Abstract
Flooding is a major problem for soybean crop as it reduces the growth and grain yield. To investigate the function of the soybean cell wall in the response to flooding stress, cell wall proteins were analyzed. Cell wall proteins from roots and hypocotyls of soybeans, which were germinated for 2 days and subjected to 2 days of flooding, were purified, separated by two-dimensional polyacrylamide gel electrophoresis and stained with Coomassie brilliant blue. Sixteen out of 204 cell wall proteins showed responses to flooding stress. Of these, two lipoxygenases, four germin-like protein precursors, three stem 28/31 kDa glycoprotein precursors, and one superoxide dismutase [Cu–Zn] were downregulated. A copper amine oxidase was found to have shifted from the basic to acidic zone following flooding stress. Based on these results, it was confirmed by the lignin staining that the lignification was suppressed in the root of soybean under the flooding stress. These results suggest that the roots and hypocotyls of soybean caused the suppression of lignification through decrease of these proteins by downregulation of reactive oxygen species and jasmonate biosynthesis under flooding stress.
Similar content being viewed by others
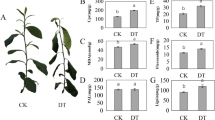
Avoid common mistakes on your manuscript.
Introduction
Flooding stress on plants is a widespread phenomenon in regions where soil is composed of an impermeable clay base or cracking gray clays with slow drainage, in areas of extreme rainfall, or inadequate land planning. Higher plants are generally aerobic organisms that die when oxygen availability is limited due to soil flooding (Voesenek et al. 2006). Flooding leads to reduced gas exchange between the plant tissues and the atmosphere as oxygen diffuses 10,000 times more slowly in water than in air (Armstrong 1979). Oxygen deprivation caused by flooding may be the main limiting factor for normal plant development, as well as the primary signal that triggers the response (Jackson and Colmer 2005). In previous gene expression studies, under low oxygen conditions, plants showed upregulated genes encoding transcription factors (Liu et al. 2005), signal transduction components (Baxter-Burrell et al. 2002), non-symbiotic hemoglobin (Dordas et al. 2004), ethylene signaling components (Reggiani 2006; Komatsu et al. 2009a), proteins of nitrogen metabolism (Mattana et al. 1994), and cell wall loosening (Saab and Sachs 1996; Komatsu et al. 2009a), as well as other proteins (Bailey-Serres and Voesenek 2008).
The cell wall in higher plants is the first compartment to respond to stress signals for transmission to the cell interior, subsequently affecting cell fate (Zhu et al. 2006). Increasing evidence has indicated continuous cross-talk between cell wall proteins and the cytoskeletal network (Shibaoka and Nagai 1994). Cell wall proteins play important roles in the cell wall structure, cell wall metabolism, cell enlargement, signal transduction, response to abiotic and biotic stresses, and numerous other physiological events (Zhu et al. 2006). Various cell wall proteins have been identified in Arabidopsis (Bayer et al. 2006; Minic et al. 2007), Medicago sativa (Watson et al. 2004), chickpea (Bhushan et al. 2006), maize (Zhu et al. 2006), and rice (Jung et al. 2008; Cho et al. 2009). Cell wall proteomics has become an attractive field due to the importance of cell wall proteins for maintenance of the cell structure and their responses to abiotic and biotic stresses (Somerville et al. 2004). Crop cell wall proteomics is of particular importance as it aims to improve crop productivity, especially under unfavorable environmental conditions. Although rapid advances in cell wall research have been reported (Jamet et al. 2006), the vast majority of research interest in plant cell walls has been directed toward the carbohydrate components for their structural role and commercial interest. The mechanisms underlying stress responses of cell wall proteins have thus remained a secondary objective (Bhushan et al. 2007).
According to interactive properties with the cell wall components, cell wall proteins may be categorized into three groups (Jamet et al. 2008): (1) the labile proteins that exhibit little or no interaction with cell wall components and thus move freely in the extracellular space, and are extracted in a low ionic strength buffer; (2) the weakly bound proteins that bind matrix via van der Waals interactions, hydrogen bonds, hydrophobic, or ionic interactions and that are extracted by salts; and (3) the strongly bound proteins that are not able to be released from the extracellular matrix. The accuracy of the proteomics analysis of a cell compartment strongly depends on the reliability of the isolation for the compartment of interest (Jamet et al. 2008). Two alternative isolation protocols have been used in cell wall proteomics to date. One protocol uses non-destructive techniques that allow the extraction of labile or weakly bound cell wall proteins without damage to the plasma membrane (Zhu et al. 2006), while the other uses destructive techniques that isolate either weakly or strongly bound cell wall proteins from the cell walls (Jamet et al. 2006).
Flooding is a major problem for soybean crops, as it reduces the growth and grain yield in many areas of the world. The mechanisms underlying low oxygen stress caused by flooding are not well understood. Using a proteomics approach, Hashiguchi et al. (2009) have shown that flooding stress involves not only hypoxic stress but also additional stresses from reduced access to light, disease, and water. We reported changes in expression of numerous proteins for nitrogen substitution, in a manner similar to flooding conditions, but not for protein destination or storage (Komatsu et al. 2010). We also reported the proteins located in the cell wall were up-regulated in plasma membrane of soybean under flooding stress using proteome technique (Komatsu et al. 2009b). In this study, to investigate the functions of the soybean cell wall in response to flooding stress, cell wall proteins were analyzed using proteomics technique. First, cell wall proteins were purified from the roots and hypocotyls of soybean seedlings, separated by two-dimensional polyacrylamide gel electrophoresis (2-DE), and then analyzed by mass spectrometry (MS) and protein sequencing. These techniques were used to analyze the cell wall proteins of soybean in response to flooding stress. It was also confirmed by the lignin staining whether the lignifications was controlled in the root of soybean under flooding stress.
Materials and methods
Plant growth and treatment
Soybean seeds (Glycine max L. cultivar Enrei) were sterilized in sodium hypochlorite solution, and germinated on sand under white fluorescent light (600 μmol m−2 s−1, 12-h light period) in a growth chamber maintained at 25°C and 70% relative humidity. For physiological analysis, soybean seeds germinated on sand for 2 days after sowing were submerged in tap water for 0, 1, 2, 3, or 4 days. Following the removal of tap water, the seeds were grown until the 14th day after sowing. The physiological parameters including fresh weight and length of the root and hypocotyl were measured for ten randomly selected seedlings. For protein analysis, soybean seeds germinated on sand for 2 days after sowing were submerged in tap water for 2 days. All experiments were biologically repeated three times.
Purification of CaCl2-extracted cell wall proteins
Cell wall purification and protein extraction were carried out as described by Feiz et al. (2006) and Kong et al. (2010) with some modifications. All purification and extraction methods were undertaken at 4°C. A portion (4 g) of roots and hypocotyls was collected and ground in a mortar and pestle. The ground tissue was homogenized in 125 mL of 5 mM acetate buffer (pH 4.6) containing 0.4 M sucrose and 1 mM phenylmethyl sulfonyl fluoride (PMSF). After the addition of 0.4 g polyvinyl polypyrrolidone (PVP), the mixture was incubated for 30 min with constant stirring. The cell walls were separated from the soluble cytoplasmic fluid by centrifugation of the homogenate at 1,000×g for 15 min. The pellet was suspended in 125 mL of 5 mM acetate buffer (pH 4.6) containing 0.6 M sucrose and centrifuged at 1,000×g for 15 min. The pellet was re-suspended in 125 mL of 5 mM acetate buffer (pH 4.6) containing 1 M sucrose and centrifuged twice at 1,000×g for 15 min each. The residue was then washed excessively with 750 mL of 5 mM acetate buffer (pH 4.6) with one layer of Miracloth (Merck, Darmstadt, Germany). The purified cell wall was extracted twice in 3 mL of 5 mM acetate buffer (pH 4.6) containing 0.2 M CaCl2 and 1 mM PMSF. The cell wall was re-suspended by vortexing for 5 min and centrifuged at 4,000×g for 15 min. The CaCl2-extracted cell wall proteins were dialyzed against deionized water overnight at 4°C.
Isolation of subcellular fractions
The isolation of cytosolic and membrane fractions was performed as described by Komatsu et al. (1997) with minor modifications. All isolation processes were undertaken at 4°C. A portion (4 g) of roots and hypocotyls was suspended in 1 mL homogenization buffer containing 0.25 M sucrose, 20 mM Tris–HCl (pH 7.5), 10 mM EGTA, 1 mM dithiothreitol and 1 mM PMSF, and homogenized using a mortar and pestle. The homogenate was then centrifuged at 3,000×g for 5 min, followed by ultracentrifugation at 259,000×g for 15 min (Beckman, Palo Alto, CA, USA) to collect the supernatant as the cytosolic fraction (S100). The pellet was re-suspended in 500 μL homogenization buffer, and washed by ultracentrifugation at 259,000×g for 15 min. After removal of the supernatant, the pellet was re-suspended in 50 μL of membrane solubilizing buffer containing 1% Triton X-100, 20 mM Tris–HCl (pH 7.5), 1 mM EDTA, and 50 mM 2-mercaptoethanol, and incubated for 30 min on ice. After centrifugation at 259,000×g for 8 min, the supernatant was collected as the membrane fraction (P100).
Extraction of total soluble proteins
All extraction processes were carried out at 4°C. A portion (200 mg) of roots and hypocotyls was homogenized with 1 mL of phosphate-buffered saline (pH 7.6) containing 65 mM K2HPO4, 2.6 mM KH2PO4, 400 mM NaCl, and 3 mM NaN3 in a mortar and pestle. The homogenate was then centrifuged twice at 15,000×g for 10 min, and the supernatant was collected as total soluble proteins.
Assay of glucose-6-phosphate dehydrogenase activity
The glucose-6-phosphate dehydrogenase (G6PDH) activity was assayed as described by Honjoh et al. (2003) with minor modifications. Briefly, the reaction was started by adding 100 μL protein solution to 2.9 mL of assay solution containing 55 mM Tris–HCl (pH 8.0), 3.3 mM MgCl2, 0.2 mM NADP, and 3.3 mM G6P. NADP reduction was monitored by the increase in absorbance at 340 nm in a double-beam recording spectrophotometer. One unit of G6PDH activity is defined as 1 μmol NADPH turnover per min at 25°C.
Concentration of proteins
Trichloroacetic acid was added to the solution of CaCl2-extracted cell wall proteins, cytosolic fraction, membrane fraction, and total soluble proteins to a final concentration of 10%. This solution was maintained on ice for 30 min and centrifuged at 15,000×g for 10 min (Zhong et al. 1997). The resultant precipitate was suspended in 100 μL lysis buffer (O’Farrell 1975) containing 8 M urea, 2% Nonidet P-40, 0.8% ampholine (pH 3.5–10) (GE Healthcare, Piscataway, NJ, USA), 5% 2-mercaptoethanol, and 5% PVP-40, and homogenized in a mortar and pestle. The homogenate was centrifuged twice at 15,000×g for 10 min, and the supernatant was used for 2-DE.
Analysis of protein content
Protein content was determined using the Bradford (1976) method with bovine serum albumin as the standard.
Separation of proteins using two-dimensional polyacrylamide gel electrophoresis
The protein (400 μg/50 μL) was separated by 2-DE in the first dimension in an isoelectric focusing tube gel (O’Farrell 1975) and in the second dimension by SDS-PAGE. The isoelectric focusing gel contained 8 M urea, 3.5% acrylamide, 2% Nonidet P-40, and 2% ampholine (pH 3.5–10 and 5–8). Electrophoresis was carried out at 200 V for 30 min, followed by 400 V for 16 h and 600 V for 1 h. After first dimension, SDS-PAGE was performed using a 15% polyacrylamide separating gel and a 5% polyacrylamide stacking gel. The gels were stained with Coomassie brilliant blue (CBB), and image analysis was performed.
Gel image analysis
The position of individual proteins on 2-DE gel evaluated automatically using PDQuest software (version 8.0, Bio-Rad, Hercules, CA, USA). The isoelectric point (pI) and molecular mass of each protein were calculated using PDQuest software with the position of a 2-DE marker (Bio-Rad) added in the 2-DE gel. The amount of a protein spot was estimated using PDQuest software, and expressed as the volume of the sample spot, which was defined as the sum of the intensities of all pixels comprising the spot. In order to correct the variability due to CBB staining and to reflect the quantitative variations in intensity of protein spots, the spot volumes were normalized as a percentage of the total volume of all of the spots present in the gel. After automated detection and matching, manual editing was carried out. Significantly changed spots were indentified according to the F test through analysis of variance (P < 0.05). Coefficient of variation was considered to confirm precision of the analysis. Proteins showed at least twofold differences in expression values compared to the control were considered as differentially expressed proteins and were subjected for analysis. These protein spots were selected for identification by protein sequencer or MS.
Analysis using protein sequencer and data analysis
To analyze N-terminal amino acid sequences following separation using 2-DE, the proteins were electroblotted onto a polyvinylidene difluoride membrane (Pall, Port Washington, NY, USA) using a semidry transfer blotter (Nippon-Eido, Tokyo, Japan) and detected by CBB staining. The stained protein spots were excised from the membrane and directly subjected to Edman degradation on a gas-phase protein sequencer (Procise cLC, Applied Biosystems, Foster City, CA, USA). The amino acid sequences obtained were compared with those of known proteins in the UNIPROT-SPROT database with Web-accessible search program FastA.
Protein preparation for mass spectrometry
Protein spots were excised from CBB-stained gels and destained with 50 mM NH4HCO3 for 1 h at 40°C. Proteins were reduced with 10 mM dithiothreitol in 100 mM NH4HCO3 for 1 h at 60°C, alkylated with 40 mM iodoacetamide in 100 mM NH4HCO3 for 30 min, and digested in 100 mM NH4HCO3 with 1 pM trypsin (Sigma-Aldrich, St. Louis, MO, USA) at 37°C overnight. The tryptic peptides were extracted from the gel grains three times with 0.1% trifluoroacetic acid in 50% acetonitrile. The procedure described above was performed with DigestPro (Intavis Bioanalytical Instruments AG, Cologne, Germany). The peptide solution obtained was dried and reconcentrated with 30 μL of 0.1% trifluoroacetic acid in 50% acetonitrile and desalted with NuTip C-18 pipette tips (Glygen, Columbia, MD, USA). Desalted peptide solution was analyzed by matrix-assisted laser desorption ionization time-of-flight MS (MALDI TOF/MS) and nano-liquid chromatography-tandem MS (nanoLC MS/MS).
Analysis using matrix-assisted laser desorption ionization time-of-flight mass spectrometry and data analysis
Desalted peptide solution was mixed with α-cyano-4-hydroxycinnamic acid. For analysis using MALDI TOF/MS (Voyager-DE RP, Applied Biosystems), calibration was external, and data were collected in the reflector mode. Data were compared to all entries in the NCBI database (release data 7 May 2008; 6,495,087 sequences) or the soybean genome sequence database (version 4; 62,199 sequences) that was especially constructed for this research based on soybean genome sequences from the Department of Energy (DOE), Joint Genome Institute and Soybean Genome Sequencing Consortium, using in-house licensed MASCOT software (version 2.2.18) (Matrix Science, London, UK). Soybean genome sequences were downloaded from the DOE database (http://www.phytozome.net) and converted into FASTA format.
Search parameters used fixed cysteine carbamidomethylation and variable methionine oxidation as modifications, peptide mass tolerance ±0.5 Da, fragments ions 1 Da, missed cleavages 1, and trypsin was specified as the proteolytic enzyme. Peptides were selected in the 500–4,000 Da mass range. For positive identification, the score result of [−10 × Log (P)] had to be over the significance (>72) threshold level (P < 0.05). Four criteria were used to assign a positive match with a known protein. These are as follows: (1) The deviation between the experimental and theoretical peptide masses should be less than 50 ppm. (2) At least ten different predicted peptide masses needed to match the observed masses for an identification to be considered valid. (3) The coverage of protein sequences by the matching peptides must reach a minimum of 15%. (4) The score that was obtained from the analysis with Mascot software indicates the probability of a true positive identification and must be at least 72. The positives matches were BLAST searched against the UniPort database (http://www.uniprot.org) and/or NCBI protein database (http://www.ncbi.nlm.nih.gov) for updated annotation and identification of homologous proteins.
Analysis using nano-liquid chromatography-tandem mass spectrometry and data analysis
Desalted peptide solution was analyzed using nanoLC MS/MS. A nanospray LTQ XL Orbitrap MS (ThermoFisher Scientific, Waltham, MA, USA) was operated in the data-dependent acquisition mode with Xcalibur software. Using Ultimate3000 nanoLC (Dionex, Germering, Germany), peptides were loaded in 0.1% formic acid onto a 300 μm ID × 5 mm C18 PepMap trap column. Elution of the peptides from the trap column and their separation on a 75 μm ID × 15 cm C18 PepMap100, 3 μm nanocolumn was undertaken using 0.1% formic acid in acetonitrile at a flow rate of 200 nL/min. To spray the sample into the MS, a PicoTip emitter (20 μm ID, 10 μm Tip ID, Woburn, MA, USA) was used with a spray voltage of 1.8 kV. Full scan mass spectra were acquired in the Orbitrap over 150–2,000 m/z with a resolution of 15,000. The three most intense ions at a threshold above 1,000 were selected for collision-induced fragmentation in the linear ion trap at a normalized collision energy of 35% after accumulation to a target value of 1,000. Dynamic exclusion was employed within 30 s to prevent repetitive selection of the peptides.
Acquired MS/MS spectra were converted to single DTA files using BioWorks software (version 3.3.1) (Thermo Fisher Scientific). The following parameters were set for creation of the peak lists: (1) parent ions in the mass range with no limitation; (2) one grouping of MS/MS scans; and (3) threshold at 100. Precursor ion tolerance was 10 ppm. Data were searched using the MASCOT software against all entries in the NCBI or DOE database described in the section of MALDI TOF/MS. Carbamidomethylation of cysteines was set as a fixed modification and oxidation of methionine was set as a variable modification. Trypsin was specified as the proteolytic enzyme, and one missed cleavage was allowed. Parameters for search were peptide mass tolerance 10 ppm, fragment mass tolerance 0.2 Da, maximum missed cleavages 1, peptide charges +1, +2, +3, variable modification methylation, and fixed modification carbamidomethylation. The proteins with at least ten matched peptides in the Mascot search results with more than 15% sequence coverage were considered. The proteins with ion scores greater than 41 and 20 were significant for NCBI and soybean genome sequence databases, respectively (P < 0.05).
RNA extraction and quantitative RT-PCR
Total RNA was extracted from a portion (100 mg) of hypocotyls and roots of soybean seedlings using an RNeasy plant mini kit with DNase (Qiagen, Valencia, CA, USA). A portion (800 ng) of total RNA from each sample was reverse-transcribed to cDNA in a 20-μL reaction volume using iScript cDNA synthesis kit (Bio-Rad) according to the manufacturer’s protocol. Real-time quantitative PCR was performed using the cDNA product corresponding to 20 ng of total RNA in a 20-μL reaction volume using the iQ SYBR Green supermix (Bio-Rad) on a MyiQ single-color real-time PCR detection system (Bio-Rad). The PCR conditions were as follows: 95°C for 3 min, then 45 cycles of 95°C for 30 s, 58°C for 30 s, and 72°C for 30 s. To normalize gene expression, 18S rRNA (X02623) was used as an internal control. A dilution series of an 18S rRNA cDNA clone was used as a template to produce a standard curve. The relative amount of mRNA was calculated by the ΔCt method. The primers were designed using the Primer3 web interface (http://frodo.wi.mit.edu/) (Rozen and Skaletsky 2000).
The staining of lignin
Roots and hypocotyls of soybean were manually cut at 10 mm from root tip to some sections which were 100 μm thick with cutter, and the sections were stained for 5 min with 1% Phloroglucine in 50% ethanol and 50% acidium hydrochloricum. The sections were then observed using microscope (Speer 1987).
Identification of function and localization, and/or theoretical molecular mass
The presence of a signal peptide sequence in the identified proteins was predicted using iPSORT (http://hc.ims.u-tokyo.ac.jp/iPSORT/) and TargetP (http://www.cbs.dtu.dk/services/TargetP/). Non-classical secretory proteins were predicted using SecretomeP (http://www.cbs.dtu.dk/services/SecretomeP/). The comparison of the functional classifications in the identified proteins was classified according to Bevan et al. (1998). Relative molecular mass calculated from the matched peptide sequence in the MASCOT search engine, or Compute pI/Mw tool of ExPASy (http://us.expasy.org/tools/pi_tool.html).
Results
Comparative proteomics between cell wall proteins and total soluble proteins identified by proteomics technique
Roots and hypocotyls were collected from 4-day-old soybean seedlings, and subsequently CaCl2-extracted cell wall proteins were isolated via sucrose gradients and washing using the method of Feiz et al. (2006) and Kong et al. (2010). To validate the purification method, the activity of G6PDH, which is a typical marker enzyme used to detect contamination of cytoplasmic proteins (Kwon et al. 2005), was measured. The G6PDH activity in the CaCl2-extracted cell wall protein, total soluble protein, membrane protein (P100), and cytosolic protein (S100) fractions were 10.4, 75.8, 10.2, and 122 mU/mg protein, respectively (Fig. 1). The G6PDH activity (Li et al. 1989) in the CaCl2-extracted cell wall proteins was 12 times lower than that in the cytosolic proteins (S100). This result shows that specific activities of G6PDH in the CaCl2-extracted cell wall protein fraction contained 8.52% of the activity of those from the cytosolic extracts. It was indicated that the contamination of intracellular proteins was minimal using this purification method.
Evaluation of purity by the G6PDH activity in the cell wall proteins isolated from the roots and hypocotyls of soybean seedlings. The activity of G6PDH was assayed in CaCl2-extracted cell wall proteins, total soluble proteins, membrane proteins (P100), and cytosolic proteins (S100) from the roots and hypocotyls of 4-day-old soybean seedlings. One unit of G6PDH activity is defined as 1 μmol of NADPH turnover per min/mg protein. Results are presented as mean ± SE of G6PDH activity from three biological replicates. The asterisks indicate significant differences in the G6PDH activity of CaCl2-extracted cell wall proteins, total soluble proteins, and membrane proteins (P100) compared to that of cytosolic proteins (S100) (*P < 0.05; **P < 0.01)
CaCl2-extracted cell wall proteins purified from the roots and hypocotyls of 4-day-old soybean seedlings were separated by 2-DE and stained with CBB. Comparison of the 2-DE gel patterns for the total soluble proteins and the CaCl2-extracted cell wall proteins showed 423 and 204 protein spots for the total soluble proteins and CaCl2-extracted cell wall proteins, respectively (Fig. 2a). Following purification of the cell wall, more than 219 proteins present in the total soluble protein fraction were removed from the CaCl2-extracted cell wall protein fraction, and then concentrated. To analyze the profiles of the CaCl2-extracted cell wall and total soluble proteins, protein sequencer and nanoLC–MS/MS and/or MALDI-TOF MS were used (Supplemental Tables 1, 2). A total of 95 and 59 proteins were identified for the total soluble proteins (Supplemental Table 1) and CaCl2-extracted cell wall proteins (Supplemental Table 2), respectively. Although their levels of accumulation were very different, trypsin inhibitor and stem 31 kDa glycoprotein were identified in both the total soluble protein and CaCl2-extracted cell wall protein fractions (Fig. 2a, white circle).
2-DE patterns and the presence of signal peptide sequences, and the subcellular localization of total soluble and cell wall proteins. a 2-DE gels for total soluble and cell wall proteins isolated from the roots and hypocotyls of soybean seedlings. Total soluble and CaCl2-extracted cell wall proteins were prepared from roots and hypocotyls of 4-day-old soybean seedlings. Extracted proteins (400 μg) were separated by 2-DE and stained with CBB. The pI and molecular mass of each protein were determined using a 2-DE marker (Bio-Rad). The circles indicate the same spots on the two gels. b, c The presence of signal peptide sequences, and the subcellular localization of total soluble and cell wall proteins. The total soluble and CaCl2-extracted cell wall proteins on the 2-DE gels were identified using protein sequencing and MS. The presence of a signal peptide sequence (b) in the identified proteins (Supplemental Tables 1, 2) was predicted using iPSORT and TargetP. Non-classical secretory proteins were predicted using SecretomeP. Mito and Chlor show mitochondrion and chloroplast, respectively. The comparison of the functional classifications (c) in the identified proteins was classified according to Bevan et al. (1998). M metabolism, D defense, PD protein destination/storage, PS protein synthesis, SM secondary metabolism, ST signal transduction, T transcription, Un unclassified. The total number of total soluble proteins and of CaCl2-extracted cell wall proteins was 95 and 59, respectively
To understand the functions of the identified proteins, the CaCl2-extracted cell wall and total soluble proteins were analyzed for signal peptides (Fig. 2b). The signal peptides present among the total soluble proteins and CaCl2-extracted cell wall proteins were predicted using the iPSORT and TargetP programs, and non-classical secretory proteins were predicted using the SecretomeP program (Fig. 2b). The presence of a secretory pathway peptide was predicted for 54% (32 of the 59) of the CaCl2-extracted cell wall proteins, but only 26% (25 of the 95) of the total soluble proteins. In the CaCl2-extracted cell wall proteins, 46% of the proteins did not have a secretory signal peptide sequence, while 23% of the proteins were predicted to be secretory proteins. For example, although methionine synthase, superoxide dismutase Cu–Zn, and lipoxygenase did not demonstrate secretory signal peptides in this study (Supplemental Tables 1, 2), the existence of these proteins in the cell wall has been previously reported by Ravanel et al. (1998), Kim et al. (2008), and Pavelic et al. (2000), respectively. The remaining 23% of unidentified proteins could be cell wall proteins; however, the current protein identification process is limited in distinguishing proteins of the same family.
To extend our understanding of the functions exhibited by the identified proteins, CaCl2-extracted cell wall proteins and total soluble proteins were classified into functional categories based on the methods reported by Bevan et al. (1998) (Fig. 2c). Mainly, proteins of soluble and cell wall were classified in metabolism (14 and 17%) and defense (28 and 22%), respectively. Moreover, more CaCl2-extracted cell wall proteins (27%) than total soluble proteins (12%) were characterized as having a role in protein destination/storage. Total soluble proteins that function in secondary metabolism (3%) and in signal transduction (4%) were even less abundantly expressed. In fact, analysis of the identified proteins indicated significant differences in the profiles of the CaCl2-extracted cell wall and total soluble proteins (Supplemental Tables 1, 2). For example, β-amylase which functions in metabolism and copper amino oxidase which functions in defense/disease were only identified in the CaCl2-extracted cell wall fraction, while phosphoglucomutase which functions in metabolism and calreticulin which functions in defense/disease were identified only in the total soluble proteins.
Comparative proteomics analysis of differentially expressed proteins in soybean cell wall during flooding stress
In this study, the physiological changes that occur during the stages immediately following flooding were investigated to measure the initial effects of the stress. Soybean seeds were germinated on sand for 2 days and flooded with tap water for 0, 1, 2, 3, or 4 days. The tap water was then removed, and the seedlings were allowed to develop until the 14th day after sowing. Physiological parameters, including fresh weight of the roots and shoot length, were measured (Fig. 3). The results demonstrated that flooding stress affected the growth of soybean seedlings after 2 days of treatment (Fig. 3a). In particular, flooding stress affected the length of the hypocotyls after 3 days of treatment and fresh weight of the roots after 2 days of treatment (Fig. 3b). Shi et al. (2008) reported that the total number of roots, the length of the main root, the length of the lateral and adventitious roots, and the fresh weight of the roots of flooded soybean seedlings were significantly suppressed after 3 days of flooding stress compared with untreated plants. Hashiguchi et al. (2009) also reported that the effects of flooding stress on soybean seedlings were obvious in every aspect of growth under stress longer than 1 day. The reduction in root growth is one of the most commonly reported parameters during flooding (Wang and Jiang 2007). Therefore, soybean seedlings that had been subjected to flooding stress for 2 days were examined to investigate the cell wall proteome.
Growth suppression of soybean seedlings after flooding. Soybean seeds were germinated on sand for 2 days and flooded with tap water for 0, 1, 2, 3, or 4 days. The tap water was then removed, and the seedlings were allowed to develop until the 14th day after sowing. Physiological parameters, including fresh weight of roots and shoot length containing hypocotyls, epicotyls, and second internode, were then measured. Ten seedlings were randomly selected for measurement, and the experiments were repeated three times. Asterisks indicate significant differences between control and flooding treatment (*P < 0.05; **P < 0.01)
To investigate mechanisms regulated by flooding stress in soybean seedlings, flooding-responsive cell wall proteins were identified using a proteomics technique. Soybean seeds were germinated for 2 days and subjected to flooding for 2 days. The cell wall proteins were purified from the roots and hypocotyls, separated by 2-DE and stained with CBB (Fig. 4a). After the analysis by PDQuest software (Fig. 4b), proteins were identified by protein sequencer and nanoLC–MS/MS (Table 1; Supplemental Table 3). A total of 204 protein spots were detected, and the pI and molecular mass of these proteins ranged from 3.5 to 9.0 and 10.0 to 100.0 kDa, respectively (Fig. 4a). Among these protein spots, 16 were altered following flooding. Of the identified proteins, 4 and 12 proteins were upregulated and downregulated, respectively (Fig. 4b). The upregulated proteins included three methionine synthases (spots 1, 2, and 16), and one copper amine oxidase (spot 3). The downregulated proteins included two lipoxygenases (spots 5 and 10), four germin-like protein precursors (spots 6, 7, 11, and 12), three stem 31 kDa glycoprotein precursors (spots 9, 13, and 14), one Cu–Zn-superoxide dismutase (spot 8), one copper amine oxidase (spot 4), and one unknown protein (spot 15). It was found that one copper amine oxidase was shifted from the basic (spot 4) to the acidic zone (spot 3) following flooding stress.
Effects on cell wall proteins located in the roots and hypocotyls of soybean seedlings following flooding stress. Soybean seeds were germinated on sand for 2 days and subjected to flooding for 2 days or left as untreated controls. Protein (400 μg) was separated by 2-DE and stained with CBB (a). Open circles show spots with altered expression. The upward arrows indicate upregulation, and the downward arrows indicate downregulation. Spot numbers are the same as Table 1. The differentially expressed proteins were quantified using PDQuet software (b). Results are presented as mean ± SE of relative protein intensity for gels from three biological replicates. White and black bars represent control and flooding treatment, respectively. The indicated proteins show the spots with significant differences in protein expression between control and flooding treatment (*P < 0.05; **P < 0.01)
Developmental stage specificity at the mRNA level of methionine synthase
Based on proteomics research, only methionine synthase was upregulated. To identify whether methionine synthase genes coding proteins are expressed, RNA expression levels were examined. Two-day-old soybean seedlings were treated for 1 or 2 days by flooding stress. Total RNA was extracted from hypocotyls and roots of soybean seedlings, and quantitative real-time PCR was performed (Fig. 5). Alcohol dehydrogenase, which is over-expressed under flooding stress (Komatsu et al. 2009a), was used as a positive control. Methionine synthase did not express under flooding stress (Fig. 5, top) though alcohol dehydrogenase expressed remarkably (Fig. 5, bottom).
Relative amount of mRNA for flooding responsive genes in hypocotyls and roots of soybean seedlings. Two-day-old soybean seedlings were subjected to flooding for 1 and 2 days. Total RNA was extracted from hypocotyls and roots of soybean seedlings after flooding stress. cDNA was synthesized by reverse transcription, and quantitative real-time PCR for alcohol dehydrogenase (top) and methionine synthase (bottom) was performed using the cDNA products. An 18S rRNA cDNA clone was used as a template to produce a standard curve, and the relative amount of mRNA was calculated by the ΔCt method. Values are the mean ± SE from three independent experiments, and asterisks indicate significant (P < 0.01) differences between control (white columns) and flooding stress (black columns)
Reducing lignification of cell walls in soybean by flooding stress
Lignification was proven with phloroglucinol staining that detects lignin (Fig. 6). Two-day-old soybean seedlings were subjected to flooding for 2 days, and the section of root were stained with Phloroglucine and acidium hydrochloricum. The reduced lignification was detected at the surface and inside of the tissue treated (Fig. 6, bottom) when compared with control (Fig. 6, top).
The pattern of lignin staining of the root of soybean seedlings following flooding stress. Two-day-old seedlings were subjected to flooding for 2 days or left as untreated controls. Root of soybean was cut manually to some sections which are about 100 μm thick with cutter, and the sections were stained for Phloroglucine and acidium hydrochloricum. The sections were observed using microscope. The bar shows the length of 1 mm. White star marks show the stained parts by Phloroglucine
Discussion
To investigate the function of the cell wall in soybean under flooding, we used a proteome-based approach. In this study, CaCl2-extracted cell wall proteins were purified from the roots and hypocotyls of 4-day-old soybean seedlings using a previously reported destructive method (Feiz et al. 2006; Kong et al. 2010). With the aid of a sucrose gradient and extensive washing in low ionic strength acidic buffer, the vast majority of the intracellular proteins were removed from the cell wall using this procedure. Of the identified cell wall proteins, 4 and 12 proteins were upregulated and downregulated, respectively, under flooding stress (Fig. 4).
The shifted copper amine oxidase (spots 3 and 4) also contained secretory pathway signal peptides. Copper amine oxidase has been implicated in both plant development and defense responses previously (Yoda et al. 2003). Reactive oxygen species, including superoxide radicals, hydroxyl radicals, and hydrogen peroxide, also play a dual role in plants, where they act as a toxin against normal cell metabolism and as a regulatory molecule in stress perception and signal transduction (Navrot et al. 2006). Considering their extracellular localization, the hydrogen peroxide-generating enzymes may act in combination with peroxidases to mediate protein cross-linking and lignin deposition in plant cell walls under mechanical or physiological stress. The report by Rea et al. (2004) demonstrated no significant changes in lignification levels in transgenic tobacco expressing copper amine oxidase compared to untransformed plants, suggesting that the effects of copper amine oxidase on lignification are not transcriptional, but rather involve post-translational modification such as phosphorylation in the cell wall, which is supported by our finding that copper amine oxidase was shifted from the basic to the acidic zone following flooding stress.
Although the superoxide dismutase [Cu–Zn] (spot 8) was found to be downregulated in the cell wall fraction following flooding stress, this protein did not contain secretory pathway signal peptides. Despite superoxide dismutase [Cu–Zn] no having secretory signal peptides based on a motif search, this protein was found to be located in the cell wall fraction in this study. Kim et al. (2008) reported that superoxide dismutase [Cu–Zn] was localized to the secondary cell walls of developing cotton fibers using immuno-gold localization analysis, suggesting that this protein may be localized to the cell wall of soybean. Reactive oxygen species scavengers, including 1-Cys peroxiredoxin, ascorbate peroxidase, glutathione peroxidase, and superoxide dismutase, function as stress indicators that are induced by various stress signals. Ascorbate peroxidase was downregulated following flooding stress in soybean seedlings (Shi et al. 2008). In this study, we also found that superoxide dismutase [Cu–Zn] was downregulated. Kim et al. (2008) and Karlsson et al. (2005) have shown that extracellular superoxide dismutase [Cu–Zn] was involved in cell wall growth and development. In addition, Ogawa et al. (1997) reported that the generation of superoxide anions and hydrogen peroxide in the vascular tissue was particularly apparent in the xylem, and was associated with the distribution of superoxide dismutase [Cu–Zn] as determined using immunohistochemistry and using the phloroglucin-HCl reaction. Although the relationship between superoxide dismutase activity and lignification processes has not been established, our results suggest that the downregulation of superoxide dismutase [Cu–Zn] may play an essential role in the regulation of lignification under flooding stress.
Four germin-like protein precursors (spots 6, 7, 11, and 12) were downregulated following flooding and contained secretory pathway signal peptides. These proteins have also been identified in the cell wall of tobacco (Millar et al. 2009) and wheat (Domon et al. 1995), and are expressed at specific stages of plant development (Dunwell et al. 2000). Of the four germin-like protein precursors identified, the largest group with known functions was associated with cell wall formation and the lignin biosynthetic pathway. This result is consistent with the fact that the pine xylem is characterized by large cell walls (Allona et al. 1998). Furthermore, Soares et al. (2009) reported that proteins mobilized within the reactive oxygen species-dependent pathway were largely ionically bound to the cell wall and included superoxide dismutase, peroxidases, and germin-like proteins, suggesting their involvement in wound-activated, reactive oxygen species regulatory loops. In addition, Ke et al. (2009) reported that germin-like protein was significantly dephosphorylated during drought stress. In this study, although four germin-like proteins were identified, only two were likely to have been phosphorylated, as acidic germin-like proteins have the same molecular weights as the basic germin-like proteins. Germin-like proteins may be related to lignification in the cell wall under flooding stress.
Two lipoxygenases (spots 5 and 10) downregulated under flooding were not associated with secretory pathway signal peptides. Although lipoxygenase did not contain secretory signal peptides based on a motif search, this protein was found in the cell wall fraction in this study. Wang et al. (2005) reported that lipoxygenase was located in the cell wall of Haenatococcus pluvialis using proteomics analysis, indicating that this protein may also be localized to the cell wall in soybean. In plants, products of lipoxygenase are known to be involved in the defense mechanisms that protect against drought, mechanical damage, temperature stress, and potential pathogens. The first step in the lipoxygenase pathway involves a reaction between linoleic or linolenic acid with molecular oxygen, through the catalysis lipoxygenase. This reaction results in the formation of hydroperoxy fatty acids that are highly reactive and dangerous for plant growth. As a result, these products are further metabolized by additional enzymes such as allene oxide synthase, hydroperoxide lyase, peroxygenase, or divinyl ether synthase (Noordermeer et al. 2001). The activity of catalase and superoxide dismutase was not shown to be significantly affected, whereas the amount and activity of lipoxygenase tended to increase on the membrane lipids during periods of anoxia (Pavelic et al. 2000), mainly due to lipoxygenase, while the contribution of reactive oxygen species is negligible. However, our results indicated that lipoxygenase was downregulated in the cell wall under flooding stress, which is in contrast to a study by Wang et al. (2005) that reported upregulation of lipoxygenase in cell wall-deficient H. pluvialis mutants that had an altered cell wall morphology and structure. These findings suggest that the downregulation of lipoxygenase directly affects cell wall metabolism under flooding stress, but not stress caused by anoxia.
Three stem 28/31 kDa glycoprotein precursors (spots 9, 13, and 14) were also downregulated by flooding stress, and contained secretory pathway signal peptides. The stem 28/31 kDa glycoproteins, which are soybean vegetative storage proteins, have been found to accumulate at high levels in the leaves of soybean (Staswick 1988). In addition, Creelman et al. (1992) reported that wounded soybean stems rapidly accumulated jasmonic acid and methyl jasmonate. They also reported that the addition of methyl jasmonate to soybean suspension cultures increased mRNA levels of soybean vegetative storage proteins, chalcone synthase, and proline-rich cell wall proteins. Jasmonate regulates plant wound and defense responses, pollen development, and stress-related growth inhibition. Soybean vegetative storage protein is induced by stresses, including drought and high salt levels (Gong et al. 2001). Ithal et al. (2007) suggested that the cell wall modification process appeared to be tightly controlled and coordinately regulated with cell wall rigidification processes that may be involved in lignification of feeding cell walls. These authors also showed local downregulation of jasmonic acid biosynthesis and response in developing syncytia, suggesting a local suppression of plant defense mechanisms. These results indicated that soybean vegetative storage protein was downregulated in the cell wall, further suggesting that the suppression of lignification is caused by downregulation of jasmonate biosynthesis following flooding stress.
Although methionine synthase (spots 1, 2, and 16) did not contain secretory signal peptides based on our motif search, this protein was found to be located in the cell wall fraction in this study. Bhushan et al. (2007) previously reported that methionine synthase was localized to the extracellular matrix in chickpea exposed to dehydration using proteomics analysis, indicating that this protein may also be localized to the cell wall of soybean. In this study, the experimental molecular weight of methionine synthase was lower than the theoretical molecular weight (Table 1), and the RNA expression experiment was confirmed that these proteins did not express under flooding (Fig. 5). A further experiment might be necessary for clarifying this reason.
The plant cell wall is the first compartment that senses stress signals, transmits these signals to the cell interior, and eventually influences cell fate. Proteomics analysis provides a novel approach for identifying proteins and pathways that play a critical role in the response to flooding stress in plants. In this study, CaCl2-extracted cell wall proteins were purified from the roots and hypocotyls during the early stages of soybean seedling development. However, this method failed to purify expansin and xyloglucan endotransglycosylase/hydrolases, which are well established cell wall-loosening proteins (Darley et al. 2001). In the cell wall, non-enzymatic excision of xyloglucan in the presence of hydrogen peroxide, copper, and ascorbate leads to cell wall loosening (Fry 1998; Fry et al. 2002). In contrast, the germins/germin-like proteins prevent cell expansion by increasing the links between polysaccharides and proteins within the cell wall, as well as favoring lignification (Dunwell et al. 2000). In combination, these results suggest that under flooding stress, the roots and hypocotyls of soybean respond through signaling cascades that result in downregulation of lignification caused by a decline in the reduction of hydrogen peroxide and ascorbate, or by the reduction in linking of polysaccharides.
Finally, it was also confirmed by the lignin staining that the lignification was suppressed in the root of soybean under the flooding stress compared with that of control plant (Fig. 6). Simone et al. (2003) reported that suberin, and not lignin, represented the barrier for the diffusion of oxygen. On the other hand, lignified secondary cell walls provide significant compressive strength relative to non-lignified walls (Sperry 2003). So, lignin has been shown to be essential to cell wall integrity. This is likely due to the finding that additional proteins for lignification, such as lipoxygenases, germin-like protein precursor, stem 31 kDa glycoprotein, and superoxide dismutase [Cu–Zn], are downregulated by flooding stress. These results suggest that the roots and hypocotyls of soybean caused the suppression of lignification through decrease of these proteins by downregulation of reactive oxygen species and jasmonate biosynthesis under flooding stress.
Abbreviations
- 2-DE:
-
Two-dimensional polyacrylamide gel electrophoresis
- MS:
-
Mass spectrometry
- CBB:
-
Coomassie brilliant blue
- pI:
-
Isoelectric point
- PMSF:
-
Phenylmethyl sulfonyl fluoride
- PVP:
-
Polyvinylpolypyrrolidone
- G6PDH:
-
Glucose-6-phosphate dehydrogenase
References
Allona I, Quinn M, Shoop E, Swope K, St Cyr S, Carlis J, Riedl J, Retzel E, Campbell MM, Sederoff R, Whetten RW (1998) Analysis of xylem formation in pine by cDNA sequencing. Proc Natl Acad Sci USA 95:9693–9698
Armstrong W (1979) Aeration in higher plants. Adv Bot Res 7:225–232
Bailey-Serres J, Voesenek LACJ (2008) Flooding stress: acclimations and genetic diversity. Ann Rev Plant Biol 16:313–339
Baxter-Burrell A, Yang Z, Springer PS, Bailey-Serres J (2002) RopGAP4-dependent Rop GTPase rheostat control of Arabidopsis oxygen deprivation tolerance. Science 296:2026–2028
Bayer EM, Bottrill AR, Walshaw J, Vigouroux M, Naldrett MJ, Thomas CL, Maule AJ (2006) Arabidopsis cell wall proteome defined using multidimensional protein identification technology. Proteomics 6:301–311
Bevan M, Bancroft I, Bent E et al (1998) Analysis of 1.9 Mb of contiguous sequence from chromosome 4 of Arabidopsis thaliana. Nature 391:485–488
Bhushan D, Pandey A, Chattopadhyay A, Choudhary MK, Chakraborty S, Datta A, Chakraborty N (2006) Lular matrix proteome of chickpea (Cicer arietinum L.) illustrates pathway abundance, novel protein functions and evolutionary perspect. J Proteome Res 5:1711–1720
Bhushan D, Pandey A, Choudhary MK, Datta A, Chakraborty S, Chakraborty N (2007) Comparative proteomics analysis of differentially expressed proteins in chickpea extracellular matrix during dehydration stress. Mol Cell Proteomics 6:1868–1884
Bradford MM (1976) A rapid and sensitive method for the quantitation of microgram quantities of protein utilizing the principle of protein-dye binding. Anal Biochem 72:248–254
Cho WK, Chen XY, Chu H, Rim Y, Kim S, Kim ST, Kim SW, Park Z-Y, Kim J-Y (2009) Proteomic analysis of the secretome of rice calli. Physiol Plant 135:331–341
Creelman RA, Tierney ML, Mullet JE (1992) Jasmonic acid, ethyl jasmonate accumulate in wounded soybean hypocotyls and modulate wound gene expression. Proc Natl Acad Sci USA 89:4938–4941
Darley CP, Forrecster AM, McQueen-Mason SJ (2001) The molecular basis of plant cell wall extension. Plant Mol Biol 47:179–195
Domon JM, Dumas B, Lain E, Meyer Y, David A, David H (1995) Three glycosylated polypeptides secreted by several embryogenic cell cultures of pine show highly specific serological affinity to antibodies directed against the wheat germin apoprotein monomer. Plant Physiol 108:141–148
Dordas C, Hasinoff BB, Rivoal J, Hill RD (2004) Class-1 hemoglobins, nitrate and NO levels in anoxic maize cell-suspension cultures. Planta 219:66–72
Dunwell JM, Khuri S, Gane PJ (2000) Microbial relatives of the seed storage proteins of higher plants: conservation of structure and diversification of function during evolution of the cupin superfamily. Microbiol Mol Biol Rev 64:153–179
Feiz L, Irshad M, Pont-Lezica RF, Canut H, Jamet E (2006) Evaluation of cell wall preparation for proteomics: a new procedure for purifying cell walls from Arabidopsis hypocotyls. Plant Methods 2:10
Fry SC (1998) Oxidative scission of plant cell wall polysaccharides by ascorbate-induced hydroxyl radicals. Biochem J 332:507–515
Fry SC, Miller J, Dumville J (2002) A proposed role for copper ions in cell wall loosening. Plant Soil 247:57–67
Gong Z, Koiwa H, Cushman MA, Ray A, Bufford D, Kore-eda S, Matsumoto TK, Zhu J, Cushman JC, Bressan RA, Hasegawa PM (2001) Genes that are uniquely stress regulated in salt overly sensitive (sos) mutants. Plant Physiol 126:363–375
Hashiguchi A, Sakata K, Komatsu S (2009) Proteome analysis of early-stage soybean seedlings under flooding stress. J Proteome Res 8:2058–2069
Honjoh K, Mimura A, Kuroiwa E, Hagisako T, Suga K, Shimizu H, Dubey RS, Miyamoto T, Hatano S, Iio M (2003) Purification and characterization of two isoforms of glucose 6-phosphate dehydrogenase from Chlorella vulgaris C-27. Biosci Biotech Biochem 67:1888–1896
Ithal N, Recknor J, Nettleton D, Maier T, Baum TJ, Mitchum MG (2007) Developmental transcript profiling of cyst nematode feeding cells in soybean roots. Mol Plant Microbe Interact 20:510–525
Jackson MB, Colmer TD (2005) Response and adaptation by plants to flooding stress. Ann Bot 96:501–505
Jamet E, Canut H, Boudart G, Pont-Lezica R (2006) Cell wall proteins: a new insight through proteomics. Trends Plant Sci 11:33–39
Jamet E, Albenne C, Boudart G, Irshad M, Canut H, Pont-Lezica R (2008) Recent advances in plant cell wall proteomics. Proteomics 8:893–908
Jung YH, Jeong SH, Kim SH, Singh R, Lee JE, Cho YS, Agrawal GK, Rakwal R, Jwa NS (2008) Systematic secretome analyses of rice leaf and seed callus suspension-cultured cells: workflow development and establishment of high-density two-dimensional gel reference maps. J Proteome Res 7:5187–5210
Karlsson M, Melzer M, Prokhorenko I, Johansson T, Wingsle G (2005) Hydrogen peroxide and expression of hipI-superoxide dismutase are associated with the development of secondary cell walls in Zinnia elegans. J Exp Bot 56:2085–2093
Ke Y, Han G, He H, Li J (2009) Differential regulation of proteins and phosphoproteins in rice under drought stress. Biochem Biophys Res Commun 379:133–138
Kim HJ, Kato N, Kim S, Triplett B (2008) Cu/Zn superoxide dismutases in developing cotton fibers: evidence for an extracellular form. Planta 228:281–292
Komatsu S, Karibe H, Masuda T (1997) Effect of abscisic acid on phosphatidylserine-sensitive calcium dependent protein kinase activity and protein phosphorylation in rice. Biosci Biotech Biochem 61:418–423
Komatsu S, Yamamoto R, Nanjo Y, Mikami Y, Yunokawa H, Sakata K (2009a) A comprehensive analysis of the soybean genes and proteins expressed under flooding stress using transcriptome and proteome techniques. J Proteome Res 8:4766–4778
Komatsu S, Wada T, Yann A, Nouri MZ, Nanjo Y, Nakayama N, Shimamura S, Yamamoto R, Nakamura T, Furukawa K (2009b) Analysis of plasma membrane proteome in soybean and application to flooding stress response. J Proteome Res 8:4487–4499
Komatsu S, Sugimoto T, Hoshino T, Nanjo Y, Furukawa K (2010) Identification of flooding stress responsible cascades in root and hypocotyls of soybean using proteome analysis. Amino Acids 38:729–738
Kong FJ, Oyanagi A, Komatsu S (2010) Cell wall proteome of wheat roots under flooding stress using gel-based and LC MS/MS-based proteomics approaches. Biochim Biophys Acta 1804:124–136
Kwon HK, Yokoyama R, Nishitani K (2005) A proteomic approach to apoplastic proteins involved in cell wall regeneration in protoplasts of Arabidopsis suspension-cultured cell. Plant Cell Physiol 46:843–857
Li ZC, McClure JW, Hagerman AE (1989) Soluble and bound apoplastic activity for peroxidase, beta-d-glucosidase, malate dehydrogenase, and nonspecific arylesterase, in barley (Hordeum vulgare L.) and oat (Avena sativa L.) primary leaves. Plant Physiol 90:185–190
Liu F, Vantoai T, Moy L, Bock G, Linford LD, Quackenbush J (2005) Global transcription profiling reveals novel insights into hypoxic response in Arabidopsis. Plant Physiol 137:1115–1129
Mattana M, Coraggio I, Bertani A, Reggiani R (1994) Expression of the enzymes of nitrate reduction during the anaerobic germination of rice. Plant Physiol 106:1605–1608
Millar DJ, Whitelegge JP, Bindschedler LV, Rayon C, Boudet AM, Rossignol M, Borderies G, Bolwell GP (2009) The cell wall and secretory proteome of a tobacco cell line synthesising secondary wall. Proteomics 9:2355–2372
Minic Z, Jamet E, Négroni L, Arsene der Garabedian P, Zivy M, Jouanin L (2007) A sub-proteome of Arabidopsis thaliana mature stems trapped on Concanavalin A is enriched in cell wall glycoside hydrolases. J Exp Bot 58:2503–2512
Navrot N, Collin V, Gualberto J, Gelhaye E, Hirasawa M, Rey P, Knaff DB, Issakidis E, Jacquot JP, Rouhier N (2006) Plant glutathione peroxidase are functional peroxiredixins distributed in several subcellular compartments and regulated during biotic and abiotic stresses. Plant Physiol 142:1364–1379
Noordermeer MA, Veldink GA, Vliegenthart JF (2001) Fatty acid hydroperoxide lyase: a plant cytochrome p450 enzyme involved in wound healing and pest resistance. Chembiochem 2:494–504
O’Farrell PH (1975) High resolution two-dimensional electrophoresis of protein. J Biol Chem 250:4007–4021
Ogawa K, Kanematsu S, Asada K (1997) Generation of superoxide anion and localization of CuZn-superoxide dismutase in the vascular tissue of spinach hypocotyls: their association with lignification. Plant Cell Physiol 38:1118–1126
Pavelic D, Arpagaus S, Rawyler A, Brandle R (2000) Impact of post-anoxia stress on membrane lipids of anoxia-pretreated potato cells. A re-appraisal. Plant Physiol 124:1285–1292
Ravanel S, Gakiere B, Job D, Douce R (1998) The specific features of methionine biosynthesis and metabolism in plants. Proc Natl Acad Sci USA 95:7805–7812
Rea G, de Pinto MC, Tavazza R, Biondi S, Gobbi V, Ferrante P, De Gara L, Federico R, Angelini R, Tavladoraki P (2004) Ectopic expression of maize polyamine oxidase and pea copper amine oxidase in the cell wall of tobacco plants. Plant Physiol 134:1414–1426
Reggiani R (2006) A role for ethylene in low-oxygen signaling in rice roots. Amino Acids 30:299–301
Rozen S, Skaletsky H (2000) Primer3 on the WWW for general users and for biologist programmers. Method Mol Biol 132:365–386
Saab IN, Sachs MM (1996) A flooding-induced xyloglucan endo-transglycosylase homolog in maize is responsive to ethylene and associated with aerenchyma. Plant Physiol 112:385–391
Shi F, Yamamoto R, Shimamura S, Hiraga S, Nakayama N, Nakamura T, Yukawa K, Hachinohe M, Matsumoto H, Komatsu S (2008) Cytosolic ascorbate peroxidase 2 (cAPX 2) is involved in the soybean response to flooding. Phytochemistry 69:1295–1303
Shibaoka H, Nagai R (1994) The plant cytoskeleton. Curr Opin Cell Biol 6:10–15
Simone OD, Haase K, Muller E, Junk WJ, Hartmann K, Schreiber L, Schmidt W (2003) Apoplasmic barriers and oxygen transport properties of hypodermal cell walls in roots from four Amazonian tree species. Plant Physiol 132:206–217
Soares NC, Francisco R, Vielba JM, Ricardo CP, Jackson PA (2009) Associating wound-related changes in the apoplast proteome of Medicago with early steps in the ROS signal-transduction pathway. J Proteome Res 8:2298–2309
Somerville C, Bauer S, Brininstool G, Facette M, Hamann T, Milne J, Osborne E, Paredez A, Persson S, Raab T, Vorwerk S, Youngs H (2004) Toward a systems approach to understanding plant cell walls. Science 306:2206–2211
Speer EO (1987) A method of retaining phloroglucinol proof lignin. Stain Technol 62:279–280
Sperry JS (2003) Evolution of water transport and xylem structure. Int J Plant Sci 164:115–127
Staswick PE (1988) Soybean vegetative storage protein structure and gene expression. Plant Physiol 87:250–254
Voesenek LA, Colmer TD, Pierik R, Millenaar FF, Peeters AJ (2006) How plants cope with complete submergence. New Phytol 170:213–226
Wang K, Jiang Y (2007) Antioxidant responses of creeping bent-grass roots to water-logging. Crop Sci 47:232–238
Wang S-B, Chen F, Sommerfeld M, Hu Q (2005) Isolation and proteomic analysis of cell wall-deficient Haematococcus pluvialis mutants. Proteomics 5:4839–4851
Watson BS, Lei Z, Dixon RA, Sumner LW (2004) Proteomics of Medicago sativa cell walls. Phytochemistry 65:1709–1720
Yoda H, Yamaguchi Y, Sano H (2003) Induction of hypersensitive cell death by hydrogen peroxide produced through polyamine degradation in tobacco plants. Plant Physiol 132:1973–2981
Zhong Z, Karibe H, Komatsu S, Ichimura H, Nagamura Y, Sasaki T, Hirano H (1997) Screening of rice genes from a cDNA catalog based on the sequence data-file of proteins separated by two-dimensional electrophoresis. Breed Sci 47:245–251
Zhu J, Chen S, Alvarez S, Asirvatham VS, Schachtman DP, Wu Y, Sharp R (2006) Cell wall proteome in the maize primary root elongation zone. I. Extraction and identification of water-soluble and lightly ionically bound proteins. Plant Physiol 140:311–325
Acknowledgments
We thank Mr. M. Z. Nouri and Dr. F.-J. Kong for their experimental help. We also thank Dr. S. Shimamura and T. Nakamura for their valuable discussion. This work was supported by a Grant-in-Aid for Scientific Research (B) (19380015) of the Japan Society for the Promotion of Science.
Author information
Authors and Affiliations
Corresponding author
Additional information
S. Komatsu and Y. Kobayashi contributed equally to the present study.
Electronic supplementary material
Below is the link to the electronic supplementary material.
Rights and permissions
About this article
Cite this article
Komatsu, S., Kobayashi, Y., Nishizawa, K. et al. Comparative proteomics analysis of differentially expressed proteins in soybean cell wall during flooding stress. Amino Acids 39, 1435–1449 (2010). https://doi.org/10.1007/s00726-010-0608-1
Received:
Accepted:
Published:
Issue Date:
DOI: https://doi.org/10.1007/s00726-010-0608-1