Abstract
Changes in volumetric activity of 85Kr radioactive inert gas take place in the atmosphere: it has increased by around 50% during the past 15 years. The main source of such gas is the operation of nuclear power plants and spent nuclear fuel reprocessing plants. 85Kr as an inert gas spreads throughout the entire atmosphere and its ionizing radiation may result in changes of atmospheric electric phenomena. Therefore it is necessary to control 85Kr emission into the atmosphere. However, there is no effective method for this as inert gases, under normal conditions, can hardly be adsorbed in different adsorbents and stored in special containers for a long period of time. This paper tries to show the possibility of keeping 85Kr longer within the adsorbent by changing its aggregate state: gas is adsorbed into liquid adsorbent and desorption takes place from solid adsorbent. For this purpose, an epoxy resin is used which, after adding a special hardener at room temperature, turns into a solid material with density of around 1.2 × 103 kg m−3. As a result of sample blending with substances which contribute to better solubility of 85Kr, diffusion coefficient of this gas (i.e. desorption speed) changes within the adsorbent in the solid state.
Similar content being viewed by others
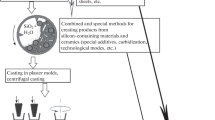
Explore related subjects
Discover the latest articles, news and stories from top researchers in related subjects.Avoid common mistakes on your manuscript.
Introduction
As nuclear power expands, radioactive inert gases enter the atmosphere. Long-lived radioactive inert gases take part in the atmospheric circulation system and spread throughout the entire atmosphere. One of the long-lived radioactive inert gases of high importance is Krypton-85 (\( {}_{36}^{85} {\text{Kr}} \)). The radioactivity decay half-life of this radionuclide is T 1/2 = 10.76 m; radioactivity decay constant is λ = 2.04 × 10 −9 s−1; 99.563% of radiation consists of beta particles with energy of 0.687 MeV, and the rest of 0.437% of radiation −0.173 MeV. Gamma radiation energy is equal to 0.514 MeV [1, 2].
One of the sources of 85Kr in the atmosphere is nuclear explosions and not only in the atmosphere but also under the earth, or under water. A 1 Mt nuclear explosion results in the fission of around 56 kilograms of 235U; this means 4.6 × 1023 of 85Kr atoms released (0.32% yield) [3]. Supposedly, emissions of 85Kr from this source amounted to 111–185 PBq in the atmosphere in 1973 [4]. Atmospheric activity of 5 × 106 Ci caused by 85Kr during the period 1945–1962 is indicated in the article Krypton-85 [1].
The other way 85Kr gets into the atmosphere is during the operation of nuclear plants; in cases where 235U fuel is used, activity of this gas amounts to 6.2 × 1012 Bq (MW m)−1, 233U—\( 12.2 \times 10^{12}\; {\text{Bq}}\;({\text{MW}}\;{\text{m}})^{ - 1} \), 239Pu—\( 2.7 \times 10^{13} \;{\text{Bq}}\;({\text{MW}}\;{\text{m}})^{ - 1} \) [3]. Large volumes of 85Kr have been released into the atmosphere during accidents in nuclear power plants; e.g. Chernobyl Nuclear Plant catastrophe resulted in emissions of 85Kr with activity of around 35 PBq [1, 4], and the Three Mille accident—around \( 5 \times 10^{4} \;{\text{Ci}}\;(185 \times 10^{13} \;{\text{Bq}}) \) [1].
The significant source of atmospheric emissions of 85Kr is spent nuclear fuel reprocessing (regeneration) plants; capacity of the activity of 85Kr emitted by large plants might amount to \( 1.8 \times 10^{9} \;{\text{Bq}}\;{\text{s}}^{ - 1} \) [2, 3]. Winger et al. [4] provided detailed evaluation of 85Kr emissions from spent nuclear fuel reprocessing plants in La Hague (France), Chelyabinsk (Russia), Tomsk (Russia), Sellafield (UK), Dounray (UK), and Hanford (USA). The above mentioned authors have estimated that activity of 85Kr emitted into the atmosphere during the period 1945–2000 reaches up to \( 10.6 \times 10^{18} \;{\text{Bq}} \) [4]. The paper of Smith et al. [5] determines significant increase of 85Kr activity at the sampling location which was in downwind direction from La Hague nuclear fuel reprocessing plant. Most of the authors’ papers [1, 4–7] declare continuous increase of 85Kr activity concentration in the atmosphere. Dubasov and Okunev [8] had been monitoring 85Kr activity concentration in Cherepovets district (Vologda region) and determined that activity concentration (volumetric activity) of this radionuclide had increased almost by 50% during 15 years and grew from 1.41 to 1.94 Bq m−3 during the period 2006–2008. This results correspond to the data of Japanese researchers [9]. As in the past, nowadays, scientists pay much of their attention to modelling the spread of 85Kr in the atmosphere as this helps not only to research the distribution of this gas in the atmosphere of the Earth, but also provides information on air mass movement [4, 10–12].
Accumulation of 85Kr in the atmosphere intensifies the process of ionization and this might result in irreversible climate changes. Increase of ionization in the atmosphere due to the impact of ionizing radiation of 85Kr is shown in the paper by Loosli [13] and proven in the article by Harrison and ApSimon [14]. Possible increase of lightweight ion concentration until 2015 had been researched in the work by Styra and Butkus [3]. Modelling experiments have determined the dependence of lightweight ion concentration increase under conditions of 85Kr gas being in contaminated environment [15–18]. Atmospheric ionization may influence geophysical processes most significantly in the atmosphere above the Earth’s surface up to thunderclouds and inside of them. Beta radiation of average energy (0.251 MeV), along the travel distance of 0.751 m, creates 6,459 ion couples in the atmosphere. Increase of ion concentration in the atmosphere results in change of aerosol particle concentration and spectrum. The question whether such processes are capable of changing electrical resistance of the atmosphere and all the atmospheric processes related to it cannot be answered yet.
The other atmospheric phenomena resulted by ionizing radiation of 85Kr is the ionization effects inside the thunderclouds. Only the 85Kr, being long-lived radionuclide and inactive in reactions with environmental objects, is able to achieve the same volumetric activity inside a thundercloud as on the Earth’s surface [2]. Distribution of radionuclides in the atmosphere provides with information on the flow of air masse [19].
For the purpose of finding out possible impact of ionizing radiation of 85Kr to the atmospheric processes, it is necessary to develop modelling experiments in that direction and compare the results with feasible natural observations. We see the necessity of the following experiments and theoretic researches:
-
1.
Containment of 85Kr to prevent its emissions into the atmosphere from nuclear power plants and nuclear fuel reprocessing companies.
-
2.
Development of radioactive inert gas chemistry with the purpose of finding substances in which 85Kr could be adsorbed or chemically combined and then disposed in radioactive material containers for a long time.
-
3.
Experimental research of the environmental ionization caused by ionizing radiation of 85Kr.
-
4.
Development of theoretic researches of the above mentioned processes.
Adsorption of 85Kr in various substances was actively researched around the middle of the twentieth century [2]. Most of the works of this nature were done using activated charcoal as an adsorbent [20]. However, effective adsorption of inert gases and long time storage in the activated charcoal or other adsorbents is only possible at low temperatures [16].
We have to admit that search for new adsorbents for 85Kr is negligible during current period; in fact, some research results have not only scientific but also practical significance [21]. Sorption capacity of radioactive materials varies with an adsorbent pH, time of material contact with an adsorbat [22, 23] and physical status of an adsorbent [24]. However, data to characterize adsorption of 85Kr was not found.
The objective of this work is to increase desorption duration and extend the period of gas storage in adsorbents by means of adsorption of long-lived radioactive inert gases into hardening mixtures.
Experimental
For the purpose to achieve the objective of the work, the modelling experiment has been carried out; it started on April 23, 1999. Samples (adsorbents) of six types have been prepared:
Sample 1. E-6 epoxy resin and a hardener; ratio 1:0.1. Sample volume \( V = 122 \times 10^{ - 6} \;{\text{m}}^{3} \) (a cylinder with diameter of \( d = 72 \times 10^{ - 3} \;{\text{m}} \) and a height of \( h = 3 \times 10^{ - 2} \;{\text{m}} \)).
Sample 2. E-6 epoxy resin, hardener and toluene; ratio 1:0.1:0.15. Sample volume \( 163 \times 10^{ - 6} \;{\text{m}}^{3} \) (a cylinder with diameter of \( d = 72 \times 10^{ - 3} \;{\text{m}} \) and a height of \( h = 4 \times 10^{ - 2} \;{\text{m}} \)).
Sample 3. E-6 epoxy resin, hardener and type BAU activated charcoal; ratio 1:0.1:0.5. Sample volume \( V = 324 \times 10^{ - 6} \;{\text{m}}^{3} \) (a cylinder with diameter of \( d = 72 \times 10^{ - 3} \;{\text{m}} \) and a height of \( h = 55 \times 10^{ - 3} \;{\text{m}} \)).
Sample 4. E-6 epoxy resin, hardener and type BAU activated charcoal; ratio 1:0.1:0.35. Sample volume \( V = 10^{ - 4} \;{\text{m}}^{3} \) (a cylinder with diameter of \( d = 72 \times 10^{ - 3} \;{\text{m}} \) and a height of \( h = 24,6 \times 10^{ - 3} \;{\text{m}} \)).
Sample 5. E-6 epoxy resin, hardener and xylene (grade A oil xylene); ratio 1:0.1:0.35. 0.1:0.13. Sample volume \( V = 122 \times 10^{ - 6} \;{\text{m}}^{3} \) (a cylinder with diameter of \( d = 72 \times 10^{ - 3} \;{\text{m}} \) and a height of \( h = 3 \times 10^{ - 2} \;{\text{m}} \)).
Sample 6. E-6 epoxy resin, hardener and butanol (\( {\text{C}}_{4} {\text{H}}_{9} {\text{OH}} \)); ratio 1:0.1:0.13. Sample volume \( V = 122 \times 10^{ - 6} \;{\text{m}}^{3} \) (a cylinder with diameter of \( d = 72 \times 10^{ - 3} \;{\text{m}} \) and a height of \( h = 3 \times 10^{ - 2} \;{\text{m}} \)).
During the experiments, temperature of samples has been increasing, especially with type BAU activated charcoal. In this case, volume and temperature of the solid has been increasing rapidly and even broke the closed glass container. There was almost no temperature increase in mixture of epoxy resin and xylene.
The experiment was engineered in the following way. In the “hot zone”, a glass container with volume of \( 0.5 \times 10^{ - 3} \;{\text{m}}^{3} \) was filled with 85Kr gas and sealed. The activity of 85Kr gas contained in the vessel has been measured and amounted to \( 2.75 \times 10^{6} \;{\text{Bq}} \). Gas with such activity was dosed into the container (1) of the experimental system shown in Fig. 1.
Layout of experimental equipment: 1—85Kr dosing container; 2—glass container with hardening mixture; 3—filter; 4—SBT-11 counter for 85Kr activity measurement; 5—reometer; 6—column submersed into a liquid nitrogen for adsorption of 85Kr contained in the system (system cleaning of 85Kr); 7—pump; 8—valve for pressurizing containers (1) and (2); 9—3-way valve for dosing system diversion; 10—hoses to/from 85Kr source camera; 11—fume cupboard
Using radioactive gas with known activity value, the effectiveness of the counter 4 (Fig. 1) has been determined and amounted to \( K_{1} = 0.79 \times 10^{ - 3} \;{\text{imp}}\;{\text{Bq}}^{ - 1} \;{\text{s}}^{ - 1} \) for 85Kr gas. It was used for determination of volumetric activity of 85Kr in the system shown in Fig. 1.
While the mixture in the container (2) (Fig. 1) is not hardened, the bubbling method is used to saturate the sample with 85Kr gas. A sample is taken for determination of volumetric activity of 85Kr by means of UMF-1500M low background radiation device. Effectiveness of this device, when measuring in the standard dish of the device, amounts to \( K_{2} = 10^{ - 3} \;{\text{imp}}\;{\text{Bq}}^{ - 1} \;{\text{s}}^{ - 1} \). Volume of the mixture used to fill the dish is \( V_{1} = 1.96 \times 10^{ - 6} \;{\text{m}}^{3} \). Calculation of measurement results is performed using this formula:
where \( A_{{V_{1} }} \) is the volumetric activity of 85Kr in a sample, Bq m−3; N 1 the sample radiation intensity, imp s−1; K 2 the effectiveness of the UMF-1500M device, imp Bq−1 s−1; and V 1 is the volume of the sample measured, m3. Activity of the background radiation equals to 0.22 imp s−1.
After the mixture in container (2) (Fig. 1) hardens, circular suction in the system is stopped, containers (1) and (2) are pressurized and 85Kr remaining in the system is sucked into the column (6). After this, container (2) is disconnected from the system, then broken and the activity of the cylinder-shaped sample taken out is measured using SBT-10 counter.
Activity of the radiation emitted from the surface of the solid sample taken out from the container (2) (Fig. 1) was measured by the SBT-10 counter contained within the lead housing, the diagram of which is shown in Fig. 2. At the beginning of each measurement, the background radiation was determined and fluctuated from 55 to 63 imp s−1.
85Kr is likely distributed evenly within the sample contained in vessel (2) and the volumetric activity of the gas is close to that of air in the system as inert gases form no compounds at normal conditions.
Results and discussion
85Kr desorption from samples
Results of measured volumetric activity of 85Kr in the system’s (Fig. 1) air are compared with those calculated by the formula (1). Data obtained presented in Table 1.
As we can see in the Table 1, accumulation of 85Kr radioactive inert gas in respect to environmental 85Kr can only be expected in pure E-6 epoxy resin and mixture with xylene.
Hardened samples were stored at room temperature and a proportion of desorpted 85Kr was measured at certain intervals using equipment shown in Fig. 2. Results of the analysis presented in Table 2.
The most intense desorption takes place during the first 100 days: it amounts to 1.91 × 10−4 m−2 s−1 from mixture of E-6 epoxy resin, hardener and toluene, and mixture of E-6 epoxy resin and a hardener. Desorption significantly slows down in 200 days. 85Kr desorption rate from mixture of E-6 epoxy resin, hardener and toluene, and mixture of E-6 epoxy resin and a hardener, not taking into account the decay of nuclear isotope, amounts to an average of \( 1.9 \times 10^{ - 5} \;{\text{m}}^{ - 2} \;{\text{s}}^{ - 1} \) and \( 2.1 \times 10^{ - 5} \;{\text{m}}^{ - 2} \;{\text{s}}^{ - 1} \) accordingly.
After all samples were stored 3,870 days at room temperature and then cut horizontally, density of the surface activity (Bq m−2) of 85Kr in the middle layer was significantly higher than the surface layer. This data presented in Table 3.
Figure 3 shows change of 85Kr activity concentration (%) in Sample 6 from the centre towards the edge depending on the time t (days).
Model of Krypton concentration distribution in a sample
Decrease of concentration of any radioactive substance in a sample is influenced by two interdependent physical phenomena: spread (diffusion) of a substance through the surface of a sample according to the diffusion equation:
and radioactive decay, intensity of which is proportional to the residual volume of a substance or the activity concentration:
where D is the diffusion coefficient, Δ the Laplace operator, T 1/2 the decay half-life, and C 1, C 2 is the respective concentrations.
As these phenomena take place simultaneously, total distribution of concentration in a cylinder-shaped sample (R—base radius, H—height) in the polar coordinate system (φ, r, z) might be defined by the following boundary value problem:
Due to the axial symmetry of the sample shape, it is assumed that concentration is not dependent on the angle φ. Moreover, it is supposed that activity concentration, at the beginning of the experiment, is the same in each point of a sample:
.
Solution of the marginal problem for distribution of activity concentration of Krypton
Traditionally, boundary value problems in mathematical physics are solved by approximate methods; however, in case of a cylinder-shaped sample, the diffusion problem (2)–(3) can be expressed in analytical form using the method of separation of variables [25]:
where
where \( C_{0} (r,z) \) is the initial distribution of concentrations; J k (r) the Bessel functions of order k; and μ j is the roots of equation J 0 (μ) = 0.
On the grounds of condition (4), integral (5) could be calculated and the result is:
Solution of the problem (2)–(3) might be expressed as a product of two separate series:
Calculation of diffusion coefficient
Let’s take Q(t) as an amount of diffused 85Kr during time period of (0,t). Then, the result of integration of flow \( - D\int_{S} {{\frac{\partial C}{\partial n}}} {\text{d}}s \) according to time is as follows:
where S,V is the sample’s surface area and volume accordingly and Δ is the Laplace operator.
The initial amount of 85Kr is \( Q_{0} = \pi R^{2} HC_{0} \) so calculation of integrals (7) including (6) results in the following expression of value \( Q(t)/Q_{0} \) characterizing the desorption rate:
Series of the obtained expression converge rapidly enough (not slower than 1/i 2 and 1/j 2) therefore, after calculation of the sum of several tens of members, the accuracy achieved is not lower than the accuracy of desorption measurements performed. Least-squares method (i.e. comparison of calculated \( Q(t)/Q_{0} \) values having volatile diffusion coefficient with measured \( \widetilde{Q}(t_{i} )/Q_{0} \) values) is used to find approximate values of diffusion coefficients of corresponding samples. These values are presented in Table 4 and distribution of measured \( \widetilde{Q}(t_{i} )/Q_{0} \) values in respect of curves (8) is shown in Fig. 4.
Measurement results and approximation curves of the data (8)
Mean-square error values, calculated using expression
stay below 2% in all samples (vary from 0.67 to 2%), and this allows us to suppose that the mathematical model used corresponds sufficiently accurately to the process of activity concentration change, and the values of diffusion coefficients calculated might be used for evaluation of adsorption features of various aggregates.
Conclusions
Cylinder shaped, E-6 epoxy resin based adsorbents with additions of hardener, xylene, toluene, butanol, and activated carbon have been made; volume of the cylinders was \( 1 \times 10^{ - 4} \;{\text{m}}^{3} \) to \( 3.24 \times 10^{ - 4} \;{\text{m}}^{3} \) and density in solid state amounted to around \( 1.2 \times 10^{3} \;{\text{kg}}\;{\text{m}}^{ - 3} \).
In different samples, the volumetric activity of 85Kr adsorbed into a liquid sample varied from \( 0.3 \times 10^{9} \;{\text{Bq}}\;{\text{m}}^{ - 3} \) (Sample 3: mixture with charcoal) to \( 3.8 \times 10^{9} \;{\text{Bq}}\;{\text{m}}^{ - 3} \) (Sample 5: mixture with xylene).
Desorption of 85Kr has been registered during 3,461 days, during 697 of which this was performed regularly. During the mentioned period, when desorption of 85Kr has been taking place at room temperature, the mean diffusion coefficient was determined in the adsorbent.
Sample 1—\( 3.77 \times 10^{ - 13} \;{\text{m}}^{ 2} {\text{s}}^{-1} \); Sample 2—\( 6.67 \times 10^{ - 13} \;{\text{m}}^{2} {\text{s}}^{-1} \); Sample 3—\( 130.79 \times 10^{ - 13} \;{\text{m}}^{2} {\text{s}}^{-1} \); Sample 4—\( 14.00 \times 10^{ - 13} \;{\text{m}}^{2} {\text{s}}^{-1} \); Sample 5—\( 3.55 \times 10^{ - 13} \;{\text{m}}^{2} {\text{s}}^{-1} \); Sample 6—\( 7.84 \times 10^{ - 13} \;{\text{m}}^{2} {\text{s}}^{-1} \).
As diffusion of 85Kr from a sample into the environment takes place, the distribution of 85Kr in not continuous in a sample; after 3,870 days, the density of the surface activity of 85Kr is higher in outer layers than in the inner ones: Sample 1—by 3.1 times; Sample 2—by 9.3 times; Sample 4—by 7.2 times; Sample 5—by 5.2 times; Sample 6—by 3.8 times.
Adsorption effectiveness and desorption rate of 85Kr in a sample depends on sample’s admixtures which contribute to rapid solubility of this gas.
References
Krypton-85. http://en.wikipedia.org/wiki/krypton-85.2009
Butkus D (1999) Radioactive inert gases of the artificial origin in the environment: scientific investigations and technical solutions. Summary of the research report presented for habilitation, 47 pp
Styra B, Butkus D (1990) Geophysical problems of Krypton-85 in the atmosphere. Hemisphere Publishing Corporation, New York, 153 pp
Winger K, Feichter J, Kalinowski MB, Sartorius H, Schlosser C (2005) A new compilation of the atmospheric Krypton-85 inventories from 1945 to 2000 and its evaluation in a global transport model. J Environ Radioact 80:183–215
Smith K, Murray M, Wong J, Long SC, Colgan PA, Rafferty B (2005) Krypton-85 and other airborne radioactivity measurements throughout Ireland. Radioprotection 40(1):5457–5463. doi:10.1051/radiopro:2005s1-067
Bилгeлмoвa Л, Toмaшeк M, Двopжaк З, Бyткyc Д, Зeмкaюc К, Cтыpo Б (1991) Oпpeдeлeниe aтмocфepныx кoнцeнтpaций \( {}^{85}{\text{Kr}} \) в Пpaгe и в Bильнюce [Vilgelmova L, Tomashek M, Dvorzhak Z, Butkus D, Zemkayus K, Styro B (1991) Determination of atmospheric concentrations of 85Kr in Prague and Vilnius]. Физикa aтмocфepы [Atmos Phys] 15:21–29
Achkasov SK, Gudkov AN, Zakharov OV, Krylov AYu, Nekrasov VM, Novichkov VP, Serbulov YuA, Ushakova NP, Zadorozhnyj YuA (1991) Monitoring the contamination of the atmosphere by 85Kr. Atomnaya Energiya 70(4):234–239
Dubasov YV, Okunev NS (2010) Xenon and Krypton-85 radionuclides monitoring in the northwest region. Pure Appl Geophys 167(4–5):487–498
Mamoshima N, Inoue F, Sugihara S, Shimada J, Taniguchi M (2010) An improved method for 85Kr analysis by liquid scintillation counting and its application to atmospheric 85Kr determination. J Environ Radioact 101(8):615–621
Kalinowski MB (1997) Measurements and modelling of atmospheric Krypton-85 as indicator for plutonium separation. International Workshop on the Status of Measurement Techniques for the Identification of Nuclear Signatures, Geel
Kalinowski M, Feichter J, Ross O (2006) Atmospheric Krypton-85 transport modeling for verification purposes. INESAP Inf Bull N 27:17–20
Takayasu M, Jida T, Watanabe H, Takeishi M, Yamamoto A (2008) Simulation of the atmospheric dispersion of 85Kr from a reprocessing plant over a coastal area. J Radioanal Nucl Chem 275(1):43–54
Loosli HH (1984) Haben künstlich erzeugte Raionuclide wie \( {}^{ 8 5}{\text{Kr,}}\;{}^{ 1 4}{\text{C}} \) und \( {}^{3}{\text{H}} \) mit der Luftionisation, mit dem sauren Regen und dem aldsterben etwas zu tun? Separatdruck ans dem SVA Bulletin N3:21–31
Harrison RG, ApSimon HM (1994) Krypton-85 pollution and atmospheric electricity. Atmos Environ 28(4):637–648
Butkus D, Krenevičius R (1996) Influence of radioactive noble gases on the air ionization variation in the environment of nuclear power plants and nuclear fuel reprocessing plants. Atmos Phys 18(2):43–49
Butkus D (1998) Atmospheric ionization caused by ionizing radiation of radioactive noble gas. Aplinkos Inžinerija 6(4):128–132
Ulevičius V, Butkus D, Plauškaitė K, Girgždys A, Byčenkienė S, Špirkauskaitė N (2009) Impact of Krypton-85 beta radiation on aerosol particle formation and transformation. Lith J Phys 49(4):471–478
Бyткyc, Дoнaтac. Paдиoaктивныe инepтныe гaзы иcкyccтвeoгo пpoиcxoждeния в oкpyжaющeй cpeдe: нayчныe иccлeдoвaния и тeчничecкиe peшeния. Гaбилитaциoннaя paбoтa. Bильнюc [Butkus, D. Radioactive inert gases of the artificial origin in the environment: scientific investigations and technical solutions. Habilitation paper. Vilnius], 1999, 166 c
Styra D, Čiučelis A, Usovaitė A, Damauskaitė J (2008) On possibility of short-term prognosis of cyclonic activity after-effects in Vilnius by variation of hard cosmic ray flux. J Environ Eng Landsc Manag 16(4):159–167
Yamamoto T, Tsukui K, Ootsuka N (1984) Storage of Krypton-85 by adsorption method. J Nucl Sci Technol 21(5):372–380
Mitev K, Pressyanov D, Dimitrova I, Georgiev S, Boshkova T, Zhivkova V (2009) Measurement of Krypton-85 in water by absorption in polycarbonates. Nucl Instrum Methods Phys Res A 602(3):491–494. ISSN 0168-9002
Galamboš M, Kufčakova J, Rajec P (2009) Sorption of stroncium on Slovak betonites. J Radioanal Nucl Chem 281(3):347–357
Galamboš M, Paučova V, Kufčakova J, Rosskopfova O, Rajec P, Adamcova R (2010) Cesium sorption on betonites and montmorillonite K10. J Radioanal Nucl Chem 284(1):55–64
Gao L, Yang Z, Shi K, Wang X, Guo Z, Wu W (2010) U(VI) sorption on kaolinite: effects of pH, U(VI) concentration and oxyanions. J Radioanal Nucl Chem 284(3):519–526
Mapтиcoн ЛК, Maлoв ЮИ. Дифepeнциaльныe ypaвнeния мaтeмaтичecкoй физики. 2-e издaниe. Mocквa: Издaтeльcтвo MГTУ им. H.E. Бayмaнa [Martison LK, Malov YuI. Differential equations of mathematical physics], 2002, 368 c. ISBN 5-7038-1911-3
Acknowledgments
Experimental research has been started at the Institute of Physics. Authors express their sincere thanks to the administration of the Institute of Physics for the material facilities, and also senior engineer Gintas Kandrotas and technician Jurijus Podoroga for their contribution in preparing the samples and conducting the research.
Author information
Authors and Affiliations
Corresponding author
Rights and permissions
About this article
Cite this article
Butkus, D., Kleiza, J. Adsorption of 85Kr radioactive inert gas into hardening mixtures. J Radioanal Nucl Chem 287, 247–254 (2011). https://doi.org/10.1007/s10967-010-0691-5
Received:
Published:
Issue Date:
DOI: https://doi.org/10.1007/s10967-010-0691-5