10.1371/journal.pone.0039191
Medzhitov R, Janeway CA (1999) Innate immune induction of the adaptive immune response. Cold Spring Harb Symp Quant Biol 64:429–436. doi:10.1101/sqb.1999.64.429
Merrifield DL, Dimitroglou A, Foey A et al (2010) The current status and future focus of probiotic and prebiotic applications for salmonids. Aquaculture 302:1–18
Miller MB, Bassler BL (2001) Quorum sensing in bacteria. Annu Rev Microbiol 55:165–199
Milton DL, O’Toole R, Horstedt P, Wolf-Watz H (1996) Flagellin A is essential for the virulence of Vibrio anguillarum. J Bacteriol 178:1310–1319
Mohamed MH, Ahmed Refat NAG (2011) Pathological evaluation of probiotic, Bacillus subtilis, against Flavobacterium columnare in tilapia nilotica (Oreochromis niloticus) fish in Sharkia governorate, Egypt. J Am Sci 7:244–256
Moloney R, Desbonnet L, Clarke G et al (2013) The microbiome: stress, health and disease. Mamm Genome 25:49–74. doi:10.1007/s00335-013-9488-5
Moriarty DJW (1998) Control of luminous Vibrio species in penaeid aquaculture ponds. Aquaculture 164:351–358. doi:10.1016/s0044-8486(98)00199-9
Morin R (2010) L’utilisation des antibiotiques pour combattre la furonculose chez l’omble de fontaine génère de l’antibiorésistance chez Aeromonas salmonicida. Bull L’Association Aquaculteurs Qué 15:3–6
Morris JJ, Lenski RE, Zinser ER (2012) The black queen hypothesis: evolution of dependencies through adaptive gene loss. mBio. doi:10.1128/mBio.00036-12
Myhr E, Larsen JL, Lillehaug A et al (1991) Characterization of Vibrio anguillarum and closely related species isolated from farmed fish in Norway. Appl Environ Microbiol 57:2750–2757
Naik S, Bouladoux N, Wilhelm C et al (2012) Compartmentalized control of skin immunity by resident commensals. Science 337:1115–1119. doi:10.1126/science.1225152
Nakanishi T, Ototake M (1997) Antigen uptake and immune responses after immersion vaccination. Dev Biol Stand 90:59
Nakayama T, Lu H, Nomura N (2009) Inhibitory effects of Bacillus probionts on growth and toxin production of Vibrio harveyi pathogens of shrimp. Lett Appl Microbiol 49:679–684. doi:10.1111/j.1472-765X.2009.02725.x
Nayak SK (2010) Probiotics and immunity: a fish perspective. Fish Shellfish Immunol 29:2–14. doi:10.1016/j.fsi.2010.02.017
Nealson KH, Platt T, Hastings JW (1970) Cellular control of the synthesis and activity of the bacterial luminescent system. J Bacteriol 104:313–322
Nelson RJ (2004) Seasonal immune function and sickness responses. Trends Immunol 25:187–192
Nematollahi A, Decostere A, Pasmans F, Haesebrouck F (2003) Flavobacterium psychrophilum infections in salmonid fish. J Fish Dis 26:563–574. doi:10.1046/j.1365-2761.2003.00488.x
Nielsen ME, Høi L, Schmidt AS et al (2001) Is Aeromonas hydrophila the dominant motile Aeromonas species that causes disease outbreaks in aquaculture production in the Zhejiang Province of China? Dis Aquat Organ 46:23–29. doi:10.3354/dao046023
Nikoskelainen S, Ouwehand AC, Bylund G et al (2003) Immune enhancement in rainbow trout (Oncorhynchus mykiss) by potential probiotic bacteria (Lactobacillus rhamnosus). Fish Shellfish Immunol 15:443–452. doi:10.1016/s1050-4648(03)00023-8
O’Mahony C, Scully P, O’Mahony D et al (2008) Commensal-induced regulatory T cells mediate protection against pathogen-stimulated NF-κB activation. PLoS Pathog 4:e1000112. doi:10.1371/journal.ppat.1000112
O’Toole R, Milton DL, Wolf-Watz H (1996) Chemotactic motility is required for invasion of the host by the fish pathogen Vibrio anguillarum. Mol Microbiol 19:625–637
O’Toole R, Lundberg S, Fredriksson SA et al (1999) The chemotactic response of Vibrio anguillarum to fish intestinal mucus is mediated by a combination of multiple mucus components. J Bacteriol 181:4308–4317
Ondračková M, Reichard M, Jurajda P, Gelnar M (2004) Seasonal dynamics of Posthodiplostomum cuticola (Digenea, Diplostomatidae) metacercariae and parasite-enhanced growth of juvenile host fish. Parasitol Res 93:131–136
Ostland VE, Lumsden JS, MacPhee DD, Ferguson HW (1994) Characteristics of Flavobacterium branchiophilum, the cause of salmonid bacterial gill disease in Ontario. J Aquat Anim Health 6:13–26
Ostland VE, MacPhee DD, Lumsden JS, Ferguson HW (1995) Virulence of Flavobacterium branchiophilum in experimentally infected salmonids. J Fish Dis 18:249–262. doi:10.1111/j.1365-2761.1995.tb00300.x
Ostland VE, McGrogan DG, Ferguson HW (1997) Cephalic osteochondritis and necrotic scleritis in intensively reared salmonids associated with Flexibacter psychrophilus. J Fish Dis 20:443–451. doi:10.1046/j.1365-2761.1997.00323.x
Ozaki A, Sakamoto T, Khoo S, Nakamura K, Coimbra MR, Akutsu T, Okamoto N (2001) Quantitative trait loci (QTLs) associated with resistance/susceptibility to infectious pancreatic necrosis virus (IPNV) in rainbow trout (Oncorhynchus mykiss). Mol Genet Genomics 265:23–31
Paillard C, Le Roux F, Borrego JJ (2004) Bacterial disease in marine bivalves, a review of recent studies: trends and evolution. Aquat Living Resour 17:477–498. doi:10.1051/alr:2004054
Palti Y (2011) Toll-like receptors in bony fish: from genomics to function. Dev Comp Immunol 35:1263–1272. doi:10.1016/j.dci.2011.03.006
Pavey SA, Sevellec M, Adam W et al (2013) Nonparallelism in MHCIIbeta diversity accompanies nonparallelism in pathogen infection of lake whitefish (Coregonus clupeaformis) species pairs as revealed by next-generation sequencing. Mol Ecol 22:3833–3849. doi:10.1111/mec.12358
Phillips I, Casewell M, Cox T et al (2004) Does the use of antibiotics in food animals pose a risk to human health? A critical review of published data. J Antimicrob Chemother 53:28–52. doi:10.1093/jac/dkg483
Pizarro-Cerdá J, Cossart P (2006) Bacterial adhesion and entry into host cells. Cell 124:715–727. doi:10.1016/j.cell.2006.02.012
Plumb JA, Grizzle JM, Defigueiredo J (1976) Necrosis and bacterial infection in channel catfish (Ictalurus punctatus) following hypoxia. J Wildl Dis 12:247–253
Pulkkinen K, Suomalainen L-R, Read AF et al (2010) Intensive fish farming and the evolution of pathogen virulence: the case of columnaris disease in Finland. Proc Biol Sci 277:593–600. doi:10.1098/rspb.2009.1659
Rakoff-Nahoum S, Paglino J, Eslami-Varzaneh F et al (2004) Recognition of commensal microflora by toll-like receptors is required for intestinal homeostasis. Cell 118:229–241. doi:10.1016/j.cell.2004.07.002
Ramsey MM, Whiteley M (2009) Polymicrobial interactions stimulate resistance to host innate immunity through metabolite perception. Proc Natl Acad Sci USA 106:1578–1583. doi:10.1073/pnas.0809533106
Rangdale RE (1995) Studies on rainbow trout fry syndrome (RTFS). PhD thesis, University of Stirling, Stirling
Rangdale RE, Richards RE, Alderman DJ (1996) Isolation of Cytophaga psychrophila, causal agent of rainbow trout fry syndrome (RTFS) from reproductive fluids and egg surfaces of rainbow trout (Oncorhynchus mykiss). Bull Eur Assoc Fish Pathol 16:63–67
Rangdale RE, Richards RH, Alderman DJ (1997a) Colonisation of eyed rainbow trout ova with Flavobacterium psychrophilum leads to rainbow trout fry syndrome in fry. Bull Eur Assoc Fish Pathol 17:108–111
Rangdale RE, Richards RH, Alderman DJ (1997b) Minimum inhibitory concentrations of selected antimicrobial compounds against Flavobacterium psychrophilum the causal agent of rainbow trout fry syndrome (RTFS). Aquaculture 158:193–201. doi:10.1016/s0044-8486(97)00202-0
Reith ME, Singh RK, Curtis B et al (2008) The genome of Aeromonas salmonicida subsp. salmonicida A449: insights into the evolution of a fish pathogen. BMC Genomics 9:427. doi:10.1186/1471-2164-9-427
Revie CW, Gettinby G, Treasurer JW et al (2002) Temporal, environmental and management factors influencing the epidemiological patterns of sea lice (Lepeophtheirus salmonis) infestations on farmed Atlantic salmon (Salmo salar) in Scotland. Pest Manag Sci 58:576–584
Ringø E, Dimitroglou A, Hoseinifar SH, Davies SJ (2014) Prebiotics in finfish: an update. In: Merrifield DL, Ringø E (eds) Aquaculture nutrition: gut health, probiotics and prebiotics. Wiley-Blackwell, Oxford, pp 410–418
Roberfroid M (2007) Prebiotics: the concept revisited. J Nutr 137:830S–837S
Rodríguez-Ramilo ST, Toro MA, Bouza C, Hermida M, Pardo BG, Cabaleiro S, Martínez P, Fernández J (2011) QTL detection for Aeromonas salmonicida resistance related traits in turbot (Scophthalmus maximus). BMC Genomics 12:541. doi:10.1186/1471-2164-12-541
Rurangwa E, Laranja JL, Van Houdt R et al (2009) Selected nondigestible carbohydrates and prebiotics support the growth of probiotic fish bacteria mono cultures in vitro. J Appl Microbiol 106:932–940
Sara M, Sleytr UB (2000) S-layer proteins. J Bacteriol 182:859–868
SCAN, European Commission Health and Consumer Protection Directorate-General (2003) Opinion of the scientific committee on Animal Nutrition on the criteria for assessing the safety of microorganisms resistant to antibiotics of human clinical and veterinary importance
Scott M (1968) The pathogenicity of Aeromonas salmonicida (Griffin) in sea and brackish waters. J Gen Microbiol 50:321–327. doi:10.1099/00221287-50-2-321
Shivu MM, Rajeeva BC, Girisha SK et al (2007) Molecular characterization of Vibrio harveyi bacteriophages isolated from aquaculture environments along the coast of India. Environ Microbiol 9:322–331
Snieszko SF (1974) The effects of environmental stress on outbreaks of infectious diseases of fishes. J Fish Biol 6:197–208. doi:10.1111/j.1095-8649.1974.tb04537.x
Sommerset I, Krossøy B, Biering E, Frost P (2005) Vaccines for fish in aquaculture. Expert Rev Vaccines 4:89–101. doi:10.1586/14760584.4.1.89
Sorensen SJ, Bailey M, Hansen LH et al (2005) Studying plasmid horizontal transfer in situ: a critical review. Nat Rev Microbiol 3:700–710
Speare DJ, Ferguson HW (1989) Clinical and pathological features of common gill diseases of cultured salmonids in Ontario. Can Vet J 30:882
Speare DJ, Ferguson HW, Beamish FWM et al (1991) Pathology of bacterial gill disease: ultrastructure of branchial lesions. J Fish Dis 14:1–20
Starliper CE (2011) Bacterial coldwater disease of fishes caused by Flavobacterium psychrophilum. J Adv Res 2:97–108. doi:10.1016/j.jare.2010.04.001
Stecher B, Maier L, Hardt W-D (2013) “Blooming” in the gut: how dysbiosis might contribute to pathogen evolution. Nat Rev Microbiol 11:277–284. doi:10.1038/nrmicro2989
Stenholm AR, Dalsgaard I, Middelboe M (2008) Isolation and characterization of bacteriophages infecting the fish pathogen Flavobacterium psychrophilum. Appl Environ Microbiol 74:4070–4078
Stephens C, Shapiro L (1997) Bacterial protein secretion—a target for new antibiotics? Chem Biol 4:637–641
Stork M, Di Lorenzo M, Welch TJ et al (2002) Plasmid-mediated iron uptake and virulence in Vibrio anguillarum. Plasmid 48:222–228
Stuber K, Burr SE, Braun M et al (2003) Type III secretion genes in Aeromonas salmonicida subsp. salmonicida are located on a large thermolabile virulence plasmid. J Clin Microbiol 41:3854–3856. doi:10.1128/JCM.41.8.3854-3856.2003
Sundell K, Wiklund T (2011) Effect of biofilm formation on antimicrobial tolerance of Flavobacterium psychrophilum. J Fish Dis 34:373–383. doi:10.1111/j.1365-2761.2011.01250.x
Tam B, Gough WA, Tsuji L (2011) The impact of warming on the appearance of furunculosis in fish of the James Bay region, Quebec, Canada. Reg Environ Change 11:123–132. doi:10.1007/s10113-010-0122-8
Tanaka KH, Dallaire-Dufresne S, Daher RK et al (2012) An insertion sequence-dependent plasmid rearrangement in Aeromonas salmonicida causes the loss of the type three secretion system. PLoS One 7:e33725. doi:10.1371/journal.pone.0033725
Touchon M, Barbier P, Bernardet J-F et al (2011) Complete genome sequence of the fish pathogen Flavobacterium branchiophilum. Appl Environ Microbiol 77:7656–7662
Trivedi B (2012) Microbiome: the surface brigade. Nature 492:S60–S61
Trust TJ, Bull LM, Currie BR, Buckley JT (1979) Obligate anaerobic bacteria in the gastrointestinal microflora of the grass carp (Ctenopharyngodon idella), goldfish (Carassius auratus), and rainbow trout (Salmo gairdneri). J Fish Res Board Can 36:1174–1179. doi:10.1139/f79-169
Turnbull JF (1993) Bacterial gill disease and fin rot. In: Inglis V, Roberts RJ, Bromage NR (eds) Bacterial disease fish. Halsted, New York, pp 40–58
Vanden Bergh P, Frey J (2013) Aeromonas salmonicida subsp. salmonicida in the light of its type-three secretion system. Microb Biotechnol. doi:10.1111/1751-7915.12091
Verrier ER, Dorson M, Mauger S, Torhy C, Ciobotaru C, Hervet C, Dechamp N, Genet C, Boudinot P, Quillet E (2013) Resistance to a rhabdovirus (VHSV) in rainbow trout: identification of a major QTL related to innate mechanisms. PLoS One 8, e55302
Vatsos IN, Thompson KD, Adams A (2001) Adhesion of the fish pathogen Flavobacterium psychrophilum to unfertilized eggs of rainbow trout (Oncorhynchus mykiss) and n-hexadecane. Lett Appl Microbiol 33:178–182
Vatsos IN, Thompson KD, Adams A (2006) Colonization of rainbow trout, Oncorhynchus mykiss (Walbaum), eggs by Flavobacterium psychrophilum, the causative agent of rainbow trout fry syndrome. J Fish Dis 29:441–444
Vilches S, Jimenez N, Tomas JM, Merino S (2009) Aeromonas hydrophila AH-3 Type III secretion system expression and regulatory network. Appl Environ Microbiol 75:6382–6392. doi:10.1128/AEM.00222-09
Vinod MG, Shivu MM, Umesha KR et al (2006) Isolation of Vibrio harveyi bacteriophage with a potential for biocontrol of luminous vibriosis in hatchery environments. Aquaculture 255:117–124
Wakabayashi H (1991) Effect of environmental conditions on the infectivity of Flexibacter columnaris to fish. J Fish Dis 14:279–290. doi:10.1111/j.1365-2761.1991.tb00825.x
Wakabayashi H, Egusa S, Fryer JL (1980) Characteristics of filamentous bacteria isolated from a gill disease of salmonids. Can J Fish Aquat Sci 37:1499–1504
Wakabayashi H, Toyama T, Iida T (1994) A study on serotyping of Cytophaga-psychrophila isolated from fishes in Japan. Fish Pathol 29:101–104
Waldvogel FA (1999) New resistance in Staphylococcus aureus. N Engl J Med 340:556–557
Welker TL, Shoemaker CA, Arias CR, Klesius PH (2005) Transmission and detection of Flavobacterium columnare in channel catfish Ictalurus punctatus. Dis Aquat Organ 63:129–138
Whitehead NA, Barnard AM, Slater H et al (2001) Quorum-sensing in Gram-negative bacteria. FEMS Microbiol Rev 25:365–404
Witte W (1998) Medical consequences of antibiotic use in agriculture. Science 279:996–997
Zapata AG, Varas A, Torroba M (1992) Seasonal variations in the immune system of lower vertebrates. Immunol Today 13:142–147
Author information
Authors and Affiliations
Corresponding author
Editor information
Editors and Affiliations
Rights and permissions
Copyright information
© 2016 Springer International Publishing Switzerland
About this chapter
Cite this chapter
Derome, N., Gauthier, J., Boutin, S., Llewellyn, M. (2016). Bacterial Opportunistic Pathogens of Fish. In: Hurst, C. (eds) The Rasputin Effect: When Commensals and Symbionts Become Parasitic. Advances in Environmental Microbiology, vol 3. Springer, Cham. https://doi.org/10.1007/978-3-319-28170-4_4
Download citation
DOI: https://doi.org/10.1007/978-3-319-28170-4_4
Published:
Publisher Name: Springer, Cham
Print ISBN: 978-3-319-28168-1
Online ISBN: 978-3-319-28170-4
eBook Packages: Biomedical and Life SciencesBiomedical and Life Sciences (R0)