Abstract
Wheat seedlings cv. Zyta were treated with Cu, Ni and Cd at the concentrations causing approximately 50 % root growth inhibition, i.e. 12.5, 50 and 60 μM, respectively. Tissue metal accumulation, membrane permeability, lipid peroxidation, protein oxidation, concentration of thiol compounds as well as protease, glutathione S-transferase (GST) and peroxidase (POD) activities were studied in roots after 7 days of metal exposure. The metals showed different concentrations in root tissues with Cu and Cd being accumulated to the smallest and to the greatest extent, respectively. Membrane permeability was significantly enhanced by Cu and Ni but not by Cd treatment. All metals induced similar increase in protein oxidation, while significant enhancement of lipid peroxidation was observed only in the case of Cu treatment. The detected thiol compounds: cysteine (Cys), homocysteine (Hcy), γ-glutamylcysteine (γ-GluCys) and glutathione (GSH) were differently influenced by the metal treatment. Ni appeared to be the most effective inductor of GSH accumulation while both Cu and Ni similarly increased Cys content in the roots. Accumulation of γ-GluCys was found in response to Cu and Cd applications. Concentration of Hcy was enhanced by Cd treatment but exposure to Ni decreased its content below the level of detection. The activity of GST was considerably elevated by Cd and Ni treatments, while POD activity was increased only in response to Cu application. Our study showed that wheat roots differently responded to treatment with metals used at the concentrations having similar impact on growth.
Similar content being viewed by others
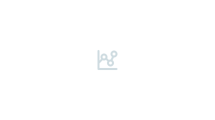
Explore related subjects
Discover the latest articles, news and stories from top researchers in related subjects.Avoid common mistakes on your manuscript.
Introduction
Heavy metal pollution resulting from industrialization, mining and other anthropogenic activities is an important factor limiting productivity of crop plants. Phytotoxicity of heavy metals has been ascribed to their detrimental impact on different physiological processes and metabolic pathways (Prasad 1995; Maksymiec 1997; Seregin and Kozhevnikova 2006). Besides, a growing body of evidence indicates that toxic effect of heavy metals on plants is related to oxidative stress resulting from imbalance between generation and removal of reactive oxygen species by antioxidative system of a cell (Sharma and Dietz 2008). Accumulation of lipid peroxides and protein carbonyls considered as markers of oxidative stress has been reported for plants exposed to various heavy metals, irrespective of their redox properties (Dietz et al. 1999).
Tolerance of plants to heavy metals depends on effective functioning of a complex defense system consisting of metal-binding compounds, low molecular antioxidants, antioxidative enzymes and those participating in detoxification processes. Formation of complexes between metal ions and thiol compounds is considered as a specific defense strategy against heavy metal toxicity (Cobbett and Goldsbrough 2002). Cysteine (Cys), produced at the final step of sulfate assimilation pathway, is indispensable for biosynthesis of all thiol metabolites in plants. Synthesis of tripeptide glutathione (γ-glutamylcysteinylglycine, GSH) is catalyzed by two ATP-dependent enzymes: γ-glutamylcysteine synthetase (EC 6.3.2.2) mediating formation γ-glutamylcysteine (γ-GluCys) from glutamate (Glu) and Cys and glutathione synthetase (EC 6.3.2.3) that ligates a glycine (Gly) residue with γ-GluCys to form GSH. Phytochelatins (PC) with the general structure (γ-GluCys)nGly that are produced by PC synthase (EC 2.3.2.15) are specific metal-binding polypeptides playing an important role in transport of heavy metals and their sequestration in a vacuole (Grill et al. 1989). Literature data indicate that Cys and GSH not only serve as substrates for PC but also have the ability to act as metal chelators (Dokken et al. 2009; Delalande et al. 2010). Additionally, GSH is a very important low-molecular antioxidant involved in maintaining the redox balance in plant cells and is used as a substrate for different GSH-dependent enzymes (Yadav 2010).
An important role in the removal of xenobiotics as well as toxic endogenous compounds, such as breakdown products of lipid and protein oxidation, is ascribed to glutathione S-transferase (GST, EC 2.5.1.18) that catalyses conjugation of electrophilic substrates to GSH (Marrs 1996; Edwards and Dixon 2004). It has also been suggested that GST may participate in the formation and transport of complexes between metals and thiol compounds (Adamis et al. 2004). Peroxidase (POD, EC 1.11.1.7.) that catalyses H2O2-dependent oxidation of numerous substrates including phenolic compounds takes part in several processes of cell wall strengthening such as lignification and cross linking of hydroxyproline rich proteins and feruloylated polysaccharides (Hiraga et al. 2001). Peroxidase-mediated changes in cell wall structure have been suggested to play a role in restriction of metal uptake by plant cells as well as to be responsible for reduction of plant growth observed under stress conditions (Díaz et al. 2001).
Wheat, showing considerably high sensitivity to heavy metals is one of the major food crops worldwide. The objective of our work was to get better insight into the functioning of this important species under heavy metal stress. In most studies aimed at comparing the biochemical response of plants to various heavy metals, the metals are used at equal concentrations. However, sensitivity of plants to metals differs a lot and the same dose of various metals usually induces diverse stress severity in a studied plant (Ivanov et al. 2003). Growth reduction, reflecting disturbances in physiological and metabolic processes is considered to be a reliable indicator of plant sensitivity to heavy metal stress. The purpose of our work was to compare the biochemical response of wheat roots to Cu, Ni and Cd used at the concentrations causing comparable effect on root growth, i.e. 50 % length reduction. Our study was focused on oxidative stress and activation of defense mechanisms in the roots of heavy metal-treated wheat seedlings. Intensity of oxidative stress was evaluated by measuring lipid peroxide and protein carbonyl contents. The studied defense reactions included production of low molecular thiol compounds and induction of protease, GST and POD activities.
Materials and methods
Plant material and growth conditions
Seeds of wheat (Triticum aestivum L., cv. Zyta) provided by Hodowla Roślin Strzelce Sp. z o.o., Poland were germinated in Petri dishes for 2 days. Then the seedlings were transferred into the diluted (1:4) Hoagland nutrient solution containing 12.5 μM Cu, 50 μM Ni or 60 μM Cd supplied as sulphates. The pH of the nutrient solution was adjusted to 5.8. The above metal concentrations caused 50 % reduction in root length increment after 7 days of exposure and were chosen on the basis of our preliminary experiment. The seedlings grown in the nutrient solution without metal supplementation were considered as the control. The seedlings were grown in a controlled climate room at 24 °C with 16-h photoperiod (175 μmol m−2 s−1 PPDF). After 7 days the roots were harvested and concentrations of Cu, Ni, Cd and thiol compounds, electrolyte leakage, lipid peroxidation and protein oxidation as well as protease, GST and POD activities were estimated.
Cu, Ni, and Cd concentrations
Concentrations of Cu, Ni and Cd in the wheat roots were determined by atomic absorption spectrometry. After harvesting the roots were carefully rinsed and dried. Then the oven-dried tissue was digested in HNO3:HClO4 (4:1, v/v) solution at 140 °C. Metal contents were determined using Varian SpectrAA 300 spectrometer (Varian Australia Pty. Ldt., Mulgrave, Australia) equipped with deuterium lamp for background correction and an air/acetylene flame. Concentrations of Cu, Ni and Cd were estimated at 324.8, 232 and 228.8 nm, respectively. The standards of Cu, Ni and Cd (T.J.Baker) were used for calibration. The accuracy of analyses was evaluated with the certified reference material (tea leaves, INCT-TL-1, Institute of Chemistry and Nuclear Techniques, Warsaw, Poland) subjected to the same digestion procedure and determination conditions as described above. Metal contents in the roots were expressed in μg per g DW.
Electrolyte leakage
To evaluate membrane permeability electrolyte leakage from cells was determined conductometrically according to the modified method of Masood et al. (2006). Briefly, the samples of root tissue (0.2 g) were washed in deionised water and then placed in flasks containing 20 ml of deionised water. The samples were shaken gently (100 rpm) for 2 h at room temperature and electrical conductance (EC1) of liquid was determined afterwards using Mettler-Toledo conductometer (Mettler-Toledo GmbH, Schwerzenbach, Switzerland). Then, the samples, still in the same solution, were incubated in boiling water bath for 10 min to kill the tissue completely and conductance (EC2) was measured again. The electrolyte leakage (EL) was calculated according to the formula: EL = (EC1/EC2) × 100 %.
Lipid peroxidation
The quantity of lipid peroxidation products was measured in terms of thiobarbituric acid-reacting substances (TBARS) content according to the modified method of Yagi (1976) as described previously (Gajewska and Skłodowska 2010). The level of lipid peroxides was expressed in nmol of TBARS per g FW.
Protein oxidation
Protein oxidation, given as carbonyl protein (CO-protein) content was measured by reaction with 2,4-dinitrophenylhydrazine (DNPH) according to Levine et al. (1990) as described previously (Gajewska and Skłodowska 2010). Carbonyl content was expressed in nmol of CO per mg protein.
Thiol compounds
To analyze thiol compounds the samples were homogenized (1:3 w/v) in an ice-cold mortar with 50 mM sodium phosphate buffer pH 7.0 and centrifuged (20,000×g, 20 min). The thiol levels were assayed using the method of Głowacki and Bald (2009). Briefly, supernatant (50 μl) was diluted with 50 μl of 0.1 M sodium phosphate buffer pH 7.4. Disulfide bonds were reduced by treatment with 10 μl of 0.25 mM tris(2-carboxyethyl)phosphine (TCEP) for 10 min, and derivatized with 10 μl of 0.1 M 2-chloro-1-methylquinolinium tetrafluoroborate (CMQT) for 3 min at room temperature. The reaction mixture was treated with 50 μl of 3 M perchloric acid, the precipitated proteins removed by centrifugation (12,000×g, 10 min), and 20 μl of the supernatant was injected onto a C18 HPLC column. Concentrations of thiol compounds in the roots were expressed in nmol per g FW.
Enzyme activity
Proteolytic activity as well as POD and GST activities were extracted and assayed as described earlier (Gajewska and Skłodowska 2010). The proteolytic activity was expressed in units, each representing the amount of enzyme causing increase in absorbance (measured at 366 nm) by 0.01 per 1 h. POD activity was expressed in units, each representing the amount of enzyme catalyzing the formation of 1 μmole of tetraguaiacol per minute. GST activity was expressed in units, each representing the amount of enzyme catalyzing the formation of 1 nmole of S-conjugates per minute.
Protein content in the samples was determined by the method of Bradford (1976), with standard curve prepared using bovine serum albumin.
Statistical analyses
The results presented are the means of 5 independent experiments (n = 5). Sample variability was estimated by standard deviation of the mean. The significance of differences between the control and treatment mean values were determined by Student’s t test using Statistica 9.0 software (StatSoft Inc., Tulsa, OK, USA). Differences at P < 0.05 were considered significant.
Results
Metal accumulation
Concentrations of Cu, Ni and Cd in roots of the control wheat seedlings differed considerably, with Cd being the least and Cu the most abundant (Table 1). Exposure of the wheat seedlings to Cu, Ni and Cd led to significant increase in these metals contents in the roots in comparison to the control, with Cu showing the lowest and Cd the highest accumulation in the root. Application of 12.5 μM Cu, 50 μM Ni and 60 μM Cd resulted in 37-fold, 280-fold and 1861-fold increases in the respective metal concentrations as compared to the control values.
Electrolyte leakage, oxidative stress and proteolytic activities
Electrolyte leakage reflecting membrane permeability of root cells was not significantly influenced by Cd application (Fig. 1a). On the contrary, Cu and Ni treatments increased electrolyte leakage by 45 and 40 % over the control level, respectively. Lipid peroxidation, estimated on the basis of TBARS concentration, was enhanced by 44 % in the case of Cu treatment, while it was not significantly altered after Cd and Ni application (Fig. 1b). All metals led to similar increase in carbonyl groups content, ranging from 28 to 34 % over the control level (Fig. 1c). Protease activities, both acid (pH 5.0) and slightly basic (pH 7.5) were not significantly influenced by metal treatments (Fig. 1d).
Electrolyte leakage (a), lipid peroxidation (b), protein oxidation (c) and protease activities (d) in wheat roots after 7 days of treatment with 12.5 μM Cu, 50 μM Ni and 60 μM Cd. Bars represent SD of means. n = 5. *, **, *** Indicate values that differ significantly from the control at P < 0.05, P < 0.01 and P < 0.001, respectively
Thiol compounds
In the samples obtained from wheat roots 4 thiol compounds were identified by HPLC analysis: Cys, Hcy, γ-GluCys and GSH. Treatment with metals had differential impact on their contents. Application of Cd did not influence Cys content in the roots, while Cu and Ni caused 2.9- and 2.4-fold enhancement of this amino acid concentration, respectively (Fig. 2a). Concentration of Hcy increased over 2-fold in response to Cd application but it was not influenced by Cu treatment. Exposure to Ni led to decrease in Hcy content below the level of detection (Fig. 2b). All metals used in our experiment caused enhancement of the total GSH content in the roots (Fig. 2c). Exposure to Cu and Cd resulted in 3.3- and 2.6-fold increase in GSH concentration, respectively. Nickel was the most effective inductor of GSH accumulation leading to almost 15-fold increase in this tripeptide content in the roots. Neither the control roots nor those treated with Ni contained detectable quantities of γ-GluCys (Fig. 2d). Concentration of this thiol in Cd-treated roots 4 times exceeded that found in Cu-treated ones.
GST and POD activities
Treatment with Cu did not significantly influence GST activity in the wheat roots (Fig. 3a). In contrast, application of Cd and Ni resulted in enhancement in this enzyme activity, by 184 and 59 %, respectively. The activity of POD increased by 50 % in response to Cu exposure, while the other metals used did not significantly alter this enzyme activity (Fig. 3b).
Discussion
Inhibition of root growth, one of the most common symptoms of metal toxicity, is often used to assess toxicity of metals for plants. Taking into consideration values of concentrations causing 50 % reduction in root growth: 12.5 μM for Cu, 50 μM for Ni and 60 μM for Cd, Cu was the most toxic for wheat plants cv. Zyta, while Ni and Cd showed similar toxicity, about 4–5 times lower in comparison to Cu. The observed differences in toxicity of the studied metals did not depend on the level of their uptake by the roots since Cu showing the greatest toxicity was accumulated at the lowest level. Our experiment also revealed that the amounts of metals taken up by the wheat roots were not closely related to their concentrations in the growing medium. Cd content in the roots treated with this metal was almost twofold higher in comparison with Ni although both metals were used at similar doses. It has been suggested that phytotoxicity of metals, besides their ability to enter plant tissues, may be also related to their physicochemical properties including affinity to SH groups or electronegativity (Ivanov et al. 2003).
Intensity of oxidative stress in plant tissues is usually estimated on the basis of the level of lipid peroxidation. In our work elevated lipid peroxidation was found in Cu-treated roots while in Ni- and Cd-treated ones it was not significantly altered. Similarly, in the roots of chamomile plants treated with the same metals significant induction of lipid peroxidation was observed only in response to Cu application (Kováčik et al. 2006, 2008, 2009). This result may be explained by differences in redox properties of the metals used. Copper, being a transition metal, exhibits the highest oxidative potential and ability to catalyze Fenton/Haber–Weiss reaction that leads to generation of hydroxyl radicals. Lack of increase in lipid peroxidation found in Ni- and Cd-treated wheat roots may be associated with the fact that these metals possess much lower redox activity in comparison with Cu and can induce oxidative stress only indirectly. However, in our earlier studies we demonstrated that higher doses of Ni and Cd enhanced lipid peroxide level in the wheat roots (Gajewska and Skłodowska 2010), which suggests that the ability of these metals to induce lipid peroxidation may depend on their concentration.
Detrimental impact of heavy metals on biological membranes, reflected by their increased permeability, is considered to be associated with oxidative damage of membrane lipids (Wong-ekkabut et al. 2007). This is in line with our results obtained for Cu-treated wheat roots in which electrolyte leakage from root cells and lipid peroxidation were increased to the similar extent. However, in Ni-exposed roots enhancement of electrolyte leakage was not accompanied by significant induction of lipid peroxidation, which suggests involvement of other disturbances leading to elevated membrane permeability. It was demonstrated that Ni might induce changes in membrane fatty acid profile, lipid composition, and plasmalemma ATPase function (Ros et al. 1992; Gajewska et al. 2012).
Contrary to lipid peroxidation, which was enhanced exclusively by Cu treatment, protein carbonylation in the wheat roots was elevated after treatment with all metals used in our experiment. Moreover, all treatments resulted in similar increase in protein carbonyls content, which corresponded with the same root growth reduction indicating comparable stress severity. It is believed that the intracellular level of oxidized proteins reflects the balance between the rate of protein oxidation and its degradation by proteases (Pena et al. 2008). In our experiment neither acid (pH 5.0) nor slightly basic (pH 7.5) protease activities were affected by the metal treatment. It seems possible that accumulation of carbonylated proteins observed in heavy metal-treated wheat roots might be associated with insufficient functioning of the proteolytic system.
Low molecular thiol compounds are considered to play an important role in protection of plants against heavy metals and their elevated concentrations were often detected in plant tissues in response to metal stress (Cobbett and Goldsbrough 2002; Yadav, 2010). In our experiment all the metals studied influenced quantities of thiol compounds, however in a different manner. Elevated level of Cys content was found in the wheat roots treated with Cu and Ni, which is in agreement with previous studies concerning the effect of these metals on plans (Gupta et al. 2004; Srivastava et al. 2006; Alves de Oliveira et al. 2009). However, in contrast to Mishra et al. (2009) we did not observe increase in this amino acid content after treatment with Cd. Enhancement of Cys concentration might be attributed to the fact that it serves as a precursor for synthesis of other thiol compounds involved in plant reaction to heavy metal stress. Besides, there is evidence that Cys itself may be directly involved in defense against metals due to its ability to form stable complexes with heavy metals such as Cu (Dokken et al. 2009).
Homocysteine is an amino acid not utilized for protein synthesis, however it serves as an intermediate in methionine metabolism (Macnicol et al. 1981; Blom and Smulders 2011). We found that Hcy content in the wheat roots was diversely influenced by Cd and Ni treatments. While Cd stress enhanced concentration of this compound, application of Ni led to its decrease below the level of detection. This suggests the metal-induced disturbances in this amino acid metabolism resulting in its accumulation or increased degradation. To our knowledge involvement of Hcy in plant response to stress has not been studied yet. Further research is needed to get insight into the role of this amino acid in plant reaction to heavy metals.
In the roots of control plants γ-GluCys is present in very low concentrations such as 0.05 nmol g−1 FW (Tari et al. 2002) or is even below the detection level (Meuwly and Rauser 1992). In our experiment the control and Ni-treated roots did not contain detectable amounts of γ-GluCys, however this dipeptide was found in the roots exposed to Cu and Cd and in Cd-treated ones was about fourfold more abundant. Accumulation of γ-GluCys in response to treatment with these metals was previously reported for wheat, maize, water hyacinth and salvinia (Meuwly and Rauser 1992; Tari et al. 2002; Alves de Oliveira et al. 2009). Increase in this dipeptide content was suggested to result from induction of γ-GluCys synthetase activity or elevated rate of GSH catabolism. The second possibility is rather not likely to occur in the case of our study, since we did not observe decreased GSH contents in the wheat roots that accumulated γ-GluCys.
In agreement with the results obtained by Gupta et al. (2004) all metals used in our experiment caused enhancement of GSH content in the wheat roots. Accumulation of GSH under heavy metal stress may be associated with increased demand for this tripeptide serving as a substrate in phytochelatin synthesis. However, in our work the most pronounced accumulation of GSH was found in the roots exposed to Ni, which is considered to be a much weaker activator of phytochelatin synthesis in comparison to Cd or Cu (Schat et al. 2002). Literature data indicate that elevated GSH content in plant tissues may be related to tolerance to Ni, however the protective role of this tripeptide seems to be associated rather with its antioxidative properties than its involvement in metal chelation (Freeman et al. 2004).
In our study an inverse relation was found between GST activity and accumulation of lipid peroxidation products. In Cu-treated roots unchanged GST activity coincided with enhanced level of TBARS while in Ni- and Cd-exposed roots, showing a considerable induction of this enzyme activity, the concentration of TBARS did not significantly differ from that of the control. This observation implies that under heavy metal stress GST may participate in controlling the level of toxic products derived from lipid peroxidation. Increase in GST activity in Cd-treated roots was much more pronounced than that found in Ni-exposed ones. Similar effect was also observed in barley roots treated with both these metals used at concentrations causing approximately 45 % root growth inhibition (Tamás et al. 2008). Stimulation of GST activity in response to Cd treatment might be associated with another function proposed for this enzyme under heavy metal stress, i.e. its involvement in metal chelation by thiol compounds. GST was demonstrated to be necessary for the formation of complexes between Cd and GSH in Saccharomyces cerevisiae (Adamis et al. 2004). However, Delalande et al. (2010) showed recently that production of Cd(GS)2 and Cd(γ-GluCys)2 complexes was spontaneous and did not require participation of GST.
Increased activity of a non-specific peroxidase accompanying reduction in root length was reported for plants exposed to heavy metals including Cu, Ni and Cd (Gabbrielli et al. 1999; Chen et al. 2000; Díaz et al. 2001). In line with these observations in our previous work dealing with the effect of equal Cu, Ni and Cd concentrations (75 μM) on wheat seedlings, the highest induction of POD activity found in Cu-treated roots coincided with the most pronounced growth reduction (Gajewska and Skłodowska 2010). Surprisingly, in the present experiment significantly increased POD activity was observed only in response to Cu application, although all the metals used led to similar growth inhibition of the wheat root system. This result indicated that induction of POD activity was not associated with the degree of root growth reduction, however its relationship with high sensitivity of the wheat roots to Cu cannot be excluded.
In conclusion, our experiment demonstrated differential responses of wheat roots to treatment with Cu, Ni and Cd used at the concentrations similarly affecting growth. Cu, causing 50 % root growth inhibition at the lowest dose was proved to be the most toxic for wheat. There was no relation between the toxicity of the metals used and their accumulation in root tissues. High toxicity of Cu may be associated with its prooxidative properties, which was evidenced by increased lipid peroxidation level in the roots. The metals used in our study induced specific changes in the concentrations of low molecular thiols. Our results suggest that induction of GST activity may be related to lower sensitivity of a plant to heavy metals while enhancement of POD activity may reflect high plant sensitivity to these stress factors.
References
Adamis PDB, Gomes DS, Pinto MLCC, Panek AD, Eleutherio ECA (2004) The role of glutathione transferases in cadmium stress. Toxicol Lett 154:81–88
Alves de Oliveira J, Cambraia J, Valle de Sousa M, Oliva MA (2009) Sulphate uptake and metabolism in water hyacinth and salvinia during cadmium stress. Aquatic Bot 91:257–261
Blom HJ, Smulders Y (2011) Overview of homocysteine and folate metabolism. With special references to cardiovascular disease and neural tube defects. J Inherit Matab Dis 34:75–81
Bradford MM (1976) A rapid and sensitive method for the quantification of microgram quantities of protein utilizing the principle of protein-dye binding. Anal Biochem 72:248–254
Chen L-M, Lin CC, Kao CH (2000) Copper toxicity in rice seedlings: changes in antioxidative enzyme activities, H2O2 level, and cell wall peroxidase activity in roots. Bot Bull Acad Sin 41:99–103
Cobbett C, Goldsbrough P (2002) Phytochelatins and metallothioneins: roles in heavy metal detoxification and homeostasis. Annu Rev Plant Biol 53:159–182
Delalande O, Desvaux H, Godat E, Valleix A, Junot C, Labarre J, Boulard Y (2010) Cadmium - glutathione solution structures provide new insights into heavy metal detoxification. FEBS J 277:5086–5096
Díaz J, Bernal A, Pomar F, Merino F (2001) Induction of shikimate dehydrogenase and peroxidase in pepper (Capsicum annuum L.) seedlings in response to copper stress and its relation to lignification. Plant Sci 161:179–188
Dietz K-J, Baier M, Krämer U (1999) Free radicals and reactive oxygen species as mediators of heavy metal toxicity in plants. In: Prasad MNV, Hagemeyer J (eds) Heavy metal stress in plants—from biomolecules to ecosystems. Springer, Berlin, pp 73–97
Dokken KM, Parsons JG, McClure J, Gardea-Torresdey JL (2009) Synthesis and structural analysis of copper(II) cysteine complexes. Inorg Chim Acta 362:395–401
Edwards R, Dixon DD (2004) Metabolism of natural and xenobiotic substrates by the plant glutathione S-transferase superfamily. In: Sandermann H (ed) Molecular ecotoxicology of plants. Ecological studies, vol 170. Springer, Berlin, pp 17–50
Freeman JL, Persans MW, Nieman K, Albrecht C, Peer W, Pickering IJ, Salt DE (2004) Increased glutathione biosynthesis plays a role in nickel tolerance in Thlaspi nickel hyperaccumulators. Plant Cell 16:2176–2191
Gabbrielli R, Pandolfini T, Espen L, Palandri MR (1999) Growth, peroxidase activity and cytological modifications in Pisum sativum seedlings exposed to Ni2+ toxicity. J Plant Physiol 155:639–645
Gajewska E, Skłodowska M (2010) Differential effect of equal copper, cadmium and nickel concentration on biochemical reactions in wheat seedlings. Ecotoxicol Environ Safe 73:996–1003
Gajewska E, Bernat P, Długoński J, Skłodowska M (2012) Effect of nickel on membrane integrity, lipid peroxidation and fatty acid composition in wheat seedlings. J Agron Crop Sci 198:286–294
Głowacki R, Bald E (2009) Fully automated method for simultaneous determination of total cysteine, cysteinylglycine, glutathione and homocysteine in plasma by HPLC with UV absorbance detection. J Chromatogr B 877:3400–3404
Grill E, Löffler S, Winnacker EL, Zenk MH (1989) Phytochelatins, the heavy-metal-binding peptides of plants, are synthesized from glutathione by a specific γ-glutamylcysteine dipeptidyl transpeptidase (phytochelatin synthase). Proc Natl Acad Sci USA 86:6838–6842
Gupta DK, Tohoyama H, Joho M, Inouhe M (2004) Changes in the levels of phytochelatins and related metal-binding peptides in chickpea seedlings exposed to arsenic and different heavy metal ions. J Plant Res 117:253–256
Hiraga S, Sasaki K, Ito H, Ohashi Y, Matsui H (2001) A large family of class III plant peroxidases. Plant Cell Physiol 42:462–468
Ivanov VB, Bystrova EI, Seregin IV (2003) Comparative impacts of heavy metals on root growth as related to their specificity and selectivity. Russ J Plant Physiol 50:398–406
Kováčik J, Tomko J, Bačkor M, Repčák M (2006) Matricaria chamomilla is not a hyperaccumulator, but tolerant to cadmium stress. Plant Growth Regul 50:239–247
Kováčik J, Grúz J, Bačkor M, Tomko J, Strnad M, Repčák M (2008) Phenolic compounds composition and physiological attributes of Matricaria chamomilla grown in copper excess. Environ Exp Bot 62:145–152
Kováčik J, Klejdus B, Kaduková J, Bačkor M (2009) Physiology of Matricaria chamomilla esposed to nickel excess. Ecotoxicol Environ Safe 72:603–609
Levine RL, Garland D, Oliver CN, Amici A, Climent I, Lenz A-G, Ahn B-W, Shaltiel S, Stadtman ER (1990) Determination of carbonyl content in oxidatively modified proteins. Methods Enzymol 186:464–478
Macnicol PK, Datko AH, Giovanelli J, Mudd SH (1981) Homocysteine biosyntheis in green plants: physiological importance of the transsulfuration pathway in Lemna paucicostata. Plant Physiol 68:619–625
Maksymiec W (1997) Effect of copper on cellular processes in higher plants. Photosynthetica 34:321–342
Marrs KA (1996) The functions and regulation of glutathione S-transferases in plants. Ann Rev Plant Physiol Plant Mol Biol 47:127–158
Masood A, Shah NA, Zeeshan M, Abraham G (2006) Differential response of antioxidant enzymes to salinity stress in two varieties of Azolla (Azolla pinnata and Azolla filiculoides). Environ Exp Bot 58:216–222
Meuwly P, Rauser WE (1992) Alteration of thiol pools in roots and shoots of maize seedlings exposed to cadmium. Plant Physiol 99:8–15
Mishra S, Tripathi RD, Srivastava S, Dwivedi S, Trivedi PK, Dhankher OP, Khare A (2009) Thiol metabolism play significant role during cadmium detoxification by Ceratophyllum demersum L. Bioresour Technol 100:2155–2161
Pena LB, Zawoznik MS, Tomaro ML, Gallego SM (2008) Heavy metal effects on proteolytic system in sunflower leaves. Chemosphere 72:741–746
Prasad MNV (1995) Cadmium toxicity and tolerance in vascular plants. Environ Exp Bot 35:525–545
Ros R, Cook DT, Martinez-Cortina C, Picazo I (1992) Nickel and cadmium-related changes in growth, plasma membrane lipid composition, ATPase hydrolytic activity and proton-pumping of rice (Oryza sativa L. cv. Bahia) shoots. J Exp Bot 43:1475–1481
Schat H, Liugany M, Vooijs R, Hartley-Whitaker J, Bleeker PM (2002) The role of phytochelatins in constitutive and adaptive heavy metal tolerances in hyperaccumulator and non-hyperaccumulator metallophytes. J Exp Bot 53:2381–2392
Seregin IV, Kozhevnikova AD (2006) Physiological role of nickel and its toxic effects on higher plants. Russ J Plant Physiol 53:257–277
Sharma SS, Dietz K-J (2008) The relationship between metal toxicity and cellular redox imbalance. Trends Plant Sci 14:43–50
Srivastava S, Mishra S, Tripathi RD, Dwivedi S, Gupta DK (2006) Copper-induced oxidative stress and responses of antioxidants and phytochelatins in Hydrilla verticillata (L.f.) Royle. Aquatic Toxicol 80:405–415
Tamás L, Dudíková J, Ďurčeková K, Huttová J, Mistrík I, Zelinová V (2008) The impact of heavy metals on the activity of some enzymes along the barley root. Environ Exp Bot 62:86–91
Tari I, Szalai G, Lôrincz Z, Bálint A (2002) Changes in thiol content in roots of wheat cultivars exposed to copper stress. Biol Plant 45:255–260
Wong-ekkabut J, Xu Z, Triampo W, Tang I-M, Tieleman DP, Monticelli L (2007) Effect of lipid peroxidation on the properties of lipid bilayers: a molecular dynamics study. Biophys J 93:4225–4236
Yadav SK (2010) Heavy metals toxicity in plants: an overview on the role of glutathione and phytochelatins in heavy metal stress tolerance of plants. South Afr J Bot 76:167–179
Yagi K (1976) A simple fluorometric assay for lipoperoxide in blood plasma. Biochem Med 15:212–216
Acknowledgments
This work was supported by University of Łódź Grant No 506/819.
Author information
Authors and Affiliations
Corresponding author
Rights and permissions
About this article
Cite this article
Gajewska, E., Głowacki, R., Mazur, J. et al. Differential response of wheat roots to Cu, Ni and Cd treatment: oxidative stress and defense reactions. Plant Growth Regul 71, 13–20 (2013). https://doi.org/10.1007/s10725-013-9803-x
Received:
Accepted:
Published:
Issue Date:
DOI: https://doi.org/10.1007/s10725-013-9803-x