Abstract
Molecular forensic techniques to trace DNA from surfaces and sediments have played important roles in conservation and species protection for decades, but their application in the field of ecology is not as well established. In the 1990s, forensic sequencing was applied to non-human samples, illuminating how DNA shed in the environment can be used to track species, populations, and individuals, with implications for animal conservation and management cases. Concurrently, molecular markers such as DNA barcodes were increasingly being published for systematics and biodiversity surveys. Now, forensic techniques are being applied as metabarcoding and metagenomics for ecology and conservation, taking advantage of massive biodiversity DNA reference databases to trace and characterize everything in environmental samples from individuals to whole communities, revealing the provenance and timing of events. Sequencing environments across space and time provides key ecosystem biometrics that point to the causes—and often the culprits—of ecological change. Ecological forensics broadens translational research opportunities in conservation, policy, and environmental justice. This chapter specifically focuses on the increased use of environmental DNA (eDNA), which can be collected from soil, sediment, water, or air, in forensic ecological research from real-time to deep time. The rise of this new subdiscipline has the potential to shape the future of biodiversity management and discovery globally.
Access provided by Autonomous University of Puebla. Download chapter PDF
Similar content being viewed by others
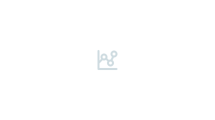
Keywords
An Introduction to Ecology, Biodiversity, and Forensics
Ecology is a broad scientific field, focused on describing organismal interactions with their associated environment (Owen and Owen 1974). Modern ecology focuses on understanding species presence, niches, and how species interact with the ecosystem across trophic levels (Futuyma and Moreno 1988). Documented increases in the severity and duration of disturbances are making ecological-based studies more commonplace, forcing the integration of novel methods and techniques to assess how organisms influence ecosystem function, and vice versa.
Traditional ecological techniques such as visual surveys, video surveillance, and trapping are commonly employed to monitor species. These techniques, however, are time-consuming and involve personnel highly trained in a given taxonomy. The use of molecular methods may prove more reliable, or may complement traditional observation, and facilitate long-term monitoring. Monitoring multiple species at a time is important because environmental resilience and resistance to disturbance depend on species interactions in a system.
Biodiversity is integral to ecosystem function. Highly biodiverse ecosystems such as coral reefs (Hughes et al. 2007), rainforests (Hoorn and Wesselingh 2010), and kelp forests (Steneck et al. 2002) have complex food webs, biogeochemical cycles, and disturbance response strategies, potentially yielding natural systems more resilient to disturbances. Compromises to biodiversity, however, can result in lower functionality (e.g., coral reefs, kelp forest). Biodiversity measurements indirectly provide assessments of ecosystem function (Pauchard et al. 2018). Ecological forensics investigates changes to a system that affect biodiversity, which may require changes in human practices, such as modified fisheries policy (Ogden 2008) and criminal punishment for illegal or damaging actions such as oil spills (Mudge 2008). Ecological forensics also encompasses applications of forensics techniques to solve ecological mysteries such as trophic changes (e.g., Tolimieri et al. 2013; Jouta et al. 2017) that can be interpreted with regard to a legislative framework.
Biodiversity is measured at all organizational levels of the ecosystem, from the diversity of genes within a sample, genetic diversity of populations, or phylogenetic or taxonomic composition of communities to intersecting species occurrence dynamics in order to describe the macrosystem (Pauchard et al. 2018). Species in a community that provide similar functions, such as the ability to metabolize certain elements like nitrogen or sulfur, form functional guilds (Blondel 2003). Functional guilds are long-standing pillars of biodiversity organization for ecological study, for example, microbial functional guilds create the foundations upon which higher trophic levels can assemble (Borst et al. 2018). Strong richness and compositional diversity within a guild as well as between guilds create highly functional ecosystems, with a stronger resilience to disturbance, such as a storm, introduction of a pollutant, or altered nutrient availability. With these concepts in mind, ecological forensic research seeks to determine the root cause of ecological change that affects biodiversity on the genetic, phylogenetic, or functional guild level and to apply these findings to crime and policy.
Box 1 When a Hallmark Food Web Needs Ecological Forensics
A pillar of ecology is the recognition that species distributions and abundances are dynamically driven by trophic interactions. In some locations, kelp forest ecosystems undergo phase shifts (Estes et al. 2004) between kelp-dominated and crustose coralline algae-dominated, depending on the abundance of sea otters (Enhydra lutris). Sea otters maintain the ecosystem through a top-down trophic cascade, and their plight has earned them the place as a poster child of a keystone species that must be protected. Sea otters were nearly over-hunted to extinction, both caused by humans since European arrival (Watson 2000). Once thriving with over 300,000 individuals, their numbers decreased to ~2000 in only 13 locations during the fur trade (Bodkin 2015). The reduction of grazing pressure on kelp resulting from the decline of sea otters broadly reshaped coastal kelp ecosystems. The recovery of sea otter populations has remarkably improved the functioning of kelp ecosystems and benefitted local human communities who can make use of increased fisheries resources associated with kelp forests (Gregr et al. 2020). Nonetheless, there is almost no genomic diversity in southern and northern sea otter populations (Beichman et al. 2019), which makes populations vulnerable.
A recent threat to sea otters has emerged: Toxoplasma gondii, which causes meningoencephalitis disease. In 2004, Toxoplasma caused 16% of sea otter mortality in recovered carcasses. Pathology and multilocus genotyping of the Toxoplasma parasite from otter carcasses and nearby cats revealed cats transmit the most lethal strains of the parasite (Shapiro et al. 2019). Snails, other mollusks, and crustaceans at the adjunct of freshwater runoff and coasts may bioaccumulate Toxoplasma and facilitate its ingestion by otters. University of California (UC) Davis researchers identified marine snow as another bioaccumulator that may enrich kelp beds with the parasite (Shapiro et al. 2012). While direct amplification of Toxoplasma from crustaceans and kelp has been proposed as biomonitoring strategies (Bigot-Clivot et al. 2016), community-based environmental DNA (eDNA) research has revealed that metabarcoding of the 18S rRNA locus from soil, sediment, or water can all detect Toxoplasma without harvesting or harming any organisms (inset figure shows results of 13 positive detections from August 2020; Map from www.ucedna.com, no copyright, permission from author Rachel Meyer; inset by DPDx Parasite Image Library from https://en.wikipedia.org/wiki/Toxoplasma_gondii#/media/File:Toxoplasma_gondii_tachy.jpg). With this data, people can better protect the keystone otter-kelp forest ecosystem that our own species almost wiped out once and that activities such as allowing domestic cats to roam outside continue to threaten.

To explore dimensions of biodiversity and the ecosystem by surveying DNA molecules in the environment, such as using DNA sequences to discern guilds (Bouskill et al. 2012; Nguyen et al. 2016), researchers must consider the nature of deposition and the temporal footprint of molecules. Most organisms shed DNA throughout their development as they move through the environment. Certain events like spawning or release of pollen can increase DNA (Fahner et al. 2016) in the environment, as can death (Tillotson et al. 2018). DNA molecules can either rapidly degrade or remain preserved for many thousands of years (Taberlet et al. 2018), depending on the local environmental conditions, and thus can be useful to characterize systems and assess changes to biodiversity at many space-time scales (e.g., Petrou et al. 2019).
For the last 30 years, the field of forensics has heavily relied on DNA detection and sequencing to solve crimes (Roewer 2013). DNA fingerprinting, microsatellites, and whole genome resequencing have been used to track criminals from cells shed at the scene of a crime from the hair, skin, blood, or semen (Jeffreys et al. 1985). The quick touch of a mirror or door handle can leave behind sufficient genetic signatures for police and prosecutors to capture the criminal and solve the crime (Roewer 2013) by direct match or by associative evidence such as a location-specific microbiome (Pechal et al. 2014). Human DNA from a crime scene is usually directly linked to sequences in curated reference databases such as the Combined DNA Index System (CODIS) that contains reference sequences from victims and registered criminals. In recent years, next-generation and third-generation DNA sequencing has enabled hundreds of thousands of genetic markers to help solve cases through more complex analyses, such as by using genetic genealogies with GEDmatch or FamilyTreeDNA data (Greytak et al. 2019). In turn, a revolution in non-human DNA detection and analysis has also occurred. This is how the Golden State Killer was caught by scientist Barbara Rae-Venter.
The employment of forensics to match a cat hair to a specific cat helped solve a murder case (Menotti-Raymond et al. 1997) in the early 1990s and was quickly followed by use of the same techniques in conservation genetics to monitor poaching and trafficking (e.g., tigers; Kitpipit et al. 2012) as well as crime involving domestic species (Budowle et al. 2005). Programs like RhODIS were designed to mirror the CODIS databases, wherein select genomic loci were matched to solve confiscated rhino horn and poaching cases (Harper 2011; Harper et al. 2018). Concurrently, the establishment of DNA barcoding (Nanney 1982; Hebert et al. 2003) became important in basic biology (e.g., in systematics and ecology), and this also became valuable reference molecular information for environmental and conservation forensics, leading to the growth of DNA databases that represent the tree of life (e.g., National Center for Biotechnology Information, NCBI; Barcode of Life Database, BOLD). NCBI’s GenBank exceeds 700,000 taxa in the year 2020.
Biodiversity sequencing aids in solving criminal cases, answering the questions of “where” and “when” an incident occurred. Metagenomic analysis of genetic sequence data reveals a microbiome that can be exploited to elucidate events, processes, and provenance. For example, the human skin microbiome is an indicator of the postmortem interval (Metcalf 2019). Trace environmental DNA at crime scenes became recognized as providing dozens to thousands of taxa that could resolve provenance robustly through community similarity, and the technique DNA metabarcoding was found to be useful for tracing DNA signatures of many species with substantially lower cost than metagenomics. Quickly, bioinformatic tools to aid metabarcoding analysis have emerged to encourage deployment of this technology in many kinds of forensics (Fløjgaard et al. 2019; Allwood et al. 2020).
Within environmental forensics, the subdiscipline of ecological forensics encompasses multiple fields of molecular investigation, but the field of environmental DNA (eDNA) encompasses most investigations. eDNA analysis commonly uses metagenomics and metabarcoding to probe genetic material found in environmental samples and identify species presence (Thomsen and Willerslev 2015) or characterize community composition (e.g., Stoeck et al. 2010). Researchers can then use this information to reveal missing information needed to explain ecological phenomena. With this in mind, environmental scientists become forensic detectives as they collect samples, extract the DNA, and analyze sequences to determine the genetic, phylogenetic/taxonomic, or functional composition of the ecosystem. This genetic information provides data that can help scientists solve fundamental questions as well as crimes that occur in the environment. These standard community ecology measurements of species richness and compositional diversity help to assess the current status of ecosystem health and aid in future conservation efforts (Deiner et al. 2016).
Methods Used to Study Environmental DNA
Environmental DNA is a mixed sample of DNA from many species that may exist in different levels of degradation and abundance. Relevant to ecological forensics are sample types ranging from bone to soil, water, surfaces, and even air. Shedding of genetic material does not happen on a universal level for organisms, and how organisms shed this material is important in its ability to transport through a variety of mediums, and its “shelf-life” in different environments impacts the types of organisms detected by eDNA sequencing and the duration for which they can be detected (for a review see Barnes and Turner 2016). Researchers mine environmental samples representing different slices of time and space primarily using four techniques: DNA metagenomics; DNA metabarcoding; quantitative PCR (qPCR); and hybridization capture.
DNA metagenomics includes a suite of methods to mine shotgun sequence data or sequences generated from fragments of sequenced DNA that are used to recreate an entire chromosome or genome and match the unknown sample to referenced sequences. Because most of the DNA in environmental samples is microbial, the depth of sequencing required is scaled somewhat with the body size and spatial density of the species of interest—microbial biomes can be characterized with a few hundred thousand reads (Dinsdale et al. 2008), but finding signatures of a specific plant or mammal DNA in a sediment sample may require 10–100 million reads. Even bone, left to decay exposed or buried, becomes overrun with microbial DNA over time, with diminishing endogenous DNA. As perhaps an extreme case, to obtain the entire genome from the bone of an extinct horse relative that roamed over 500,000 years ago, 12.2 billion sequences were required (Orlando et al. 2013). Fortunately, smaller and more prevalent organisms can serve as proxies for larger organisms. For example, dung fungus Sporormiella is an indicator of large mammal presence that can be found in sediments (see Perrotti and van Asperen 2019). The investment in metagenomic sequencing environmental samples can offer incredible returns for ecology, such as by offering multiple lines of evidence to determine whether humans or climate change led to past megafaunal extinctions (Wang et al. 2017) and paleoecological transitions (Hofman et al. 2015). Additionally, metagenomics offers the only means to obtain whole genomes from environmental samples that are needed to potentially de-extinct species (Shapiro 2017). Metagenomics also allows for determination of ancient versus contemporary DNA, because chemical damage to DNA after the organism’s death causes deamination of cytosine (Hofreiter et al. 2001). Thus, metagenomics is conducive to historical forensic cases.
Metagenomes from contemporary samples also provide functional genetic information for microbial strains. Identifying metabolic pathway genes and their links on the same genome is critical to accurately modeling functional guilds and biotic interactions in ecosystems (Barnum et al. 2018). Protocols are continuously being improved to optimize DNA extraction (Rohland et al. 2018) and shotgun library preparation (e.g., SRSLY Kits from Claret Bioscience) for metagenome analysis from degraded samples that allow more genomic information to be obtained from smaller amounts of starting DNA. These same protocols are being used to examine historic human forensic samples (see Astrea Forensics; astreaforensics.com).
DNA metabarcoding is the amplification of target barcode loci to characterize specific groups of organisms such as plants, microbes, or animals. From a single sample, researchers can separately amplify multiple barcode loci and then combine amplicons into a single indexing reaction that is sequenced on Illumina or PacBio instruments to produce multiplexed metabarcoding data and analyzed by matching the DNA reads to reference DNA databases. Some of the most frequently metabarcoded loci include the 16S ribosomal RNA (rRNA) locus, which is diagnostic for bacteria and for mammals; the 18S rRNA locus, which is diagnostic for broad eukaryotes; the internal transcribed spacer (ITS) rRNA gene, often used for diagnosing plants and fungi; the cytochrome C oxidase subunit 1 (CO1) mitochondrial gene, which is diagnostic of animals and protozoa; and the 12S mitochondrial gene to diagnose vertebrates (Creer et al. 2016).
eDNA metabarcoding has proven to be a useful tool to assess community composition, where targeted amplification enhances the representation of specific taxa, even if their sequences are relatively rare compared to bacterial sequences. However, metabarcoding is not without limitations. Rare organisms may be missed (Barnes and Turner 2014), biases inherent in PCR polymerase (Nichols et al. 2018) may skew results, and the inability to discern species from a short barcoding fragment alone may all hamper the abilities of this method to fully elucidate true communities. Additionally, ancient DNA damage patterns cannot be detected with metabarcoding. Nonetheless, with very few reads, an environmental sample usually has sufficient community information to match samples to a candidate provenance (Fløjgaard et al. 2019), or to detect changes in the community (Fahner et al. 2016), which are major objectives. For example, bacterial 16S metabarcoding of chimpanzee feces revealed the “humanization” of the gut microbiome under captivity, which, when combined with deep shotgun sequencing metagenomic analysis, demonstrated a reduction in plants being consumed in captivity as the cause of the microbiome perturbation (Clayton et al. 2016). This signal of captivity and a propensity to disease have policy implications for the ecological structuring of parks, reserves, and zoos.
Quantitative PCR (qPCR) is a powerful tool to rapidly detect a species or group by amplification rather than sequencing. For qPCR, custom primers are typically designed to specifically and unequivocally amplify a short (~50 bp–200 bp) region of DNA from a target in a mixed sample. Either an endogenous control gene fragment is included for semi-quantitative PCR that is useful for comparison of cycle thresholds among samples, or a serial dilution of known target DNA is used as a scaling metric to estimate the absolute number of target DNA molecules in samples. Throughout this chapter we highlight many case studies that use qPCR in these ways. Species detection by qPCR is so heavily relied on for rapid quantification that some workflows have joined field and laboratory steps to actually succeed in obtaining qPCR results in the field within a few hours with portable thermocyclers that connect to mobile phones (Thomas et al. 2019).
Quantitative PCR is sometimes used on eDNA sample extracts to determine the presence of inhibitors such as humic acids, proteins, or salts (Lloyd et al. 2010). Inhibitors are common in the geochemistry of soils and certain plants. Failing to remove inhibitors during DNA extraction can skew results derived from standard methods such as metabarcoding because target DNA will not have an equal chance of being amplified across samples. Many forensic sample types contain inhibitors which can be removed by a variety of purification steps (see Hedman and Rådström 2013). Obtaining qPCR cycle thresholds for a range of sample dilutions can signal the appropriate working dilution where inhibitors have no perceived effect (see Murray et al. 2015).
Hybridization capture using enrichment techniques such as bead-bound “baits” in solution or microarrays with baits on a solid surface combines the benefits of targeted sequencing and metagenomics. Baits are synthesized DNA that probes for and can capture thousands of non-repetitive polymorphic DNA fragments for organisms that are rare in environmental samples. Capture probes allow even old, degraded fragments of DNA as short as 70 bases to be sequenced. Examples of baits used include population-level diagnostics to sort ancient from contemporary DNA to detect Neanderthal relatives of humans (Gansauge and Meyer 2014), to obtain entire mitochondria from degraded human skeletal samples (Marshall et al. 2017), to reconstruct mammalian communities from sediments (e.g., PalaeoChip; Murchie et al. 2019), and to analyze historical plant specimens in herbaria (e.g., Angiosperm353; Johnson et al. 2019) and arthropod collections in museums (Knyshov et al. 2019) at thousands of homologous loci. Like metagenomes, baits-captured DNA molecules retain ancient damage signatures. Functional gene families involved in stress response can be probed in the environment using hybridization to a gene chip such as the GeoChip (He et al. 2007). The GeoChip has been a key to characterize microbial functioning affected by contaminants, pollutants, and naturally occurring elements, from aquifers to groundwater and wastewater (Jiang et al. 2019; Li et al. 2019). Hybridization capture methods have also been successfully used in tracing infectious disease in humans (Gaudin and Desnues 2018), invertebrates (Carpi et al. 2015), and vertebrates such as koalas (Tsangaras et al. 2014).
Tracking Species of Interest with eDNA
Conservation ecology evaluates human impacts on biodiversity and develops practical approaches to simultaneously maximize the preservation of biodiversity and the improvement of human well-being (Kareiva and Marvier 2012). A main objective of conservation science is to establish pragmatic methods for preserving or restoring species and associated ecological communities while reconciling concerns and supporting needs of people. As a consequence, conservation research often focuses on monitoring biodiversity and identifying threats to species or ecosystems. eDNA has the potential to contribute to both biodiversity and threat assessments, and we discuss these below.
Monitoring species of conservation concern is an appealing use for eDNA because the approaches can be sensitive enough to detect rare species and because obtaining eDNA is usually non-destructive. To date, the bulk of studies have focused simply on the presence/absence of species (Shelton et al. 2019), and in many situations such information is sufficient to inform conservation efforts. For instance, Spear et al. (2015) used eDNA and quantitative PCR (qPCR) across 61 sites to assess the presence of the Eastern hellbender (Cryptobranchus alleganiensis alleganiensis), a declining, secretive salamander (Spear et al. 2015). The cryptic nature of hellbenders makes traditional, non-genetic sampling methods extremely difficult, and knowing sites where the salamander resides is a first step in prioritizing conservation efforts. Other studies have used eDNA metabarcoding to assess the occurrence of assemblages of species. For example, sampling natural saltlicks in tropical forests revealed the presence of diverse mammals, including orangutan and banteng (Ishige et al. 2017). In another example, Deiner and colleagues (2016) detected 296 families of eukaryotes, spanning 19 phyla across the catchment of a river. These examples highlight how such spatially integrated snapshots of biodiversity provide useful means for assessing conservation and restoration efforts of rare, imperiled species, as well as the total biodiversity of whole landscapes.
While presence/absence of data is valuable, knowing the relative abundance of individuals can be essential for many conservation applications. Thus, a critical step toward making eDNA methods even more useful in conservation settings is developing the ability to use eDNA quantitatively. Recent evidence suggests that in many situations using eDNA to estimate relative abundance is quite feasible. For example, Tillotson and colleagues (2018) report a strong quantitative relationship between eDNA concentration and the abundance of spawning sockeye salmon in a small stream in Alaska, USA. Similarly, in threatened Chinook salmon (Oncorhynchus tshawytscha) from an estuary in Washington, USA, abundance indices derived from eDNA qPCR reflect the seasonal migration of salmon and provide virtually identical quantitative information as conventional net-based methods (Shelton et al. 2019). Water samples were used to monitor sea otter and fish abundance in California kelp forest (Port et al. 2016), and these abundances were also found to align closely with visual dive survey data.
In addition to assessing the occurrence or abundance of species, eDNA has been used to monitor or evaluate threats to biodiversity. For example, because the structure of communities varies with the primary pollutants in an environment as well as the magnitude of contaminants (Wang et al. 2019b), eDNA is a powerful tool for assessing impacts of pollution. In the case of oil pollution, hydrocarbon-degrading bacterial families will be present, varying in a manner that can be related to the time since an oil spill (Xie et al. 2018). Similarly, the presence of specific bacterial families can indicate a wider sensitivity to oil pollution (Xie et al. 2018). Other researchers have used eDNA focused on eukaryotes to diagnose environmental impacts. For instance, using eDNA technology focused on zooplankton, Yang and colleagues developed an assay for ammonia nitrogen pollution based on the relative abundances of rotifers, copepods, and Cladocera (Yang et al. 2017).
Detection of the presence and dispersal of invasive species has been a mainstay of eDNA conservation research. Early detection of an incipient invasion by a harmful species increases the feasibility of rapid responses to eradicate the species or contain its spread, and eDNA has proven useful as an early detection tool. Jerde et al. (2011) used eDNA to delimit the invasion fronts of two species of Asian carps in area canals and waterways feeding into the Laurentian Great Lakes, leading to better management of these invaders. Assays of Burmese python eDNA in concert with occupancy modeling were able to detect the leading edge of the python invasion in Florida, USA, improving conservation efforts in this region (Hunter et al. 2015). eDNA surveillance of an invasive fish (ruffe, Gymnocephalus cernua) revealed a much more rapid spread of the invader than predicted by conventional sampling techniques (Tucker et al. 2016). As a final example, the non-native American bullfrog, an invasive species in French wetlands (Ficetola et al. 2008), was successfully detected from water samples. Successful detection can give researchers inferences regarding the range dynamics of non-native species, helping to classify the non-native species as invasive. While this technology is generally employed after invasion, there is interest in employing eDNA as a preventative measure. For example, ballast water, a common transport of marine invasive species, could be used to detect non-native species before they invade an ecosystem (Mahon et al. 2014; Egan et al. 2015). The introduction of non-native species can cause major problems for an ecosystem, especially if a population rises to invasive status, making the use of eDNA to track these species a viable and appealing management strategy.
Conservation projects utilizing eDNA have occurred in many different terrestrial and aquatic systems from the tropics to the desert and the Arctic and from wild to captive systems. For example, eDNA was successfully acquired from water holes and used to identify inhabitants of a Japanese zoo (Ushio et al. 2017), suggesting a promising future for forest mammal biodiversity monitoring. eDNA was shown to be a useful tool to inventory mammals from artificial wallows in Colorado, USA (Williams et al. 2018), and from spring and stock tank water in Utah, USA (Rodgers and Mock 2015). In African parks, water hole mammal DNA hybrid capture has become a new tool for biomonitoring (Seeber et al. 2019). These recent findings are leading researchers to ask how deep down in lake and pond sediments can plant and animal DNA be detected from these hot climate areas (Bremond et al. 2017). It may be possible to also use sediments that date back in time to reconstruct the landscape composition of areas before and during civil wars so we know what landscape reconstruction should resemble.
Case Study on eDNA from Sediments: Species Networks to Diagnose Processes
Since the first eDNA study focused on sediment (Ogram et al. 1987), the patterns of occurrence in both microbial and macrobial taxa have shown their value to diagnose ecosystem processes. The co-occurrence of species provides hypotheses about species interactions, which in turn can be used to propose hypotheses regarding the ecological role of species (e.g., keystone species) to disturbance of ecosystems (e.g., invasive species) (Ficetola et al. 2008). Patterns of species can be found in sample sets that vary across both space and time using software such as SPIEC-EASI (Kurtz et al. 2015). In this example case study with preliminary results from Moore (2019), we describe the study in a forensics framework and then show how network analysis of eDNA leads us to posit the mechanism of disturbance processes.
Lagoons
Moore (2019) sought to identify the effects of large macroalgal blooms on the community composition of lagoon systems, specifically during decomposition of the blooms (Moore 2019). Algal blooms are thought to be caused by the increased input of nutrients from human agricultural and wastewater systems. Standard ecological methods were used to identify a cascade of biogeochemical changes caused by these blooms. They then used the ecological forensic method of metabarcoding to characterize biodiversity changes to the lagoon microbiome as microbes are involved in decomposition processes. In between lagoon sampling events, the largest wildfire in California history (the Thomas fire, December 2017) occurred near the lagoons. Because the fire was human-caused, this case study provided evidence of the interaction between two human-caused hazards (suspected culprits), wildfire and nutrient enrichment, on fragile lagoon systems, from the lens of the microbiome. Which culprit has a larger effect, and how does the system respond?
The crime report: Large macroalgal blooms of Ulva sp. in nutrient-enriched lagoon, Carpinteria Salt Marsh in California, lead to large amounts of decomposing material in summers causing eutrophied and acidic water columns. A nearby lagoon in Newport, which is perceived to have more nutrient input from urbanization, does not experience the extreme bloom and eutrophication as Carpinteria, but does experience summer acidification. In addition, the Thomas Fire (December 2017) occurred near the Carpinteria lagoon, which caused movement of biological materials and is expected to alter nutrient pools. The Thomas Fire is also suspected to be exacerbating nutrient deposition and erosion.
The evidence: Algal biomass surveys and acidification measurements (decreased pH) from November 2017 and June 2018.
The victims: Water quality, which is now acidified, and carbonate organisms, which are directly impacted by acidification.
The suspects: Human nutrient pollution from upstream and nutrient-rich sediment runoff following a human induced wildfire.
The weapon: Nutrient alterations interacting with the decomposer microbial species in the system. The way this weapon harmed the victims specifically is unsolved.
The forensic eDNA investigation (Fig. 1a–c): Metabarcoding of the 16S locus targeting bacteria (Caporaso et al. 2011) from multiple samples in November 2017 (winter) and in June 2018 (summer) showed both the mouth and the middle of the Carpinteria lagoon (where the bloom was located) sampled in the summer after the fire were very different from the lagoon head. Because the head did not compositionally shift in the beta diversity (species diversity between samples) ordination plot (Fig. 1a), and because Carpinteria has a much larger bloom than Newport lagoon, wildfire was ruled out as a suspect in causing the microbiome change, and human nutrient pollution was determined to be the culprit.
(a) Beta diversity ordination plot. (b) Aerial photo of Carpinteria Salt Marsh during the Thomas Fire. (NASA MODIS data from LANCE/EOSDIS Rapid Response and Sentinel-2 from https://en.wikipedia.org/wiki/Thomas_Fire#/media/File:Thomas_Fire_burn_scar_on_Dec_7.jpg)(c) Conventional ecological algal biomass survey with both healthy and decomposing Ulva sp. and carbonate snails present. (Photo by Tiara N. Moore)
To characterize the new-found culprit further, the taxon presence and abundance patterns were used to calculate a species co-occurrence network, and of 2046 taxa detected with the 16S metabarcode, a robust network of 32 taxa was discovered (Fig. 2). While many of these microbes are yet uncharacterized or poorly characterized, some, such as the Deltaproteobacteria and Myxococcales, are known decomposers found in lagoon sediments, and gammaproteobacteria, Chromatiales, are sulfide reducers known to increase with increasing decomposition due to elevated nutrient loads (Aires et al. 2019). Myxococcales act as an endophyte in halophytic plants and select green algae (Aires et al. 2015). The presence of Myxococcales in this network suggests it is associated with Ulva. In addition, another member of the network, Micrococcales, has been associated previously linked with Ulva, but not linked to its decomposition (FitzGerald et al. 2015), while other studies have noted Micrococcales performing cellulolysis and have observed a correlation with lower pH on farms in China (Wang et al. 2019a). These results link these members of the lagoon microbiome with Ulva and with eutrophication via their activity as an ecological community.
Taxa present in the ecological co-occurrence network of microbial communities identified across sites and samples. Finding these taxa in a network suggests their cooperation to perform the metabolic function of decomposition. (Background Forest Service photo by Stuart Palley from https://commons.wikimedia.org/wiki/File:Thomas_Fire_(24469612857).jpg)
These findings are typical of classical ecological studies that assess how disturbances affect ecosystems, but by employing ecological forensics methods to characterize the culprit, an additional layer of evidence was discovered: the assessment of the microbial community in the sediment found particular decomposing bacteria that could be linked as co-conspirators in the crime. Due to the increase of these decomposing bacteria, other healthy bacteria typically found in lagoon sediment were suppressed, potentially catalyzing changes in ecosystem services and making other species unexpected victims. The wildfire was also eliminated as a suspect for causing the community turnover by considering it should have effects on the entire system. Ultimately, the paired use of ecological forensics and conventional surveys provided enough evidence to solve our crime and close the investigation.
The Future
Biodiversity is vanishing at an astounding rate and facing the sixth greatest mass extinction (Ceballos et al. 2015; Shivanna 2020). Molecular forensic techniques offer a means to non-invasively and rapidly monitor biodiversity and develop a deeper understanding of how it is maintained on a systems level. The ecological forensics community, however, will need to confront the nature of how DNA molecules distribute and preserve. Perhaps every animal and plant has associated microbes and parasites that can serve as surrogate evidence for their presence. Small organisms are easier to detect than larger ones, and they may be highly diagnostic of patterns and processes, as revealed by the success of microbiome use in forensics and molecular ecology discussed above. The next years will require tremendous effort to better align the signals from eDNA and the signals from conventional ecological methods.
The past several decades have demonstrated that the opening of new forensic techniques opens new fields and subfields of study in ecology. However, the convergence of forensics with phylogenetics and systematics may have produced the greatest gain. Like the usefulness of CODIS and GEDMatch, biodiversity references have revolutionized our precision. Questions must be pursued at the intersection of multiple disciplines, ultimately enriching science. Now that there are many ways in which environmental samples and eDNA can technically be integrated into biodiversity, conservation, and ecological research, can another revolutionary advance for understanding all of the components in an ecosystem simultaneously be produced? Can the acquisition of big data encompassing biodiversity baselines, reference communities, and known patterns of change from disturbances and environmental gradients across space and time allow researchers to finally mechanistically solve the outstanding questions about how ecosystems work and who the key species are? Can these data cause legislation to be revised to support sustainable systems and undo the crimes of the Anthropocene to the environment? In order to focus forensics and policy on systems rather than species, a concerted effort will be required to bring molecular biological interactions in ecosystems and ecological forensics together with social science and public education to become genomics literate and appreciative of microbial biodiversity.
References
Aires T, Moalic Y, Serrao EA, Arnaud-Haond S (2015) Hologenome theory supported by cooccurrence networks of species-specific bacterial communities in siphonous algae (Caulerpa). FEMS Microbiol Ecol. https://doi.org/10.1093/femsec/fiv067
Aires T, Muyzer G, Serrão EA, Engelen AH (2019) Seaweed loads cause stronger bacterial community shifts in coastal lagoon sediments than nutrient loads. Front Microbiol. https://doi.org/10.3389/fmicb.2018.03283
Allwood JS, Fierer N, Dunn RR et al (2020) Use of standardized bioinformatics for the analysis of fungal DNA signatures applied to sample provenance. Forensic Sci Int. https://doi.org/10.1016/j.forsciint.2020.110250
Barnes M, Turner CR (2014) Environmental conditions influence eDNA persistence in aquatic systems. Environ Sci Technol 48:1819–1827. https://doi.org/10.1021/es404734p
Barnes MA, Turner CR (2016) The ecology of environmental DNA and implications for conservation genetics. Conserv Genet. https://doi.org/10.1007/s12686-012-9843-y
Barnum TP, Figueroa IA, Carlström CI et al (2018) Genome-resolved metagenomics identifies genetic mobility, metabolic interactions, and unexpected diversity in perchlorate-reducing communities. ISME J. https://doi.org/10.1038/s41396-018-0081-5
Beichman AC, Koepfli KP, Li G et al (2019) Aquatic adaptation and depleted diversity: a deep dive into the genomes of the Sea Otter and Giant Otter. Mol Biol Evol. https://doi.org/10.1093/molbev/msz101
Bigot-Clivot A, Palos Ladeiro M, Lepoutre A et al (2016) Bioaccumulation of toxoplasma and Cryptosporidium by the freshwater crustacean Gammarus fossarum: involvement in biomonitoring surveys and trophic transfer. Ecotoxicol Environ Saf. https://doi.org/10.1016/j.ecoenv.2016.07.006
Blondel J (2003) Guilds or functional groups: does it matter? Oikos 100:223–231. https://doi.org/10.1034/j.1600-0706.2003.12152.x
Bodkin JL (2015) Historic and contemporary status of sea otters in the North Pacific. Sea Otter Conservation
Borst ACW, Verberk WCEP, Angelini C et al (2018) Foundation species enhance food web complexity through non-trophic facilitation. PLoS One. https://doi.org/10.1371/journal.pone.0199152
Bouskill NJ, Tang J, Riley WJ, Brodie EL (2012) Trait-based representation of biological nitrification: model development, testing, and predicted community composition. Front Microbiol. https://doi.org/10.3389/fmicb.2012.00364
Bremond L, Favier C, Ficetola GF et al (2017) Five thousand years of tropical lake sediment DNA records from Benin. Quat Sci Rev. https://doi.org/10.1016/j.quascirev.2017.06.025
Budowle B, Garofano P, Hellman A et al (2005) Recommendations for animal DNA forensic and identity testing. Int J Legal Med. https://doi.org/10.1007/s00414-005-0545-9
Caporaso JG, Lauber CL, Walters WA et al (2011) Global patterns of 16S rRNA diversity at a depth of millions of sequences per sample. Proc Natl Acad Sci USA. https://doi.org/10.1073/pnas.1000080107
Carpi G, Walter KS, Bent SJ et al (2015) Whole genome capture of vector-borne pathogens from mixed DNA samples: a case study of Borrelia burgdorferi. BMC Genomics. https://doi.org/10.1186/s12864-015-1634-x
Ceballos G, Ehrlich PR, Barnosky AD et al (2015) Accelerated modern human-induced species losses: entering the sixth mass extinction. Sci Adv. https://doi.org/10.1126/sciadv.1400253
Clayton JB, Vangay P, Huang H et al (2016) Captivity humanizes the primate microbiome. Proc Natl Acad Sci U S A. https://doi.org/10.1073/pnas.1521835113
Creer S, Deiner K, Frey S, et al (2016) The ecologist’s field guide to sequence-based identification of biodiversity. Methods Ecol Evol
Deiner K, Fronhofer EA, Mächler E et al (2016) Environmental DNA reveals that rivers are conveyer belts of biodiversity information. Nat Commun. https://doi.org/10.1038/ncomms12544
Dinsdale EA, Edwards RA, Hall D et al (2008) Functional metagenomic profiling of nine biomes. Nature. https://doi.org/10.1038/nature06810
Egan SP, Grey E, Olds B et al (2015) Rapid molecular detection of invasive species in ballast and harbor water by integrating environmental DNA and light transmission spectroscopy. Environ Sci Technol 49:4113–4121. https://doi.org/10.1021/es5058659
Estes JA, Danner EM, Doak DF et al (2004) Complex trophic interactions in kelp forest ecosystems. Bull Mar Sci
Fahner NA, Shokralla S, Baird DJ, Hajibabaei M (2016) Large-scale monitoring of plants through environmental DNA metabarcoding of soil: recovery, resolution, and annotation of four DNA markers. PLoS One. https://doi.org/10.1371/journal.pone.0157505
Ficetola GF, Miaud C, Pompanon F, Taberlet P (2008) Species detection using environmental DNA from water samples. Biol Lett 4:423–425. https://doi.org/10.1098/rsbl.2008.0118
FitzGerald JA, Allen E, Wall DM et al (2015) Methanosarcina play an important role in anaerobic co-digestion of the seaweed Ulva lactuca: taxonomy and predicted metabolism of functional microbial communities. PLoS One. https://doi.org/10.1371/journal.pone.0142603
Fløjgaard C, Frøslev TG, Brunbjerg AK et al (2019) Predicting provenance of forensic soil samples: linking soil to ecological habitats by metabarcoding and supervised classification. PLoS One. https://doi.org/10.1371/journal.pone.0202844
Futuyma DJ, Moreno G (1988) The evolution of ecological specialization. Annu Rev Ecol Syst Vol. https://doi.org/10.1146/annurev.es.19.110188.001231
Gansauge MT, Meyer M (2014) Selective enrichment of damaged DNA molecules for ancient genome sequencing. Genome Res. https://doi.org/10.1101/gr.174201.114
Gaudin M, Desnues C (2018) Hybrid capture-based next generation sequencing and its application to human infectious diseases. Front Microbiol
Gregr EJ, Christensen V, Nichol L et al (2020) Cascading social-ecological costs and benefits triggered by a recovering keystone predator. Science. https://doi.org/10.1126/science.aay5342
Greytak EM, Moore CC, Armentrout SL (2019) Genetic genealogy for cold case and active investigations. Forensic Sci Int. https://doi.org/10.1016/j.forsciint.2019.03.039
Harper C (2011) RhODIS–DNA profiling and a DNA database as a tool to protect the rhino. In: Proceedings of the tenth meeting of the IUCN African Rhino Specialist Group
Harper C, Ludwig A, Clarke A et al (2018) Robust forensic matching of confiscated horns to individual poached African rhinoceros. Curr Biol
He Z, Gentry TJ, Schadt CW et al (2007) GeoChip: a comprehensive microarray for investigating biogeochemical, ecological and environmental processes. ISME J 1:67–77. https://doi.org/10.1038/ismej.2007.2
Hebert PDN, Cywinska A, Ball SL, DeWaard JR (2003) Biological identifications through DNA barcodes. Proc R Soc B Biol Sci. https://doi.org/10.1098/rspb.2002.2218
Hedman J, Rådström P (2013) Overcoming inhibition in real-time diagnostic PCR. Methods Mol Biol. https://doi.org/10.1007/978-1-60327-353-4_2
Hofman CA, Rick TC, Fleischer RC, Maldonado JE (2015) Conservation archaeogenomics: ancient DNA and biodiversity in the Anthropocene. Trends Ecol Evol
Hofreiter M, Jaenicke V, Serre D et al (2001) DNA sequences from multiple amplifications reveal artifacts induced by cytosine deamination in ancient DNA. Nucleic Acids Res. https://doi.org/10.1093/nar/29.23.4793
Hoorn C, Wesselingh FP (2010) Amazonia, landscape and species evolution: a look into the past
Hughes TP, Bellwood DR, Folke CS et al (2007) No-take areas, herbivory and coral reef resilience. Trends Ecol Evol
Hunter ME, Oyler-McCance SJ, Dorazio RM et al (2015) Environmental DNA (eDNA) sampling improves occurrence and detection estimates of invasive Burmese pythons. PLoS One. https://doi.org/10.1371/journal.pone.0121655
Ishige T, Miya M, Ushio M et al (2017) Tropical-forest mammals as detected by environmental DNA at natural saltlicks in Borneo. Biol Conserv. https://doi.org/10.1016/j.biocon.2017.04.023
Jeffreys AJ, Wilson V, Thein SL (1985) Hypervariable ‘minisatellite’ regions in human DNA. Nature 314:67–73. https://doi.org/10.1038/314067a0
Jerde CL, Mahon AR, Chadderton WL, Lodge DM (2011) “Sight-unseen” detection of rare aquatic species using environmental DNA. Conserv Lett. https://doi.org/10.1111/j.1755-263X.2010.00158.x
Jiang Z, Li P, Wang Y et al (2019) Arsenic mobilization in a high arsenic groundwater revealed by metagenomic and geochip analyses. Sci Rep. https://doi.org/10.1038/s41598-019-49365-w
Johnson MG, Pokorny L, Dodsworth S et al (2019) A universal probe set for targeted sequencing of 353 nuclear genes from any flowering plant designed using k-Medoids clustering. Syst Biol. https://doi.org/10.1093/sysbio/syy086
Jouta J, Dietz MW, Reneerkens J et al (2017) Ecological forensics: using single point stable isotope values to infer seasonal schedules of animals after two diet switches. Methods Ecol Evol. https://doi.org/10.1111/2041-210X.12695
Kareiva P, Marvier M (2012) What is conservation science? Bioscience. https://doi.org/10.1525/bio.2012.62.11.5
Kitpipit T, Tobe SS, Kitchener AC et al (2012) The development and validation of a single SNaPshot multiplex for tiger species and subspecies identification - implications for forensic purposes. Forensic Sci Int Genet. https://doi.org/10.1016/j.fsigen.2011.06.001
Knyshov A, Gordon ERL, Weirauch C (2019) Cost-efficient high throughput capture of museum arthropod specimen DNA using PCR-generated baits. Methods Ecol Evol. https://doi.org/10.1111/2041-210X.13169
Kurtz ZD, Müller CL, Miraldi ER et al (2015) Sparse and compositionally robust inference of microbial ecological networks. PLoS Comput Biol. https://doi.org/10.1371/journal.pcbi.1004226
Li Q, You H, Xie W, et al (2019) Review in recent researches and applications of technology of environmental microbiology metagenomics in water treatment engineering. In: IOP conference series: earth and environmental science
Lloyd KG, MacGregor BJ, Teske A (2010) Quantitative PCR methods for RNA and DNA in marine sediments: maximizing yield while overcoming inhibition. FEMS Microbiol Ecol. https://doi.org/10.1111/j.1574-6941.2009.00827.x
Mahon AR, Nathan LR, Jerde CL (2014) Meta-genomic surveillance of invasive species in the bait trade. Conserv Genet Resour 6:563–567. https://doi.org/10.1007/s12686-014-0213-9
Marshall C, Sturk-Andreaggi K, Daniels-Higginbotham J et al (2017) Performance evaluation of a mitogenome capture and Illumina sequencing protocol using non-probative, case-type skeletal samples: implications for the use of a positive control in a next-generation sequencing procedure. Forensic Sci Int Genet. https://doi.org/10.1016/j.fsigen.2017.09.001
Menotti-Raymond MA, David VA, O’Brien SJ (1997) Pet cat hair implicates murder suspect. Nature 386(6627):774
Metcalf JL (2019) Estimating the postmortem interval using microbes: knowledge gaps and a path to technology adoption. Forensic Sci Int Genet
Moore TN (2019) Nutrient enrichment promotes eutrophication in the form of macroalgal blooms causing cascading effects in two Anthropogenically disturbed coastal ecosystems. University of California, Dissertation
Mudge SM (2008) Environmental forensics and the importance of source identification
Murchie TJ, Kuch M, Duggan A et al (2019) PalaeoChip Arctic1.0: an optimised eDNA targeted enrichment approach to reconstructing past environments. bioRxiv 730440. https://doi.org/10.1101/730440
Murray DC, Coghlan ML, Bunce M (2015) From benchtop to desktop: important considerations when designing amplicon sequencing workflows. PLoS One. https://doi.org/10.1371/journal.pone.0124671
Nanney DL (1982) Genes and phenes in tetrahymena. Bioscience. https://doi.org/10.2307/1308971
Nguyen NH, Song Z, Bates ST et al (2016) FUNGuild: an open annotation tool for parsing fungal community datasets by ecological guild. Fungal Ecol. https://doi.org/10.1016/j.funeco.2015.06.006
Nichols RV, Vollmers C, Newsom LA et al (2018) Minimizing polymerase biases in metabarcoding. Mol Ecol Resour. https://doi.org/10.1111/1755-0998.12895
Ogden R (2008) Fisheries forensics: the use of DNA tools for improving compliance, traceability and enforcement in the fishing industry. Fish Fish. https://doi.org/10.1111/j.1467-2979.2008.00305.x
Ogram A, Sayler GS, Barkay T (1987) The extraction and purification of microbial DNA from sediments. J Microbiol Methods. https://doi.org/10.1016/0167-7012(87)90025-X
Orlando L, Ginolhac A, Zhang G et al (2013) Recalibrating equus evolution using the genome sequence of an early middle Pleistocene horse. Nature. https://doi.org/10.1038/nature12323
Owen DF, Owen J (1974) Species diversity in temperate and tropical Ichneumonidae. Nature. https://doi.org/10.1038/249583a0
Pauchard A, Meyerson LA, Bacher S et al (2018) Biodiversity assessments: origin matters. PLoS Biol 16:e2006686
Pechal JL, Crippen TL, Benbow ME et al (2014) The potential use of bacterial community succession in forensics as described by high throughput metagenomic sequencing. Int J Legal Med. https://doi.org/10.1007/s00414-013-0872-1
Perrotti AG, van Asperen E (2019) Dung fungi as a proxy for megaherbivores: opportunities and limitations for archaeological applications. Veg Hist Archaeobot
Petrou EL, Drinan DP, Kopperl R et al (2019) Intraspecific DNA contamination distorts subtle population structure in a marine fish: decontamination of herring samples before restriction-site associated sequencing and its effects on population genetic statistics. Mol Ecol Resour. https://doi.org/10.1111/1755-0998.12978
Port JA, O’Donnell JL, Romero-Maraccini OC et al (2016) Assessing vertebrate biodiversity in a kelp forest ecosystem using environmental DNA. Mol Ecol. https://doi.org/10.1111/mec.13481
Rodgers TW, Mock KE (2015) Drinking water as a source of environmental DNA for the detection of terrestrial wildlife species. Conserv Genet Resour. https://doi.org/10.1007/s12686-015-0478-7
Roewer L (2013) DNA fingerprinting in forensics: past, present, future. Investig Genet
Rohland N, Glocke I, Aximu-Petri A, Meyer M (2018) Extraction of highly degraded DNA from ancient bones, teeth and sediments for high-throughput sequencing. Nat Protoc. https://doi.org/10.1038/s41596-018-0050-5
Seeber PA, McEwen GK, Löber U et al (2019) Terrestrial mammal surveillance using hybridization capture of environmental DNA from African waterholes. Mol Ecol Resour 19:1486–1496. https://doi.org/10.1111/1755-0998.13069
Shapiro B (2017) Pathways to de-extinction: how close can we get to resurrection of an extinct species? Funct Ecol 31:996–1002. https://doi.org/10.1111/1365-2435.12705
Shapiro K, Silver MW, Largier JL et al (2012) Association of Toxoplasma gondii oocysts with fresh, estuarine, and marine macroaggregates. Limnol Oceanogr. https://doi.org/10.4319/lo.2012.57.2.0449
Shapiro K, VanWormer E, Packham A et al (2019) Type X strains of toxoplasma gondii are virulent for southern sea otters (Enhydra lutris nereis) and present in felids from nearby watersheds. Proc R Soc B Biol Sci. https://doi.org/10.1098/rspb.2019.1334
Shelton AO, Kelly RP, O’Donnell JL et al (2019) Environmental DNA provides quantitative estimates of a threatened salmon species. Biol Conserv. https://doi.org/10.1016/j.biocon.2019.07.003
Shivanna KR (2020) The sixth mass extinction crisis and its impact on biodiversity and human welfare. Resonance. https://doi.org/10.1007/s12045-019-0924-z
Spear SF, Groves JD, Williams LA, Waits LP (2015) Using environmental DNA methods to improve detectability in a hellbender (Cryptobranchus alleganiensis) monitoring program. Biol Conserv. https://doi.org/10.1016/j.biocon.2014.11.016
Steneck RS, Graham MH, Bourque BJ et al (2002) Kelp forest ecosystems: biodiversity, stability, resilience and future. Environ Conserv
Stoeck T, Bass D, Nebel M et al (2010) Multiple marker parallel tag environmental DNA sequencing reveals a highly complex eukaryotic community in marine anoxic water. Mol Ecol. https://doi.org/10.1111/j.1365-294X.2009.04480.x
Taberlet P, Bonin A, Zinger L, Coissac E (2018) Environmental DNA: for biodiversity research and monitoring. Oxford University Press, Oxford
Thomas AC, Tank S, Nguyen PL et al (2019) A system for rapid eDNA detection of aquatic invasive species. Environ DNA. https://doi.org/10.1002/edn3.25
Thomsen PF, Willerslev E (2015) Environmental DNA - an emerging tool in conservation for monitoring past and present biodiversity. Biol Conserv 183:4–18. https://doi.org/10.1016/j.biocon.2014.11.019
Tillotson MD, Kelly RP, Duda JJ et al (2018) Concentrations of environmental DNA (eDNA) reflect spawning salmon abundance at fine spatial and temporal scales. Biol Conserv. https://doi.org/10.1016/j.biocon.2018.01.030
Tolimieri N, Samhouri JF, Simon V et al (2013) Linking the trophic fingerprint of groundfishes to ecosystem structure and function in the California current. Ecosystems. https://doi.org/10.1007/s10021-013-9680-1
Tsangaras K, Siracusa MC, Nikolaidis N et al (2014) Hybridization capture reveals evolution and conservation across the entire koala retrovirus genome. PLoS One. https://doi.org/10.1371/journal.pone.0095633
Tucker AJ, Chadderton WL, Jerde CL et al (2016) A sensitive environmental DNA (eDNA) assay leads to new insights on Ruffe (Gymnocephalus cernua) spread in North America. Biol Invasions. https://doi.org/10.1007/s10530-016-1209-z
Ushio M, Fukuda H, Inoue T et al (2017) Environmental DNA enables detection of terrestrial mammals from forest pond water. Mol Ecol Resour 17:e63–e75. https://doi.org/10.1111/1755-0998.12690
Wang Y, Heintzman PD, Newsom L et al (2017) The southern coastal Beringian land bridge: cryptic refugium or pseudorefugium for woody plants during the last glacial maximum? J Biogeogr. https://doi.org/10.1111/jbi.13010
Wang CY, Zhou X, Guo D et al (2019a) Soil pH is the primary factor driving the distribution and function of microorganisms in farmland soils in northeastern China. Ann Microbiol. https://doi.org/10.1007/s13213-019-01529-9
Wang P, Yan Z, Yang S, et al (2019b) Environmental DNA: an emerging tool in ecological assessment. Bull Environ Contam Toxicol
Watson J (2000) The effects of sea otters (Enhydra lutris) on abalone (Haliotis spp.) populations. In: Eorkshop on rebuilding abalone stocks in British Columbia
Williams KE, Huyvaert KP, Vercauteren KC et al (2018) Detection and persistence of environmental DNA from an invasive, terrestrial mammal. Ecol Evol. https://doi.org/10.1002/ece3.3698
Xie Y, Zhang X, Yang J et al (2018) eDNA-based bioassessment of coastal sediments impacted by an oil spill. Environ Pollut. https://doi.org/10.1016/j.envpol.2018.02.081
Yang J, Zhang X, Xie Y et al (2017) Ecogenomics of zooplankton community reveals ecological threshold of Ammonia nitrogen. Environ Sci Technol. https://doi.org/10.1021/acs.est.6b05606
Author information
Authors and Affiliations
Editor information
Editors and Affiliations
Rights and permissions
Copyright information
© 2021 Springer Nature Switzerland AG
About this chapter
Cite this chapter
Moore, T., Gaynus, C., Levin, P.S., Meyer, R. (2021). The Intersection of Forensic Techniques with Ecological Issues. In: Underkoffler, S.C., Adams, H.R. (eds) Wildlife Biodiversity Conservation. Springer, Cham. https://doi.org/10.1007/978-3-030-64682-0_7
Download citation
DOI: https://doi.org/10.1007/978-3-030-64682-0_7
Published:
Publisher Name: Springer, Cham
Print ISBN: 978-3-030-64681-3
Online ISBN: 978-3-030-64682-0
eBook Packages: Biomedical and Life SciencesBiomedical and Life Sciences (R0)