Abstract
The key role of APP in the pathogenesis of Alzheimer disease is well established. However, postnatal lethality of double knockout mice has so far precluded the analysis of the physiological functions of APP and the APLPs in the brain. Previously, APP family proteins have been implicated in synaptic adhesion, and analysis of the neuromuscular junction of constitutive APP/APLP2 mutant mice showed deficits in synaptic morphology and neuromuscular transmission. Here, we generated animals with a conditional APP/APLP2 double knockout (cDKO) in excitatory forebrain neurons using NexCre mice. Electrophysiological recordings of adult NexCre cDKOs indicated a strong synaptic phenotype with pronounced deficits in the induction and maintenance of hippocampal LTP and impairments in paired pulse facilitation, indicating a possible presynaptic deficit. These deficits were also reflected in impairments in nesting behavior and hippocampus-dependent learning and memory tasks, including deficits in Morris water maze and radial maze performance. Moreover, while no gross alterations of brain morphology were detectable in NexCre cDKO mice, quantitative analysis of adult hippocampal CA1 neurons revealed prominent reductions in total neurite length, dendritic branching, reduced spine density and reduced spine head volume. Strikingly, the impairment of LTP could be selectively rescued by acute application of exogenous recombinant APPsα, but not APPsβ, indicating a crucial role for APPsα to support synaptic plasticity of mature hippocampal synapses on a rapid time scale. Collectively, our analysis reveals an essential role of APP family proteins in excitatory principal neurons for mediating normal dendritic architecture, spine density and morphology, synaptic plasticity and cognition.
Similar content being viewed by others
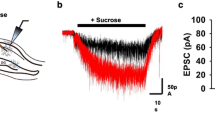
Avoid common mistakes on your manuscript.
Introduction
The loss of cognitive ability in Alzheimer disease (AD) is caused by impairments in synaptic function that occur already at early stages of AD. Loss of synapses is highly correlated with disease severity and cognitive decline [50, 57]. In contrast, deposition of the β-amyloid peptide Aβ in extracellular plaques, a major hallmark of AD, develops only at later stages of AD. Thus, deciphering the mechanism underlying these early synaptic changes is of crucial importance. The prevailing view of AD pathogenesis is that aberrant proteolytic processing of the amyloid precursor protein APP leads to the accumulation of Aβ oligomers and fibrils that trigger a detrimental synapto- and neurotoxic cascade. To date, however, AD pathogenesis is still incompletely understood, despite enormous efforts focusing on Aβ generation and Aβ-induced neurotoxicity, including numerous studies on transgenic APP overexpressing mice. One important aspect that is still not resolved is the natural in vivo function of APP for brain physiology and whether the loss of APP-mediated functions contributes to AD pathogenesis, either directly or by a shift in proteolytic processing. Thus, it is essential to elucidate the in vivo function(s) of APP and its various proteolytic fragments, including their roles not only for synapse formation and maintenance, but also for the modulation of synaptic plasticity in the adult brain, required for newly formed declarative memory.
Aβ is generated by sequential cleavage of APP by β- and γ-secretase. In the competing and physiologically predominant non-amyloidogenic pathway α-secretase cleavage of APP occurs at a membrane-proximal site within the Aβ region [33, 46]. Thus, α-secretase processing precludes the formation of Aβ peptides and also liberates the soluble, neuroprotective ectodomain APPsα, which is secreted into the extracellular space in a process coupled to neuronal and synaptic activity [24, 25]. Consistent with a protective function of APPsα, a mutation associated with late-onset AD has been identified within the gene encoding the major α-secretase huADAM10 (a disintegrin and metalloprotease, ADAM) that attenuated ADAM10 activity [55]. Interestingly, APPsα can also affect APP processing directly, by reducing β-secretase (BACE-1) activity in AD model mice [43]. Moreover, there is a large body of evidence that implicates APPsα in neuroprotection against excitotoxicity, growth factor deprivation, proteasomal stress or traumatic brain injury in vivo [7, 29, 41].
APP belongs to a gene family including the amyloid precursor-like proteins, APLP1 and APLP2, in mammals [4]. Although APLP1 and APLP2 lack the Aβ region, they are similarly processed by α-, β-, and γ-secretases [60]. APP and APLP2 show widespread expression throughout the brain, which is particularly high in pyramidal cells of the cortex and hippocampus [34]. APP and APLP2 have been localized to synaptic sites, including the presynaptic active zone [31, 64]. In addition to the neuroprotective functions of APPsα, APP has been implicated in several biological processes including cell adhesion, gene transcription, neuronal differentiation, migration, neurite outgrowth, and synaptogenesis [4, 42]. Of note, for the majority of these proposed functions, direct in vivo evidence by genetic loss-of-function studies is still missing. These studies have been hampered by partially redundant functions within the gene family, with mice lacking individual family members being fully viable and showing only subtle phenotypes, whereas combined APP/APLP2 double knockout (DKO) and APLP1/APLP2-DKO mice die perinatally [21, 22, 35, 48]. Previous studies from us and others revealed that lethality of DKO mice is due to essential functions of APP family proteins at the neuromuscular junction, with APP/APLP mutants exhibiting abnormal neuromuscular morphology and impaired transmitter release including reductions in quantal content, readily releasable pool, and the ability to sustain vesicle release [6, 28, 61, 62]. Until now, the perinatal lethality of APP/APLP2-DKO mice precluded the analysis of APP/APLP2-mediated functions in the adult nervous system.
To circumvent lethality, we generated mice with a conditional APP/APLP2 double knockout (cDKO) in excitatory forebrain neurons using the NexCre driver line. NexCre cDKO mice showed severely impaired short-term and long-term synaptic plasticity associated with impairments in hippocampus-dependent tasks. In addition, hippocampal neurons of cDKO mice revealed pronounced reductions in neurite length, dendritic branching, spine density and spine head size, related to synaptic strength. Our mechanistic analysis revealed an acute function of secreted APPsα to modulate adult central nervous system (CNS) synapses and rescue on a rapid time scale defects in synaptic plasticity.
Materials and methods
Mice
Experiments on animals were performed in accordance with the guidelines and regulations set forth by the German Animal Welfare Act and the Regierungspräsidium Karlsruhe, Germany. Generation and genotyping of mouse lines were as described previously: APPflox [36]; APLP2-KO [59]; NexCre [20]. The three strains were crossbred and final matings were APPflox/floxAPLP2−/− × APPflox/floxAPLP2−/−NexCre+/T to obtain 50 % NexCre cDKOs and 50 % APLP2-KO littermate controls (see also mating scheme Fig. S1d).
LacZ staining, in situ hybridization, CNS immunohistochemistry, Western blot analysis, monoclonal antibody production, transfection of HEK293T cells, and brain stereology
See Electronic Supplementary Material (ESM).
Behavioral analysis
A total of 17 NexCre cDKOs (9 males, 8 females) and 15 littermate controls (7 males, 8 females) were tested, a sample size recommended in the literature [10].
Mice were analyzed in a blinded manner in the following order of tests: home cage activity (age 9–13 weeks), open field (11–15 weeks), grip test (11–15 weeks), water maze (12–16 weeks), nesting (13–17 weeks), and radial maze (14–18 weeks). All behavioral procedures were approved by the Veterinary Office of the Canton of Zurich (License 2012/29).
Grip strength, home cage activity, open field, water maze place navigation, radial maze, nesting test and statistics
See ESM.
Neuronal morphology, spine density and spine morphology
In brief, CA1 pyramidal cells were visualized by postfixation filling with Alexa 568 in 200 μm horizontal brain sections of 11- to 13-week-old mice. For iontophoretic filling of CA1 pyramidal neurons, one brain slice at a time was placed in a custom-made chamber filled with cold PBS and visualized on an Olympus BX51WI fixed stage upright microscope. Sharp quartz glass electrodes (Sutter instruments, Quartz electrodes with filament; O.D: 1.0 mm, I.D.: 0.7 mm; 10 cm length) were pulled using the Sutter P-2000 Laser Puller. The tip of the electrode was loaded with 5 mM Alexa 568 dye dissolved in distilled water and backfilled with 0.1 M LiCl2 dissolved in distilled water. Using a motorized 3D micromanipulator, the electrode was lowered into the hippocampal CA1 region under visual control using a Calcium Crimson filter cube (HQ580/20x, U-Q595LP (339038), HQ630/60m) while applying a negative voltage pulse (−1 V, 1 Hz) to the electrode via a silver wire in line with a 500 MΩ resistor. When piercing of a cell body was observed, the cell was filled by application of a negative 1 Hz current pulse (~5 nA) to the electrode. Filling was for 10 min or until no further filling was observed. Afterwards, slices were fixed for 2 days in 4 % PFA at 4 °C, washed three times in cold PBS and finally mounted in Mowiol. Upon imaging neurons were manually reconstructed using Neurolucida software (MicroBrightField, Williston, USA) blind to genotype. Morphometric Sholl analysis, spine density counts and spine morphology analysis were performed as described [63]. For details including statistics see ESM.
Extracellular recordings in acute hippocampal slices, patch clamp analysis
Extracellular recordings for basal synaptic properties, LTP and PPF analysis were performed in acute hippocampal slices from 16- to 23-week-old NexCre cDKO and APLP2-KO littermate control mice. For patch clamp electrophysiology, 19- to 22-week-old mice were used. Electrophysiological recordings were performed as previously described [53, 62] and detailed in ESM.
LTP recordings in the presence of recombinant APPsα and APPsβ peptide
The peptide concentrations (for purification of recAPPsα and recAPPsβ see ESM) were set to 10 nM for the recHis-APPsα and 50 nM for recHis-APPsβ in a total volume of 30 ml of carbogented ACSF, circulating in a closed-loop perfusion system. The boiled peptides (20 min at 95 °C) served as negative control. To circumvent sticking to the surface, silicone tubings (Pharmed® Ismaprene, Ismatec) were used. For electrophysiological recordings the perfusion rate in the recording chamber was kept at 1.5 ml/min. All slices were pre-incubated with the respective peptide for 60 min at room temperature and were transferred to the recording chamber for investigating the basal synaptic properties and studying the LTP.
Group means were compared using a two-tailed Student’s t test, level of significance was set at p ≤ 0.05. Data are presented as mean ± SEM.
Results
Generation of NexCre-driven APP/APLP2 conditional double knockout mice in excitatory forebrain neurons
To circumvent lethality of APP/APLP2 double KO mice and to elucidate the role of APP and APLP2 in the adult CNS we generated animals with a conditional CNS specific APP/APLP2 double knockout (cDKO). To this end, we crossed APPflox/flox/APLP2-KO mice to NexCre mice [20] expressing Cre prenatally (from about E11.5 onwards) in postmitotic neuronal progenitor cells as confirmed by crosses to Cre reporter mice (see Fig. S1a; [20]), leading to APP gene deletion selectively in excitatory forebrain neurons of the cortex and hippocampus. NexCre cDKO mice lacking APP in excitatory forebrain neurons on a global constitutive APLP2-KO background had normal brain and bodyweight, as compared to APLP2-KO littermate controls (Fig. S1b, c). NexCre cDKO mice lacking APP only in brain while retaining APP expression at the neuromuscular junction proved fertile and fully viable (see also Fig. S1d) up to at least 1 year of age. This further supports the view that the perinatal lethality of constitutive global APP/APLP2-DKO mice is primarily due to deficits in neuromuscular transmission [62]. APP gene deletion was assessed by in situ hybridization (Fig. S2a) and Western blot analysis, and confirmed efficient abrogation of APP expression to about 10 % of wild-type (WT) level in both cortex and hippocampus (Fig. S2b–d). To validate the APP gene knockout at cellular resolution we employed immunohistochemistry (IHC). As most commercial APP antibodies show high levels of unspecific staining or crossreact with the highly related APLPs in IHC on tissue sections we used a newly developed mouse monoclonal antibody (JRD32) that is directed against the extracellular APP-E1 domain and detects APP in Western blot and IHC with high specificity, as demonstrated by using APP-KO brain as a negative control (see Fig. S3). JRD32 staining of littermate control sections showed a particularly high APP immunoreactivity in pyramidal neurons within the hippocampal CA3–CA1 region, whereas staining of granule cells of the dentate gyrus was much weaker (Fig. S2e), consistent with specific but low-intensity signals obtained by in situ hybridization (Fig. S2a). JRD32 staining of NexCre cDKO brain sections further confirmed APP deletion in excitatory forebrain neurons of the cortex and hippocampus including CA3 and CA1 pyramidal cells (Fig. S2e), whereas APP expression remained detectable in interneurons (Fig. S2f).
NexCre cDKO mice show pronounced deficits in hippocampus-dependent learning and memory tasks, but normal motor behavior
Previously, we had demonstrated that APP-KO mice show muscular weakness [48]. Moreover, our analysis of APPsα-DM mice, a line of double mutant mice (DM) that lack transmembrane APP and expressed solely the secreted APPsα fragment on an APLP2-KO background, showed impaired neuromuscular transmission that was associated with pronounced deficits in neuromotor tasks [62]. In contrast, NexCre cDKO mice showed no muscular weakness as evidenced by normal grip strength (Fig. S4a). We also assessed basal locomotor activity and exploratory behavior. Monitoring of the diurnal activity profile in a familiar home cage revealed no significant differences (Fig. S4b). Likewise, we failed to detect any alteration of activity or center field avoidance in the open field, which assesses locomotor activity and exploratory behavior in a novel environment (Fig. S4c, d).
Having established that NexCre cDKO mice show normal muscle strength, motor behavior and exploration, we went on to assess their performance in hippocampus-dependent tasks. Spatial learning and memory of NexCre cDKO mice was first tested in the water maze place navigation task. Our previous analysis of APP-KO mice had indicated impaired Morris water maze performance only in aged (9- to 12-month-old) mice [48]. While swim speed was normal (Fig. 1a), we observed overall impaired performance during acquisition and reversal training already in young adult (12- to 16-week-old) NexCre cDKO mice (n = 15 NexCre cDKO and n = 12 littermate controls, balanced proportion of males and females), pointing toward an important role of APLP2 in addition to APP. While both groups of mice did show learning, escape latencies (Fig. 1b) of NexCre cDKO mice and in males also swim path lengths (Fig. 1c) were significantly increased, especially during the last trials of acquisition learning and at the beginning of reversal learning. Post hoc analyses (see ANOVA table, Fig. S5e) revealed that the absence of a significant difference in swim path length between NexCre cDKO females and control females (Fig. 1d) was due to poor performance of female controls. Female controls had significantly longer swim paths than male controls, with no sex difference in the NexCre cDKO group (see ANOVA table, Fig. S5e). Detailed analysis of swim paths revealed reduced goal orientation of NexCre cDKO mice, as evidenced by increased cumulative search error (Fig. S5c) and in male NexCre cDKOs also increased Whishaw’s error, especially at the end of acquisition (Fig. S5a). As observed for swim path length, post hoc analyses (see ANOVA table, Fig. S5e) revealed that female controls had earned poorer scores than male controls. Thigmotaxis decreased strongly in both groups during the early training phase (Fig. S5d) but was overall slightly increased in NexCre cDKO mice. During the probe trial (first trial after platform relocation), NexCre cDKO mice spent significantly less time in the target zone and as a group failed to show a significant preference for the trained target (Fig. 1e), indicating a deficit in spatial reference memory. Impaired probe trial performance was clearly independent of sex (see Fig. S5e, genotype × sex × place: F(1,23) = 0.2 p = 0.639, ns).
NexCre cDKOs show deficits in hippocampus-dependent memory tasks. a–e Place navigation in the Morris water maze. a Swim speed during acquisition and reversal learning is unaffected by the mutation (each point represents a bin of 3 trials, with 6 trials per day). b During acquisition learning and reversal, NexCre cDKOs show an overall reduced performance compared to littermate controls. c, d Measurements of swim path length confirms the training performance deficit, although due to poor scores in female controls this effect is contributed by males (see ANOVA table, Fig. S5e). e In the probe trial mutants spend significantly less time in the target zone and fail to prefer it significantly over control zones in adjacent quadrants. f–h Spatial working memory analysis in the radial arm maze. f NexCre cDKOs are less efficient in collecting the baits leading to a smaller number of correct among the first 8 choices. g, h Mutants make more reentry errors during training. g Reentry errors per collected bait plotted as function of trial (averaged across bait numbers), h Reentry errors per collected bait plotted as function of bait number (averaged across trials). Error rate increases more strongly with the number of baits already collected during a trial than in controls, indicative of greater sensitivity to memory load. i NexCre cDKOs show a significantly reduced nest score. Age of animals: water maze 12–16 weeks; nesting 13–17 weeks; radial maze 14–18 weeks. a–e n = 15 NexCre cDKOs, n = 12 littermate controls. f–i n = 17 NexCre cDKOs, n = 15 littermate controls. *p < 0.05, **p < 0.01. Error bars SEM. Dashed lines represent chance level. Data were analyzed using mixed ANOVA models with genotype (NexCre cDKO vs. control) as between subject factor and within subject factors to explore the dependence of genotype effects on place, time, or response type. Separate evaluation of male and female data is only presented where the ANOVA model indicated a significant sex × genotype interaction. See Fig. S5e for full ANOVA data
To assess spatial working memory, mice underwent testing in an 8-arm fully baited radial maze. Whereas littermate controls learned the task very well, achieving on average 7–8 correct choices at the end of training, NexCre cDKO mice were clearly impaired (Fig. 1f). When collecting 8 baits from the 8 freely accessible arms of the radial maze, NexCre cDKO mice entered arms from which they had already collected the bait more often than controls, resulting in significantly increased reentry errors (Fig. 1g). In both groups, the number of reentry errors increased with the number of baits already collected, reflecting the increasing challenge of working memory, but in NexCre cDKO mice this increase was significantly steeper than in littermate control mice (Fig. 1h). Finally, animals were tested in nesting behavior, a hippocampus-dependent species-typical behavior, where NexCre cDKO mice proved to be strongly impaired as compared to littermate controls (Fig. 1i). Importantly, all impairments in radial maze and nesting were independent of sex (see Fig. S5e). Taken together NexCre cDKO mice showed impairments in three independent hippocampus-dependent tasks indicating that APP/APLP2 are required for spatial reference and working memory, as well as normal nesting behavior.
NexCre cDKO leads to reduced neurite length and impaired dendritic branching
Histopathological analysis of the brain of adult NexCre cDKO mice using Giemsa-stained glycolmethacrylate sections revealed neither gross abnormalities, nor abnormal necrotic or apoptotic cell death (Fig. 2a). In contrast to previously generated APP/APLP1/APLP2 triple knockout mice that showed neuronal ectopias in the marginal zone of the cerebral cortex [22], we observed no overt disruptions of the cortical laminar cytoarchitecture or ectopias in NexCre cDKO mice. We used serial frontal sectioning to estimate the volume of the hippocampus and neocortex (Fig. 2b, c). This way, we observed a small reduction in hippocampal volume (9.3 ± 0.4 vs. 8.3 ± 0.3 mm3, Student’s t test, p = 0.033; Fig. 2b) that is most likely due to reductions in dendritic complexity of NexCre cDKO neurites (see Fig. 3 and results below).
NexCre cDKOs show no alterations in hippocampal and cortical morphology but slightly reduced hippocampal volume. a Giemsa-stained, glycolmethacrylate-embedded brain sections display no abnormal necrotic or overt apoptotic cell death, and no overt disruption of nuclear or cortical laminar cytoarchitecture in cortex and hippocampus. b Stereological evaluation showed reductions of hippocampal volume by about 10 % (Student’s t test, p = 0.033). c Cortical volume was unaffected (Student’s t test, p = 0.18, ns). n = 3 brains/genotype (age of animals: 16–19 weeks). Error bars SD. Scale bars overview 500 µm; CA1, Cortex 50 µm
Neurons of NexCre cDKO mice exhibit impaired dendritic complexity and reduced total neurite length. a Representative 3D-reconstructions of CA1 pyramidal neurons from littermate control (left) and NexCre cDKO mice (right). b Schematic representation of parameters assessed. c Sholl analysis reveals a significant overall genotype effect on apical dendritic morphology that was most prominent in proximal regions (repeated measures ANOVA: genotype F(1,20) = 9.818, p = 0.0052, with post hoc Bonferroni multiple comparison test, *p < 0.05). d NexCre cDKO neurons display a significantly reduced total apical dendritic length and e reduced dendritic branching. f Sholl analysis reveals a significant overall genotype effect on basal dendritic morphology (repeated measures ANOVA: genotype F(1,19) = 4.710, p = 0.043, with post hoc Bonferroni multiple comparison test, *p < 0.05 **p < 0.01). g NexCre cDKO neurons display a significantly reduced total basal dendritic length and h dendritic branching. n = number of neurons analyzed (from 5 animals/genotype, age: 11–13 weeks). Error bars SEM
Conditional CaMKIICre-driven knockout of the major α-secretase ADAM10 (cADAM10-KO) has recently been reported. As the cADAM10-KO led to a drastic reduction in APPsα levels that was associated with pronounced cortical and hippocampal gliosis [45], this prompted us to assess potential gliosis in cDKO mice. Using GFAP staining we did not detect, however, any significant astrogliosis in NexCre cDKO mice as compared to littermate controls, neither in young (12 weeks) nor aged (1-year-old) mice (Fig. S6), indicating that selective lack of APP/APLP2 does not induce astrogliosis.
Previously, we and others have shown that APP family proteins are involved in trans-cellular adhesion [51], and that lack of APP/APLP2 in mice results in impaired synaptogenesis at the neuromuscular junction [61, 62]. Prompted by the absence of gross anomalies in brain architecture we asked whether defects in hippocampal function might be related to compromised morphology of hippocampal neurons. We focused on CA1 neurons because this region is highly vulnerable in AD, is one of the best studied brain regions with regard to synaptic plasticity, and our previous analyses indicated LTP defects at CA3/CA1 synapses in APP mutant mice [48, 62]. To visualize neuronal architecture, we performed iontophoretic postfixation filling of mature hippocampal CA1 pyramidal cells in brain slices from adult (11- to 13-week-old) mice with a fluorescent dye. CA1 neurons of NexCre cDKO mice displayed distinct alterations of neuronal architecture, including considerably reduced dendritic branching, that were already apparent when visually inspecting reconstructed images (Fig. 3a). In view of their different morphology and connectivity, we analyzed apical and basal dendrites of CA1 neurons separately. Performing morphometric Sholl analysis, we plotted the dendritic length measured within circles centered on the soma against the distance from the cell body (see scheme in Fig. 3b). For the measurement of dendritic complexity the complete apical dendritic arbor was analyzed. This detailed analysis revealed significant reductions in dendritic length and complexity in proximal as well as distal regions of apical dendrites (Fig. 3c). Overall this resulted in a highly significant reduction (on average by about 21 %) in total dendritic length of apical dendrites (Fig. 3d) of NexCre cDKO CA1 neurons, while the maximal extension of the longest apical neurite was unaffected (data not shown). We also observed a prominent reduction in total dendritic branching (Fig. 3e) in cDKO neurons in comparison to littermate controls. Similar to our findings in apical dendrites, also the basal tree of NexCre cDKO CA1 neurons showed an impaired morphology (Fig. 3f), a significant reduction (on average by about 20 %) in basal dendritic length (Fig. 3g), and reduced dendritic branching (Fig. 3h).
NexCre cDKO impairs spine density and spine morphology in hippocampal CA1 neurons. a, b Comparison of spine densities of midapical and basal dendritic segments. NexCre cDKOs show an average reduction in spine density of a 16 % (2.05 ± 0.06 vs. 1.72 ± 0.10 spines/µm) in midapical dendrites and of b 10 % (2.19 ± 0.04 vs. 1.97 ± 0.08 spines/µm) in basal dendrites. c Representative images of midapical and basal dendritic segments of control and mutant animals and criteria used for spine type analysis. D = diameter, L = length. Images are maximum projections of deconvolved z-stacks. d NexCre cDKOs show a significant reduction of mushroom spines (82.7 ± 1.1 vs. 76.7 ± 1.9 %) and a concomitant increase in stubby spines (13.6 ± 0.9 vs. 20.1 ± 1.8 %). The proportion of thin spines is unaltered. e Spine head diameters of all evaluated spines of the two genotypes plotted as cumulative frequency. The distribution is significantly shifted toward smaller spine heads in NexCre cDKOs (Kolmogorov–Smirnov (K–S) test, p < 0.0001). f Histogram of spine head diameters in 0.05 µm bins. X-axis shows bin center. n = number of neurons (from 5 animals/genotype, age: 11–13 weeks). Data represent mean ± SEM. Scale bar (c): 5 µm
NexCre cDKO dendrites show reduced spine density and fewer mushroom-type spines
Next we assessed spine density as a correlate of excitatory synapses. Numerous studies have demonstrated that overexpression of APP, e.g., in transgenic AD model mice leads to reduced spine density in the cortex and hippocampus, presumably due to detrimental effects of Aβ accumulation and oligomerization (reviewed in [27, 52]). Previous in vitro studies using dissociated APP-deficient neurons obtained conflicting results with an increase in functional synapses found in low-density autaptic cultures of APP-KO neurons [44] contrasting with reports of reduced spine counts in high-density APP-KO cultures or upon APP knockdown [32, 58]. In vitro systems, however, are prone to high experimental variability and may fall short of mimicking physiological concentrations of soluble factors and neuronal connectivity. To determine whether lack of APP/APLP2 affects spine counts in the adult brain in vivo, we performed a detailed analysis of spine density in three-dimensional high-magnification z-stacks of midapical portions of apical dendrites and in basal dendrites. Importantly, we detected in both apical and basal dendrites of NexCre cDKO CA1 neurons a prominent reduction in spine density (Fig. 4a, b). Next, we asked whether deficits in spine density are associated with differences in spine size and morphology, which in turn might impact electrophysiological properties of synapses including synaptic strength that has been correlated with spine head size. Three major spine types can be distinguished by morphological criteria: stubby, thin and mushroom spines. This classification is based on measurements of spine length and additionally the ratio between the spine head and spine neck diameter providing objective criteria (see Fig. 4c). Spine type analysis revealed that NexCre cDKO CA1 neurons show significantly fewer mushroom-type spines (82.7 ± 1.1 vs. 76.7 ± 1.9 %; Student’s t test, p = 0.010, Fig. 4d) and a corresponding increase in stubby spines (13.6 ± 0.9 vs. 20.1 ± 1.8 %; Student’s t test, p = 0.0032, Fig. 4d). Detailed analysis indicated a significant shift toward smaller spine head diameters in NexCre cDKOs (Fig. 4e, f), while the average spine length was not significantly different (0.90 ± 0.33 vs. 0.87 ± 0.32 µm, Student’s t test, p = 0.23, ns, data not shown). Taken together our findings indicate that APP and APLP2 are required for the maintenance of dendritic spine numbers and morphology, suggesting a reduction of active synapses in NexCre cDKO mice.
NexCre cDKO mice exhibit impaired synaptic function including deficits in paired pulse facilitation and long-term potentiation
Having established that NexCre cDKO mice exhibit pronounced deficits in hippocampus-dependent learning tasks and changes in the morphology of CA1 neurons, we went to the functional network level of neurons and analyzed whether these deficits are also represented in a defect of activity-dependent synaptic plasticity in NexCre cDKO mice. After 20 min of baseline activity recording we induced long-term potentiation (LTP) by application of theta burst stimulation (TBS) in acute hippocampal slices from adult NexCre cDKO mice or APLP2-KO littermate controls (16–23 weeks old). Importantly, our previous analysis [62, 67] did not show any significant difference in potentiation of APLP2-KOs compared to wild-type mice. Enlargement of fEPSP was recorded for either 60 min following TBS in order to cover the phase of protein synthesis-independent LTP (E-LTP, Fig. 5a) or for 180 min after TBS for analyzing protein synthesis dependent events (Late-LTP, Fig. S7a). In both cases, a pronounced defect of post-tetanic potentiation (PTP) as well as LTP can be observed. Nevertheless, NexCre cDKOs still reveal a stable, albeit reduced LTP and thus an increased efficiency of synaptic transmission after TBS stimulation. The average potentiation in littermate control slices was 161 ± 6.0 % (n = 31), whereas NexCre cDKOs revealed a significantly reduced potentiation of 127 ± 2.3 % (n = 26) 55–60 min after TBS (Fig. 5a (right), p < 0.0001, Student’s t test) in LTP recordings. Reduced potentiation was observed, even though less pronounced, also in long-term recordings (Fig. S7a). In order to investigate whether the observed LTP defect is also reflected in disturbed basal synaptic properties we examined pre- and postsynaptic functionality. Comparing the fEPSP size at defined stimulus intensities revealed no significant difference between both genotypes (Fig. 5b). Furthermore, we also failed to detect any defect in input–output strength at given fiber volley amplitudes (Fig. 5c). To assess whether presynaptic components are altered and thus contributing to the observed LTP deficit we investigated short-term plasticity using the paired pulse facilitation (PPF) paradigm. Compared to littermate controls NexCre cDKO mice revealed a highly significant impairment at shorter interstimulus intervals (ISI) of 10 and 20 ms (Fig. 5d, Student’s t test, p ≤ 0.01). As APP has been shown to interact with NMDARs [8, 9], we also performed patch clamp recordings of CA1 pyramidal cells in acute slices and recorded spontaneous synaptic miniature mEPSCs. These experiments revealed no significant differences between the cDKO and control mice (Fig. 5e–i). Despite the reduction in spine densities in basal and apical dendrites of cDKO mice we found no significant differences of mEPSC frequencies (controls 0.4 ± 0.06 Hz vs. cDKO 0.5 ± 0.07 Hz; Fig. 5e). Amplitudes of mixed (AMPA + NMDA) mEPSCs were −42.99 ± 3.36 pA for controls and −41.32 ± 1.64 pA for NexCre cDKOs (Fig. 5f, h). Likewise, isolated AMPA mEPSCs recorded in the presence of 30 µM APV had similar amplitudes (controls: −35.84 ± 2.73 pA vs. cDKO: −33.69 ± 1.24 pA; Fig. 5g, h). The calculated NMDA component of mEPSCs was also unaltered with NMDA components of 0.16 ± 0.04 in control animals and 0.18 ± 0.03 in the NexCre cDKOs (Fig. 5i). We also determined kinetic parameters that might indicate possible differences in subunit composition of postsynaptic receptors. Again, no significant differences were found for mEPSC rise or decay times (data not shown). To complement our electrophysiological and morphological analysis we used Western blotting to assess the expression of the postsynaptic density marker PSD95 that is concentrated in spines. Consistent with our finding of reduced spine density and more spines with a smaller head diameter in hippocampal neurons of NexCre cDKO mice (Fig. 4e, f), a pronounced reduction of PSD95 expression was found in both cortical and hippocampal brain extracts (Fig. 5j).
NexCre cDKOs show deficits in LTP and PPF, while basal synaptic transmission is unchanged. a Littermate controls (white circles) show a robust and stable potentiation of 161 ± 6.0 % for the last 5 min of recording (t75–80). NexCre cDKO mice (red circles) show a highly significant defect in LTP induction and maintenance compared to the control group (127 ± 2.3 %). t75–80: Student’s t test p < 0.0001, right. Insets show original traces of representative experiments 5 min before the TBS and 55 min after the TBS (vertical scale bar 1 mV, horizontal scale bar 5 ms). b, c Input–output strength was unaltered. d At shorter interstimulus intervals (ISIs), NexCre cDKO mice revealed a pronounced and highly significant presynaptic defect compared to the littermate controls tested by a PPF paradigm (Student’s t test: 10 ms p = 0.0039; 20 ms p = 0.0065). n = number of slices/number of animals (age of animals: 16–23 weeks). Error bars SEM. e–h Recordings of mEPSCs frequencies and amplitudes in CA1 pyramidal cells revealed no differences between littermate control and NexCre cDKO mice. e Quantification of AMPA mEPSC frequencies recorded in the presence of APV (left) and representative traces of mEPSC recordings (right). Vertical scale bar 20 pA, horizontal scale bar 1 s. f–h Quantification of median amplitudes of mEPSCs. f In the ACSF condition (Mg-free) events include NMDA and AMPA currents. g In the ACSF + APV condition, events are presumably pure AMPA currents due to blockage of NMDARs. h Averaged mEPSC traces of all analyzed events for both groups before (continuous line) and after (dashed line) wash-in of the NMDAR blocker APV (30 µM). Vertical scale bar 10 pA, horizontal scale bar 10 ms. i Relative contribution of APV-sensitive NMDA receptor-mediated current components, calculated as 1 (values recorded in the presence of APV/values recorded in ACSF). There were no statistical differences detected (Student’s t test; p > 0.05); test for normality passed; n = 6 cells from 5 animals/genotype (age of animals: 16–19 weeks) error bars SEM. j Western blot analysis and quantification of PSD95. Control and cDKO lysates were run on the same gel. Membranes were processed and incubated together with the antibodies indicated. The dashed line indicates where membranes were cut to allow incubation with PSD95 or anti-β-tubulin antibody, respectively. Note the pronounced reduction of PSD95 in NexCre cDKO hippocampus and cortex (Student’s t test, p = 0.0063 and p = 0.0035, respectively) n = 4 mice/genotype (age of animals: 16–19 weeks). Error bars SD
Impaired synaptic function of NexCre cDKO mice is rescued by acute application of recombinant APPsα but not APPsβ
Our findings of structural alterations in NexCre cDKO neurons raise the question of whether APP and APLP2 are primarily needed during the development (e.g., to specify basal neuronal morphology and circuit formation) or whether they are also required in the adult CNS to modulate dynamic properties of synapses such as synaptic plasticity (LTP), e.g., via secretion and signaling of APPsα in an activity-dependent manner. We therefore studied whether synaptic deficits of NexCre cDKO mice can be rescued by acute application of exogenous recombinant APPsα. Native His-tagged APPsα and His-tagged APPsβ were affinity-purified from the supernatants of stably transfected HEK cells. Acute slices of NexCre cDKO mice were pre-incubated for 60 min at room temperature either with native 10 nM recombinant His-APPsα (recHis-APPsα), 50 nM recHis-APPsβ, or the corresponding boiled peptides that served as negative control. Again, after 20 min of stable baseline recording TBS-induced LTP was recorded for 60 min. During the whole measurement, the respective peptides diluted in ACSF were circulating in a closed-loop system. Strikingly, and consistent with our hypothesis that APPsα is required for mediating synaptic plasticity, we found that the application of recHis-APPsα is sufficient to largely rescue the PTP and LTP defects observed in NexCre cDKO slices. The potentiation was 143 ± 5.1 % for t75–80 after TBS, whereas NexCre cDKO treated with the boiled recHis-APPsα peptide only reached potentiation levels of 130 ± 2.3 % (Fig. 6a, b), levels similar to the defect observed before in naive slices (compare Fig. 5a, for basal synaptic transmission see Fig. S7b–d). Having shown that APPsα is sufficient to restore synaptic plasticity defects observed in slices of NexCre cDKO mice we next asked whether the slightly shorter fragment APPsβ generated by β-secretase cleavage of APP might have similar properties. Interestingly, we found that LTP induction and maintenance are still compromised in the presence of recHis-APPsβ (even at 5 times higher molar concentration of 50 nM; t75–80: 126 ± 4.5 vs. 119 ± 3.9 %) irrespective of whether native or boiled recHis-APPsβ peptide was applied to NexCre cDKO slices (Fig. 6c, d; for basal synaptic transmission see Fig. S7e–g). These findings suggest a selective role of APPsα but not APPsβ as a mediator of activity-dependent synaptic plasticity in the mature nervous system.
Acute application of recAPPsα, but not recAPPsβ, rescues the LTP defect of NexCre cDKOs. a, b Treatment of NexCre cDKO slices with recAPPsα (green circles) partially restored the LTP defect (compare Fig. 5a, b) to a potentiation level of 143 ± 5.1 % (t75–80, p = 0.029, Student’s t test). NexCre cDKO mice treated with boiled recAPPsα (control, white circles) revealed a stable, but significantly smaller potentiation of 130 ± 2.3 % for t75–80 min, reflecting the LTP defect observed in Fig. 5a. c, d NexCre cDKO slices treated with recAPPsβ (orange circles) revealed a stable potentiation of 126 ± 4.5 % for t75–80 min which is indistinguishable from the LTP of NexCre cDKO slices treated with boiled recAPPsβ (white circles, t75–80: 119 ± 3.9 %, p = 0.28, ns, Student’s t test). Insets show original traces of representative experiments 5 min before the TBS and 55 min after the TBS (vertical scale bar 1 mV, horizontal scale bar 5 ms). n = number of slices/number of animals (age of animals: 16–23 weeks). Error bars SEM
Discussion
In this study, we provide direct in vivo evidence from genetic loss-of-function studies for an essential role of APP and the close homologue APLP2 for adult brain physiology. Our analysis of NexCre cDKO mice lacking APP selectively in excitatory forebrain neurons on a global APLP2-KO background revealed several important new findings: (1) lack of APP/APLP2 resulted in impaired synaptic plasticity (LTP and PPF) and was associated with defects in hippocampus-dependent learning and memory tasks; (2) lack of APP/APLP2 led to compromised neuronal morphology of adult CA1 neurons characterized by reduced neurite length, impaired dendritic branching and a reduction in spine density and spine head diameter; (3) mechanistic studies showed that LTP defects can be rescued by application of nanomolar amounts of recombinant APPsα, while APPsβ was ineffective. This novel key finding suggests an acute and specific function of endogenous APPsα in the adult brain to support the propensity of neuronal networks to adjust their synaptic strength on a rapid time scale according to changes in neuronal activity.
Previously, early postnatal lethality of APP/APLP2-DKO mice precluded the investigation of the presumably partially redundant functions of APP and APLP2 in the adult CNS. The analysis of neuronal morphology in the cortex and hippocampus of adult APP-KO mice indicated reduced dendritic branching and spine densities only in neurons from aged (12–14 month old) mice [49, 58]. No abnormalities were found in either young or aged APLP2-KO mice [40, 63]. Using a genetic approach, we now circumvented lethality and show pronounced defects in neuronal architecture of CA1 pyramidal cells already in young adult mice. Thus, our findings from NexCre cDKO mice are in clear contrast to the age-dependent deficits of APP-KO mice and to the largely unaltered phenotype of APLP2-KO mice, suggesting functional complementation by APLPs in young APP-KO mice. CA1 pyramidal cells of NexCre cDKO showed highly significant reductions of dendritic complexity and neurite length. Consistently, hippocampal volume of cDKO mice was slightly, but significantly decreased. Apical dendrites of NexCre cDKO neurons showed reduced complexity that was most prominent in the proximal and in the distal dendritic segments. Interestingly, our findings in mature adult CA1 neurons of NexCre cDKO mice fully confirm our recent analysis in organotypic hippocampal cultures (OHCs) from viable constitutive APP-KO, APLP2-KO and lethal constitutive APP/APLP2-DKO mice [63]. Similar to the in vivo analysis of adult mice conducted in this study, CA1 neurons from OHCs of APLP2-KOs showed normal morphology, whereas APP-KO neurons revealed a reduced dendritic complexity in distal dendrites. Importantly, OHCs of combined APP/APLP2-DKO mutants showed an additional branching defect in proximal apical dendrites, thus unmasking a function of APLP2 likely compensated by APP in single mutants [63] and closely resembling defects of fully mature, adult NexCre cDKO neurons. Taken together our results corroborate that both APP and APLP2 are required for normal neuronal morphology in vivo. A dual (potentially redundant) role of APP and APLP2 for neuronal complexity also suggests that conserved domains residing in the N- and C-termini likely mediate this effect, consistent with previous data from the neuromuscular junction in the peripheral nervous system [28, 61, 62] and in vitro studies [2, 13, 19, 23, 66]. In addition to deficits in dendritic complexity, we observed in mature neurons of NexCre cDKO mice a marked deficit in spine density and a significant shift toward smaller spine head size, which is corroborated by a reduced PSD95 level. Most interestingly, we also saw a reduction in the proportion of mushroom spines in 11- to 13-week-old NexCre cDKO mice, the spine type which is thought to represent mature synapses. Our data thus now establish a clear in vivo role of APP family members for the formation and/or maintenance of central synapses, in addition to their previously recognized role at the NMJ.
To investigate the underlying mechanisms of the observed defects, we studied hippocampal synaptic plasticity, which is considered to represent the cellular basis of newly formed declarative memories. Despite normal basal synaptic transmission, young adult NexCre cDKO mice showed pronounced deficits in the induction and maintenance phase of LTP. Again, these findings contrast with age-dependent LTP and learning defects of APP-single mutant mice [11, 48], and absence of alterations even in aged APLP2-KO mice [62]. These data suggest a combined role of APP and APLP2 for synaptic plasticity and hippocampus-dependent behaviors now unmasked by the combined knockout in NexCre cDKO mice. Interestingly, we also found a robust and highly significant impairment in paired pulse facilitation, especially at the important short interstimulus intervals, which may indicate a possible presynaptic deficit that could contribute to the reduced ability to induce LTP in NexCre cDKO mice. As APP and APLP2 are expressed both at pre- and postsynaptic sites [25, 31] APP/APLP-mediated function(s) in either or both compartments are conceivable. Consistent with a presynaptic role of APP, we previously found a reduction in quantal content and readily releasable pool at neuromuscular synapses of APP/APLP2 mutant mice, and biochemical interaction studies on HEK293 cells indicated that the cytoplasmic domain of APP may form a trimeric complex with Mint/Munc18 proteins involved in transmitter release [62]. In addition, an upregulation of presynaptic L-type Ca2+ channels (Cav1.2) and altered short-term plasticity were found in GABAergic striatal APP-KO neurons [65]. Previous immunoprecipitation studies indicated that APP can bind to GluN1/GluN2 NMDARs [8, 9]. To investigate if LTP deficits of NexCre cDKO mice arise due to impaired basal NMDAR levels we performed patch clamp recordings. Our analysis of spontaneous mEPSCs revealed, however, no significant differences in current amplitudes, kinetics or NMDA component of mixed EPSCs. Thus, we have no indication of any major alteration in postsynaptic glutamate receptor expression or composition, respectively. This also excludes that impaired PPF responses at very short interstimulus intervals are due to a reduced number of postsynaptic receptors. In apparent contrast to the reduction in synapse number, analysis of mEPSC frequency failed to reveal significant differences between control and cDKO mice. However, mEPSC frequency is a highly variable parameter and might not be sensitive enough to reflect the 10–16 % reduction in spine density in basal and apical dendrites, respectively. While these findings indicate normal excitatory synaptic function, they do not rule out, however, a pre- or postsynaptic function of APP or one of its fragments, e.g., APPsα, during phases of activity-dependent synaptic plasticity. Indeed, exogenously applied APPsα has been shown to potentiate tetanically evoked NMDAR currents in rat brain slices in vitro, whereas AMPAR currents were not affected [56]. Taken together, a dual role of APP and APLP2 at both pre- and postsynaptic sites appears likely (see also below).
Defects of activity-dependent synaptic plasticity in NexCre cDKO mice were not only reflected in lower LTP levels, lower spine densities and a reduced number of mushroom spines, but are also reflected in significant impairments of hippocampal function in processes of learning and memory in vivo. Using the Morris water maze paradigm we found clear deficits in place navigation learning in NexCre CDKO mice. For two training parameters, swim path and Whishaw’s error, a genotype-dependent effect was only found in male mice. This was in part explained by the fact that female controls had earned poorer scores than male controls. The rapid decrease of thigmotaxis within the first day of water maze training in both groups indicates that NexCre cDKO were able to overcome their initial orientation toward the sidewalls and rapidly learned that there was no way of escape at the pool border. It further suggests that the modest overall increase of their thigmotaxis scores is secondary to the inefficient navigation as revealed by cumulative search error and Whishaw’s error. Of note, careful and thorough examination of motor and exploratory behaviors excluded potential confounding effects. Results of the open field test show that the reaction of NexCre cDKO mice to a novel aversive environment is normal and thus excludes that deficits of NexCre cDKO mice in learning tests are due to emotional factors. Importantly, NexCre cDKO mice also showed, independent of sex, impaired long-term memory, with severely impaired probe trial performance. Moreover, radial maze analysis of NexCre cDKO mice showed highly significant impairments in spatial working memory, that were unaffected by sex. These deficits in hippocampus-dependent learning and memory were further corroborated by pronounced deficits in nest building, a species-specific test highly sensitive to hippocampal lesions [12]. In summary, we provide converging evidence for a cognitive deficit in NexCre cDKO mice that is based on three tests: water maze place navigation, radial maze and the nesting test.
In principle, deficits of NexCre cDKO mice can be due to the lack of either full length APP, APLP2 or any of their proteolytic fragments. Indeed, previous work from us and others implicated APPsα in synaptic plasticity [11, 16, 26, 48, 49, 56]. Our surprising findings that LTP defects of NexCre cDKO mice can be rescued by acute application of low amounts of recAPPsα are intriguing in several ways. First, they indicate that defects in neuronal morphology and possible impairments in circuit formation arising during development are unlikely to be the primary cause of LTP defects in adult mice. In fact, we consider it more likely that the reduced expression of PSD95 and the reduction in mushroom-type mature spines result from a reduced ability to undergo activity-dependent synaptic plasticity that is known to increase the size of postsynaptic densities (see e.g., [54]). Even more importantly, these novel findings suggest an endogenous role of APPsα for acutely modulating on a rapid time scale the properties of synapses in the adult brain. High-frequency stimulation, neuronal depolarization and synaptic NMDA receptor activation have been shown to stimulate α-secretase processing of APP [16, 19, 25]. Indeed, the major α-secretase ADAM10 has been localized at synaptic sites [37] and a recent conditional ADAM10-KO led to impairments in spine morphology, LTP and behavior [45]. This suggests that APPsα release needs to occur in a highly regulated fashion by synapses undergoing LTP [16]. In this regard, we previously showed LTP deficits in APPsα knockin mice that constitutively express only APPsα (in the absence of transmembrane APP) on an APLP2-KO background (APPsα-DM, [62]). We hypothesize that in these mutants, unregulated constitutive APPsα expression may have lead to insufficient local amounts or abnormal timing of APPsα release. Moreover, we should also consider that APLP2 may contribute to plasticity processes. As APP is a very complex molecule, having established that APPsα can rescue the LTP deficits of NexCre cDKO mice does by no means rule out further synaptic roles of other APP domains including the conserved APP C-terminus known to bind a number of adaptor proteins (reviewed in [4]) including Mint, Fe65, Dab1, Grb and Shc that may link APP to various intracellular signaling pathways (see e.g., [38, 62]). Future studies are needed to investigate whether defects in neuronal morphology and behavior of NexCre cDKO mice may also be rescued by specific APP (or APLP2) domains or fragments.
Recent studies implicated Aβ as an endogenous modulator of presynaptic release [1, 47] functioning via binding to presynaptic APP homodimers [17]. APPsα, on the other hand, was able to rescue synaptic plasticity deficits of NexCre cDKO mice, indicating that the still elusive receptor of APPsα-mediating plasticity must be distinct from either APP or APLP2. A further very intriguing finding was the differential effect of purified recAPPsα that was able to rescue LTP deficits while recAPPsβ was inefficient, as this may indicate that the critical domain is encoded by the unique last 17 aa of APPsα or that the presence of this sequence may alter the conformation of APPsα in a critical way. The finding that APPsβ is unable to substitute for APPsα in synaptic plasticity has important implications for AD pathogenesis, as there is a shift toward β-secretase processing during AD and insufficient amounts of APPsα might thus contribute to defects in synaptic plasticity and cognitive function in AD patients. Differential effects of APPsα and APPsβ have also been reported for other paradigms, most notably in assays of neuroprotection in which APPsβ has been reported by several studies as being far less effective (see e.g., [7, 18, 41] and review by [29]). Our data are in line with and further extend previous results from Taylor et al. [56] who reported impaired in vivo LTP in the dentate gyrus of rats upon intra-hippocampal infusion of antibodies directed against the APPsα C-terminus, whereas antibodies recognizing other epitopes were ineffective. Clear interpretation of these data was, however, hampered by the fact that these APPsα targeted antibodies also recognized Aβ and transmembrane APP [56]. In line with our data, the pharmacological inhibition of α-secretase lead to LTP defects and could be partially rescued selectively by APPsα, but not APPsβ [56].
Collectively, our data provide compelling evidence for an essential role of both APP and APLP2 for neuronal and synaptic morphology as well as hippocampal function. They also suggest an acute and specific function of endogenous APPsα to facilitate synaptic plasticity. AD is characterized by a shift toward amyloidogenic APP processing, and reduced levels of APPsα or ADAM10 were reported in patients with amyloid deposits and AD ([14, 30], reviewed in [15]). Further, poor memory performance was correlated with reduced APPsα in the CSF of aged rats [3]. Thus, together with the well-established neuroprotective role of APPsα and evidence that infusion of APPsα is able to enhance memory performance in wild-type mice [5, 39] our data further support the concept of enhancing/restoring APPsα levels as a potential strategy to alleviate AD-related symptoms and synaptic impairments especially during early stages of disease.
References
Abramov E, Dolev I, Fogel H, Ciccotosto GD, Ruff E, Slutsky I (2009) Amyloid-beta as a positive endogenous regulator of release probability at hippocampal synapses. Nat Neurosci 12(12):1567–1576. doi:10.1038/nn.2433
Allinquant B, Hantraye P, Mailleux P, Moya K, Bouillot C, Prochiantz A (1995) Downregulation of amyloid precursor protein inhibits neurite outgrowth in vitro. J Cell Biol 128(5):919–927
Anderson JJ, Holtz G, Baskin PP, Wang R, Mazzarelli L, Wagner SL, Menzaghi F (1999) Reduced cerebrospinal fluid levels of alpha-secretase-cleaved amyloid precursor protein in aged rats: correlation with spatial memory deficits. Neuroscience 93(4):1409–1420. pii: S0306-4522(99)00244-4
Aydin D, Weyer SW, Muller UC (2012) Functions of the APP gene family in the nervous system: insights from mouse models. Exp Brain Res 217(3–4):423–434. doi:10.1007/s00221-011-2861-2
Bour A, Little S, Dodart JC, Kelche C, Mathis C (2004) A secreted form of the beta-amyloid precursor protein (sAPP695) improves spatial recognition memory in OF1 mice. Neurobiol Learn Mem 81(1):27–38. pii: S1074742703000716
Caldwell JH, Klevanski M, Saar M, Muller UC (2013) Roles of the amyloid precursor protein family in the peripheral nervous system. Mech Dev 130(6–8):433–446. doi:10.1016/j.mod.2012.11.001
Copanaki E, Chang S, Vlachos A, Tschape JA, Muller UC, Kogel D, Deller T (2010) sAPPalpha antagonizes dendritic degeneration and neuron death triggered by proteasomal stress. Mol Cell Neurosci 44(4):386–393. doi:10.1016/j.mcn.2010.04.007
Cousins SL, Hoey SE, Anne Stephenson F, Perkinton MS (2009) Amyloid precursor protein 695 associates with assembled NR2A- and NR2B-containing NMDA receptors to result in the enhancement of their cell surface delivery. J Neurochem 111(6):1501–1513. doi:10.1111/j.1471-4159.2009.06424.x
Cousins SL, Innocent N, Stephenson FA (2013) Neto1 associates with the NMDA receptor/amyloid precursor protein complex. J Neurochem 126(5):554–564. doi:10.1111/jnc.12280
Crawley JN (2008) Behavioral phenotyping strategies for mutant mice. Neuron 57(6):809–818. doi:10.1016/j.neuron.2008.03.001
Dawson GR, Seabrook GR, Zheng H, Smith DW, Graham S, O’Dowd G, Bowery BJ, Boyce S, Trumbauer ME, Chen HY, Van der Ploeg LH, Sirinathsinghji DJ (1999) Age-related cognitive deficits, impaired long-term potentiation and reduction in synaptic marker density in mice lacking the beta-amyloid precursor protein. Neuroscience 90(1):1–13. pii: S0306-4522(98)00410-2
Deacon RM, Rawlins JN (2005) Hippocampal lesions, species-typical behaviours and anxiety in mice. Behav Brain Res 156(2):241–249. doi:10.1016/j.bbr.2004.05.027
Deyts C, Vetrivel KS, Das S, Shepherd YM, Dupre DJ, Thinakaran G, Parent AT (2012) Novel GalphaS-protein signaling associated with membrane-tethered amyloid precursor protein intracellular domain. J Neurosci 32(5):1714–1729. doi:10.1523/JNEUROSCI.5433-11.2012
Dobrowolska JA, Kasten T, Huang Y, Benzinger TL, Sigurdson W, Ovod V, Morris JC, Bateman RJ (2014) Diurnal patterns of soluble amyloid precursor protein metabolites in the human central nervous system. PLoS One 9(3):e89998. doi:10.1371/journal.pone.0089998
Endres K, Fahrenholz F (2012) Regulation of alpha-secretase ADAM10 expression and activity. Exp Brain Res 217(3–4):343–352. doi:10.1007/s00221-011-2885-7
Fazeli MS, Breen K, Errington ML, Bliss TV (1994) Increase in extracellular NCAM and amyloid precursor protein following induction of long-term potentiation in the dentate gyrus of anaesthetized rats. Neurosci Lett 169(1–2):77–80
Fogel H, Frere S, Segev O, Bharill S, Shapira I, Gazit N, O’Malley T, Slomowitz E, Berdichevsky Y, Walsh DM, Isacoff EY, Hirsch JA, Slutsky I (2014) APP homodimers transduce an amyloid-beta-mediated increase in release probability at excitatory synapses. Cell Rep 7(5):1560–1576. doi:10.1016/j.celrep.2014.04.024
Furukawa K, Sopher BL, Rydel RE, Begley JG, Pham DG, Martin GM, Fox M, Mattson MP (1996) Increased activity-regulating and neuroprotective efficacy of alpha-secretase-derived secreted amyloid precursor protein conferred by a C-terminal heparin-binding domain. J Neurochem 67(5):1882–1896
Gakhar-Koppole N, Hundeshagen P, Mandl C, Weyer SW, Allinquant B, Muller U, Ciccolini F (2008) Activity requires soluble amyloid precursor protein alpha to promote neurite outgrowth in neural stem cell-derived neurons via activation of the MAPK pathway. Eur J Neurosci 28(5):871–882. doi:10.1111/j.1460-9568.2008.06398.x
Goebbels S, Bormuth I, Bode U, Hermanson O, Schwab MH, Nave KA (2006) Genetic targeting of principal neurons in neocortex and hippocampus of NEX-Cre mice. Genesis 44(12):611–621. doi:10.1002/dvg.20256
Heber S, Herms J, Gajic V, Hainfellner J, Aguzzi A, Rulicke T, von Kretzschmar H, von Koch C, Sisodia S, Tremml P, Lipp HP, Wolfer DP, Muller U (2000) Mice with combined gene knock-outs reveal essential and partially redundant functions of amyloid precursor protein family members. J Neurosci 20(21):7951–7963
Herms J, Anliker B, Heber S, Ring S, Fuhrmann M, Kretzschmar H, Sisodia S, Muller U (2004) Cortical dysplasia resembling human type 2 lissencephaly in mice lacking all three APP family members. EMBO J 23(20):4106–4115
Hoe HS, Fu Z, Makarova A, Lee JY, Lu C, Feng L, Pajoohesh-Ganji A, Matsuoka Y, Hyman BT, Ehlers MD, Vicini S, Pak DT, Rebeck GW (2009) The effects of amyloid precursor protein on postsynaptic composition and activity. J Biol Chem 284(13):8495–8506. doi:10.1074/jbc.M900141200
Hoe HS, Lee HK, Pak DT (2012) The upside of APP at synapses. CNS Neurosci Ther 18(1):47–56. doi:10.1111/j.1755-5949.2010.00221.x
Hoey SE, Williams RJ, Perkinton MS (2009) Synaptic NMDA receptor activation stimulates alpha-secretase amyloid precursor protein processing and inhibits amyloid-beta production. J Neurosci 29(14):4442–4460. doi:10.1523/JNEUROSCI.6017-08.2009
Ishida A, Furukawa K, Keller JN, Mattson MP (1997) Secreted form of beta-amyloid precursor protein shifts the frequency dependency for induction of LTD, and enhances LTP in hippocampal slices. Neuroreport 8(9–10):2133–2137
Jung CK, Herms J (2012) Role of APP for dendritic spine formation and stability. Exp Brain Res 217(3–4):463–470. doi:10.1007/s00221-011-2939-x
Klevanski M, Saar M, Baumkotter F, Weyer SW, Kins S, Muller UC (2014) Differential role of APP and APLPs for neuromuscular synaptic morphology and function. Mol Cell Neurosci 61C:201–210. doi:10.1016/j.mcn.2014.06.004
Kogel D, Deller T, Behl C (2012) Roles of amyloid precursor protein family members in neuroprotection, stress signaling and aging. Exp Brain Res 217(3–4):471–479. doi:10.1007/s00221-011-2932-4
Lannfelt L, Basun H, Wahlund LO, Rowe BA, Wagner SL (1995) Decreased alpha-secretase-cleaved amyloid precursor protein as a diagnostic marker for Alzheimer’s disease. Nat Med 1(8):829–832
Lassek M, Weingarten J, Einsfelder U, Brendel P, Muller U, Volknandt W (2013) Amyloid precursor proteins are constituents of the presynaptic active zone. J Neurochem 127(1):48–56. doi:10.1111/jnc.12358
Lee KJ, Moussa CE, Lee Y, Sung Y, Howell BW, Turner RS, Pak DT, Hoe HS (2010) Beta amyloid-independent role of amyloid precursor protein in generation and maintenance of dendritic spines. Neuroscience 169(1):344–356. doi:10.1016/j.neuroscience.2010.04.078
Lichtenthaler SF, Haass C, Steiner H (2011) Regulated intramembrane proteolysis—lessons from amyloid precursor protein processing. J Neurochem 117(5):779–796. doi:10.1111/j.1471-4159.2011.07248.x
Lorent K, Overbergh L, Moechars D, De Strooper B, Van Leuven F, Van den Berghe H (1995) Expression in mouse embryos and in adult mouse brain of three members of the amyloid precursor protein family, of the alpha-2-macroglobulin receptor/low density lipoprotein receptor-related protein and of its ligands apolipoprotein E, lipoprotein lipase, alpha-2-macroglobulin and the 40,000 molecular weight receptor-associated protein. Neuroscience 65(4):1009–1025
Magara F, Muller U, Li ZW, Lipp HP, Weissmann C, Stagljar M, Wolfer DP (1999) Genetic background changes the pattern of forebrain commissure defects in transgenic mice underexpressing the beta-amyloid-precursor protein. Proc Natl Acad Sci USA 96(8):4656–4661
Mallm JP, Tschape JA, Hick M, Filippov MA, Muller UC (2010) Generation of conditional null alleles for APP and APLP2. Genesis 48(3):200–206. doi:10.1002/dvg.20601
Marcello E, Gardoni F, Mauceri D, Romorini S, Jeromin A, Epis R, Borroni B, Cattabeni F, Sala C, Padovani A, Di Luca M (2007) Synapse-associated protein-97 mediates alpha-secretase ADAM10 trafficking and promotes its activity. J Neurosci 27(7):1682–1691. doi:10.1523/JNEUROSCI.3439-06.2007
Matrone C, Luvisetto S, La Rosa LR, Tamayev R, Pignataro A, Canu N, Yang L, Barbagallo AP, Biundo F, Lombino F, Zheng H, Ammassari-Teule M, D’Adamio L (2012) Tyr682 in the Abeta-precursor protein intracellular domain regulates synaptic connectivity, cholinergic function, and cognitive performance. Aging Cell 11(6):1084–1093. doi:10.1111/acel.12009
Meziane H, Dodart JC, Mathis C, Little S, Clemens J, Paul SM, Ungerer A (1998) Memory-enhancing effects of secreted forms of the beta-amyloid precursor protein in normal and amnestic mice. Proc Natl Acad Sci USA 95(21):12683–12688
Midthune B, Tyan SH, Walsh JJ, Sarsoza F, Eggert S, Hof PR, Dickstein DL, Koo EH (2012) Deletion of the amyloid precursor-like protein 2 (APLP2) does not affect hippocampal neuron morphology or function. Mol Cell Neurosci 49(4):448–455. doi:10.1016/j.mcn.2012.02.001
Milosch N, Tanriover G, Kundu A, Rami A, Francois JC, Baumkotter F, Weyer SW, Samanta A, Jaschke A, Brod F, Buchholz CJ, Kins S, Behl C, Muller UC, Kogel D (2014) Holo-APP and G-protein-mediated signaling are required for sAPPalpha-induced activation of the Akt survival pathway. Cell Death Dis 5:e1391. doi:10.1038/cddis.2014.352
Muller UC, Zheng H (2012) Physiological functions of APP family proteins. Cold Spring Harb Perspect Med 2(2):a006288. doi:10.1101/cshperspect.a006288
Obregon D, Hou H, Deng J, Giunta B, Tian J, Darlington D, Shahaduzzaman M, Zhu Y, Mori T, Mattson MP, Tan J (2012) Soluble amyloid precursor protein-alpha modulates beta-secretase activity and amyloid-beta generation. Nat Commun 3:777. doi:10.1038/ncomms1781
Priller C, Mitteregger G, Paluch S, Vassallo N, Staufenbiel M, Kretzschmar HA, Jucker M, Herms J (2009) Excitatory synaptic transmission is depressed in cultured hippocampal neurons of APP/PS1 mice. Neurobiol Aging 30(8):1227–1237. doi:10.1016/j.neurobiolaging.2007.10.016
Prox J, Bernreuther C, Altmeppen H, Grendel J, Glatzel M, D’Hooge R, Stroobants S, Ahmed T, Balschun D, Willem M, Lammich S, Isbrandt D, Schweizer M, Horre K, De Strooper B, Saftig P (2013) Postnatal disruption of the disintegrin/metalloproteinase ADAM10 in brain causes epileptic seizures, learning deficits, altered spine morphology, and defective synaptic functions. J Neurosci 33(32):12915–12928. doi:10.1523/JNEUROSCI.5910-12.2013 (12928a)
Prox J, Rittger A, Saftig P (2012) Physiological functions of the amyloid precursor protein secretases ADAM10, BACE1, and Presenilin. Exp Brain Res 217(3–4):331–341. doi:10.1007/s00221-011-2952-0
Puzzo D, Privitera L, Leznik E, Fa M, Staniszewski A, Palmeri A, Arancio O (2008) Picomolar amyloid-beta positively modulates synaptic plasticity and memory in hippocampus. J Neurosci 28(53):14537–14545. doi:10.1523/JNEUROSCI.2692-08.2008
Ring S, Weyer SW, Kilian SB, Waldron E, Pietrzik CU, Filippov MA, Herms J, Buchholz C, Eckman CB, Korte M, Wolfer DP, Muller UC (2007) The secreted beta-amyloid precursor protein ectodomain APPs alpha is sufficient to rescue the anatomical, behavioral, and electrophysiological abnormalities of APP-deficient mice. J Neurosci 27(29):7817–7826. doi:10.1523/JNEUROSCI.1026-07.2007
Seabrook GR, Smith DW, Bowery BJ, Easter A, Reynolds T, Fitzjohn SM, Morton RA, Zheng H, Dawson GR, Sirinathsinghji DJ, Davies CH, Collingridge GL, Hill RG (1999) Mechanisms contributing to the deficits in hippocampal synaptic plasticity in mice lacking amyloid precursor protein. Neuropharmacology 38(3):349–359. pii: S0028390898002044
Selkoe DJ (2002) Alzheimer’s disease is a synaptic failure. Science 298(5594):789–791. doi:10.1126/science.1074069
Soba P, Eggert S, Wagner K, Zentgraf H, Siehl K, Kreger S, Lower A, Langer A, Merdes G, Paro R, Masters CL, Muller U, Kins S, Beyreuther K (2005) Homo- and heterodimerization of APP family members promotes intercellular adhesion. EMBO J 24(20):3624–3634
Spires-Jones T, Knafo S (2012) Spines, plasticity, and cognition in Alzheimer’s model mice. Neural Plast 2012:319836. doi:10.1155/2012/319836
Steigerwald F, Schulz TW, Schenker LT, Kennedy MB, Seeburg PH, Kohr G (2000) C-Terminal truncation of NR2A subunits impairs synaptic but not extrasynaptic localization of NMDA receptors. J Neurosci 20(12):4573–4581
Steiner P, Higley MJ, Xu W, Czervionke BL, Malenka RC, Sabatini BL (2008) Destabilization of the postsynaptic density by PSD-95 serine 73 phosphorylation inhibits spine growth and synaptic plasticity. Neuron 60(5):788–802. doi:10.1016/j.neuron.2008.10.014
Suh J, Choi SH, Romano DM, Gannon MA, Lesinski AN, Kim DY, Tanzi RE (2013) ADAM10 missense mutations potentiate beta-amyloid accumulation by impairing prodomain chaperone function. Neuron 80(2):385–401. doi:10.1016/j.neuron.2013.08.035
Taylor CJ, Ireland DR, Ballagh I, Bourne K, Marechal NM, Turner PR, Bilkey DK, Tate WP, Abraham WC (2008) Endogenous secreted amyloid precursor protein-alpha regulates hippocampal NMDA receptor function, long-term potentiation and spatial memory. Neurobiol Dis 31(2):250–260. doi:10.1016/j.nbd.2008.04.011
Terry RD, Masliah E, Salmon DP, Butters N, DeTeresa R, Hill R, Hansen LA, Katzman R (1991) Physical basis of cognitive alterations in Alzheimer’s disease: synapse loss is the major correlate of cognitive impairment. Ann Neurol 30(4):572–580. doi:10.1002/ana.410300410
Tyan SH, Shih AY, Walsh JJ, Maruyama H, Sarsoza F, Ku L, Eggert S, Hof PR, Koo EH, Dickstein DL (2012) Amyloid precursor protein (APP) regulates synaptic structure and function. Mol Cell Neurosci 51(1–2):43–52. doi:10.1016/j.mcn.2012.07.009
von Koch CS, Zheng H, Chen H, Trumbauer M, Thinakaran G, van der Ploeg LH, Price DL, Sisodia SS (1997) Generation of APLP2 KO mice and early postnatal lethality in APLP2/APP double KO mice. Neurobiol Aging 18(6):661–669
Walsh DM, Minogue AM, Sala Frigerio C, Fadeeva JV, Wasco W, Selkoe DJ (2007) The APP family of proteins: similarities and differences. Biochem Soc Trans 35(Pt 2):416–420. doi:10.1042/BST0350416
Wang P, Yang G, Mosier DR, Chang P, Zaidi T, Gong YD, Zhao NM, Dominguez B, Lee KF, Gan WB, Zheng H (2005) Defective neuromuscular synapses in mice lacking amyloid precursor protein (APP) and APP-Like protein 2. J Neurosci 25(5):1219–1225
Weyer SW, Klevanski M, Delekate A, Voikar V, Aydin D, Hick M, Filippov M, Drost N, Schaller KL, Saar M, Vogt MA, Gass P, Samanta A, Jaschke A, Korte M, Wolfer DP, Caldwell JH, Muller UC (2011) APP and APLP2 are essential at PNS and CNS synapses for transmission, spatial learning and LTP. EMBO J 30(11):2266–2280. doi:10.1038/emboj.2011.119
Weyer SW, Zagrebelsky M, Herrmann U, Hick M, Ganss L, Gobbert J, Gruber M, Altmann C, Korte M, Deller T, Muller UC (2014) Comparative analysis of single and combined APP/APLP knockouts reveals reduced spine density in APP-KO mice that is prevented by APPsalpha expression. Acta Neuropathol Commun 2(1):36. doi:10.1186/2051-5960-2-36
Wilhelm BG, Mandad S, Truckenbrodt S, Krohnert K, Schafer C, Rammner B, Koo SJ, Classen GA, Krauss M, Haucke V, Urlaub H, Rizzoli SO (2014) Composition of isolated synaptic boutons reveals the amounts of vesicle trafficking proteins. Science 344(6187):1023–1028. doi:10.1126/science.1252884
Yang L, Wang Z, Wang B, Justice NJ, Zheng H (2009) Amyloid precursor protein regulates Cav1.2 L-type calcium channel levels and function to influence GABAergic short-term plasticity. J Neurosci 29(50):15660–15668. doi:10.1523/JNEUROSCI.4104-09.2009
Young-Pearse TL, Chen AC, Chang R, Marquez C, Selkoe DJ (2008) Secreted APP regulates the function of full-length APP in neurite outgrowth through interaction with integrin beta1. Neural Dev 3:15. doi:10.1186/1749-8104-3-15
Zhang X, Herrmann U, Weyer SW, Both M, Muller UC, Korte M, Draguhn A (2013) Hippocampal network oscillations in APP/APLP2-deficient mice. PLoS One 8(4):e61198. doi:10.1371/journal.pone.0061198
Acknowledgments
We are grateful to Julia Gobbert, Michael Neumann, Claudia Meyer and Inger Drescher for excellent technical assistance. We thank Paul Mathews for kindly providing the M3.2 antibody and Klaus-Armin Nave for providing NexCre mice. We would also like to thank the Nikon Imaging Center (University of Heidelberg) for support with confocal microscopy and image analysis. This work was supported by the Deutsche Forschungsgemeinschaft Grants (MU 1457/8-1 and MU 1457/9-1, 9-2 to UM; KO 1674/3-1, 3-2 to MK; DR 326/7-1, 7-2 to AD), the ERA-Net Neuron (01EW1305A to UM) and the Breuer Stiftung (to UM). DPW is a member of the Zurich Center of Integrative Human Physiology ZIHP and the Neuroscience Center Zürich.
Conflict of interest
The authors declare no competing financial interests.
Author information
Authors and Affiliations
Corresponding author
Additional information
M. Hick and U. Herrmann have equal contribution.
M. Korte and U. C. Müller shared senior authorship.
Electronic supplementary material
Below is the link to the electronic supplementary material.
Rights and permissions
About this article
Cite this article
Hick, M., Herrmann, U., Weyer, S.W. et al. Acute function of secreted amyloid precursor protein fragment APPsα in synaptic plasticity. Acta Neuropathol 129, 21–37 (2015). https://doi.org/10.1007/s00401-014-1368-x
Received:
Revised:
Accepted:
Published:
Issue Date:
DOI: https://doi.org/10.1007/s00401-014-1368-x