Abstract
The average orientation of a liquid crystal (LC) director to the grating formation, morphology, and switching properties of a holographic polymer-dispersed liquid crystal (HPDLC) grating was systematically investigated in this study. The grating possessed high diffraction efficiency and low scattering with the LC director being parallel to the grating vector. The scanning electron microscope confirmed the well-defined morphology with the LC director being parallel to the grating vector. The grating was easily switched when the LC director was perpendicular to the grating vector. Moreover, polarization excitation was performed to investigate the polarization dependence behavior of the HPDLC-distributed feedback (DFB) laser. The results confirmed that the HPDLC grating is suitable as a laser oscillation when the LC director is parallel to the grating vector. Finally, the tuning range was obtained for the HPDLC DFB laser by applying an external electric field. The tunability, ease of fabrication, and mass production make the HPDLC DFB lasers suitable as smart laser sources for spectroscopy and communication.
Similar content being viewed by others
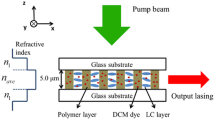
Explore related subjects
Discover the latest articles, news and stories from top researchers in related subjects.Avoid common mistakes on your manuscript.
1 Introduction
Holographic polymer-dispersed liquid crystal (HPDLC) gratings are promising multifunctional materials and have recently attracted increasing scientific attention [1,2,3,4]. HPDLC gratings are created under conventional two- or multi-coherent laser beams by holography [1]. The polymerization-induced phase separation (PIPS) making the polymer and liquid crystal (LC) mainly segregates into constructive and destructive interference zones, respectively. Then, a periodic configuration of alternating polymer and LC layers, namely HPDLC gratings, emerges [1]. Their interesting qualities, such as high diffraction efficiency, external field tunability [5], ease of fabrication, mass production, and cost-effectiveness, make HPDLC gratings extremely suitable for use in display [6], telecommunication [7], lens [8], and laser oscillation cavity [9] fields.
Fasanella et al. [10] reported that the molecular orientation of the nematic liquid crystal E7 in polymer liquid crystal polymer slices is perpendicular to the polymeric slices with Raman spectroscopy. The orientation is ascribed to anchoring of the LC molecules at the LC–polymer interface [11]. Moreover, the molecular orientation of the liquid crystals in HPDLC is perpendicular to the polymer wall because the diffraction efficiency for p-polarization probe light is as high as 90%, whereas it is nearly zero for s-polarization light [12]. The experimental explanation for the orientation of LC in HPDLC is the scaffolding effect of the polymer network, which is attributed to the planar anchoring of polymer filaments to LC molecules [13]. The effects of material systems on the polarization behavior of HPDLC have been studied [14]. However, reports demonstrating the orientation effect of liquid crystal on the performance of HPDLC gratings and HPDLC-distributed feedback (DFB) laser operation properties have been insufficient [9, 15], especially for the orientation of LC along polymer walls.
In this work, the average orientation of the LC director to the grating formation, its morphology, and switching properties of the HPDLC grating were systematically investigated. The grating formation properties were obtained by investigating the polarization-dependent diffraction efficiency using a 632.8 nm He–Ne laser. Scanning electron microscope (SEM) was used to investigate the morphology of the HPDLC gratings. Moreover, polarization excitation was performed to investigate the polarization-dependent behavior of the HPDLC DFB lasers. Finally, tuning experiments were performed on the HPDLC DFB lasers by applying an external electric field. Its tunability, ease of fabrication, and mass production make the HPDLC DFB laser suitable as a smart laser source for spectroscopy and communication.
2 Experimental
2.1 Materials
The mixture used to fabricate the HPDLC grating mainly contained monomer di-pentaerythritol penta/hexa acrylate (DPHA, 58.8 wt%, Aldrich) or phthalic diglycol di-acrylate (PDDA, 58.8 wt%, Eastern Acrylic Chem), liquid crystal (TEB30A, n o = 1.522 and Δn = 0.170 at 589.0 nm, 29.4 wt%, Silichem), crosslinking agent N-vinylpyrrolidone (NVP, 9.8 wt%, Aldrich), co-initiator N-phenylglycine (NPG, 1.5 wt%, Aldrich), and photo-initiator Rose Bengal (RB, 0.5 wt% Aldrich) [1, 12,15]. All the materials were used as received and without further purification. The mixture was stirred for 24 h and injected into a clean sample glass cell via calibration action in a darkroom. The thickness of sample cells was controlled at 9 μm using spacers. The refractive indexes of the mixture after illumination were 1.536 and 1.548 for the DPHA and PDDA-fabricated mixtures, respectively (2WA, Kernco). An indium tin oxide-coated sample cell was used so that the electric field could be applied to the sample to perform switching experiments. For the lasing application, the laser dye [4-(dicyanomethylene)-2-methyl-6-(p-dimethylaminostyryl)-4H-pyran] (DCM) was doped into the pre-polymerization mixture at a weight ratio at 0.5% to optimize the lasing performance because the laser dye showed a concentration quenching phenomenon [16]. Figure 1 depicts the chemical structure of the monomers used in this study.
2.2 HPDLC grating fabrication and characterization
Figure 2a shows the schematic experimental setup for HPDLC fabrication and characterization. A conventional two-coherent beam interference configuration, delivered from a continuous s-polarized neodymium-doped yttrium aluminum garnet (Nd3+:YAG, second-harmonic generation [17]) 532 nm laser (New Industries Optoelectronics), was used to fabricate the HPDLC via PIPS [1]. The main incident laser beams were expanded and filtered into a uniform plane wave. Then, the beams were split into object and reference beams. The interference between the object and reference beams created the HPDLC gratings. The grating period Λ was determined by the intersection angle between the object and the reference beams, which is expressed as [9]:
where λ rec is the recording laser wavelength in vacuum and the angle θ is half of the intersection angle, as shown in Fig. 2a. The recording time was 60 s for every sample. Moreover, the experiment temperature for HPDLC grating fabrication was 303 K. The optimum recording intensity values were 2.2 and 2.5 mW/cm2 for PDDA and DPHA fabricated HPDLC gratings, respectively.
A 632.8 nm He–Ne laser with an incident at the Bragg angle was used to investigate the diffraction properties for fabricating HPDLC [15]. Detectors 1 and 2 were used to record the s- and p-polarized diffracted beam intensities in real time. Furthermore, detectors 3 and 4 were used to record the p- and s-polarized transmitted beam intensities in real time. The incident beam intensities were recorded before impinging onto the sample. The diffraction efficiency η was defined as:
where I dif and I inc are the diffraction and incident intensities. Moreover, the scattering α is defined as:
where I tra and I ref are the transmittance and reflectance intensities, respectively.
Figure 2b represents the schematic illustration setup of switching experiments. The s- or p-polarized incident lights impinged the HPDLC gratings at the Bragg angle θ Bragg, which was delivered from a continuous He–Ne laser. A variable transformer was used to control the electric field that was continuously applied to the HPDLC gratings. The transmittance and diffraction beam intensities were investigated using detectors 1 and 2 in real time.
2.3 HPDLC SEM characterization
The HPDLC grating samples were fractured and immersed in ethanol for 24 h to remove the LC. The samples were dried in a clean room after removing the LC. The sample surfaces were evaporated using Au nanoparticles to enhance electro-conductivity before SEM testing. The SEM characterizations were performed using a Hitachi S-4800 SEM.
2.4 HPDLC DFB laser excitation
Figure 2c depicts the schematic illustration of the experimental setup for HPDLC DFB laser excitation. A 532 nm Q-switched frequency-doubled Nd3+:YAG pulsed laser (New Industries Optoelectronics) with a pulse duration of 8 ns and repetition at 10 Hz was used as the excitation source to transversely excite the HPDLC DFB laser, as shown in Fig. 2c. The incident beams were expanded with a reverse telescope system to deliver a uniform plane wave. An attenuator and polarizers were used to regulate the beam energy and polarization. Then, the incident beams were filtered using an adjustable slit to select the central part for uniform excitation. Moreover, the incident beams were reshaped using a cylindrical lens into a 3 by 1 mm linear spot to excite the HPDLC DFB laser. The lasing emission was collected into a fiber-coupled spectrometer (Sofn Instruments) with a solution at 0.23 nm. Furthermore, part of the energy was split off into an energy meter using a beam splitter to investigate the excitation energy in real time. Figure 2d shows the device configuration for HPDLC DFB laser, which has a low refractive index cover glass, low refractive index substrate glass plate, and high refractive index dye-doped HPDLC grating. The grating period Λ and the orientation of grating vector G are illustrated in the schematic illustration. The lasing emission was excited from the sample edge.
3 Results and discussion
3.1 Grating formation property
Figure 3a shows the time evolution characterizations of the diffraction efficiency during the HPDLC grating formation with a grating period at 594 nm. The diffraction efficiency increased with time and peaked within several seconds. The grating diffraction efficiency values were 89.2 and 4.3% for the p- and s-polarized probe lights with DPHA as the monomer, respectively. Therefore, the director average orientation for LC molecules was deduced to be parallel to the grating vector, as shown in Fig. 2d. When the grating formation ended, the refractive index of the p-polarized light was approximate to the extraordinary refractive index n e, whereas the s-polarized light in the LC layer possessed an ordinary refractive index n o. As a result, the refractive index modulation between the liquid crystal layers and polymer layers for p-polarized light provided high diffraction efficiency. However, the refractive index modulation between the liquid crystal and polymer layers for the s-polarized light was approximately zero, thereby leading to the low diffraction efficiency [1]. The grating diffraction efficiency values were 78.1 and 3.6% for s- and p-polarized probe lights with PDDA as the monomer, respectively. Thus, the LC director average orientation was perpendicular to the grating vector, e.g., the LC molecules were along the grating grooves for the PDDA-fabricated grating. As for the LC director average orientation that was perpendicular to the grating vector, the refractive index of the s-polarized light was approximate to the extraordinary refractive index n e, whereas the p-polarized light possessed an ordinary refractive index n o. As a result, the refractive index modulation between the liquid crystal and polymer layers for the s-polarized light contributed to the high diffraction efficiency. However, the refractive index modulation between the liquid crystal and polymer layers for p-polarized light was approximately zero, which led to the low diffraction efficiency. The experimental results indicated that the diffraction efficiency is increased when the LC director average orientation is parallel to the grating vector. The induction time is the duration of oxygen depletion. After which, the LC becomes immiscible and exhibits phase separation from the mixture of the monomer and polymer accompanied by grating formation [1, 18, 19]. The induction time values for grating formation were 9.5 and 15 s for the LC director average parallel and perpendicular to the grating vector. The long induction time confirmed that the grating formation was insensitive to the recording illumination with the LC director average orientation being perpendicular to the grating vector.
Figure 3b shows the time evolution characterization of the scatterings in the grating formation period. With the grating formation, the miscible LC phase was separated because of the conversion of the chemical potential [19]; moreover, the uniform mixture began to show anisotropic properties, and finally, scatterings occurred [20, 21]. The scattering values were 3.1 and 1.4% for the p- and s-polarized probe lights when the LC director average orientation was parallel to the grating vector. The scattering values were 4.4 and 9% for the p- and s-polarized probe light when the LC director average orientation was perpendicular to the grating vector. The results confirmed that the scatterings were reduced when the LC director average orientation was parallel to the grating vector.
3.2 Morphology characterization with SEM
The surface morphology of the HPDLC grating is believed to be one of the most important properties in the HPDLC DFB laser. Therefore, we investigated the morphology of the HPDLC grating using SEM. Figure 4 shows the SEM images of the HPDLC grating samples with the period at 594 nm; the volume grating configuration can be seen in Fig. 3c, d. Images (a) and (c) are the top and side views, respectively, of the grating sample when the LC director average orientation was perpendicular to the grating vector. Images (b) and (d) are the top and side views, respectively, of the grating sample when the LC director average orientation was parallel to the grating vector. The dark zone is mainly the LC layer, which was removed during the sample preparation period. In Fig. 4a, c, the grating with the LC director average orientation that was perpendicular to the grating vector showed an imperfect outline and a rough and unclear interface, thereby contributing to the strong scattering shown in Fig. 3b. The morphology is smooth and clear for the grating with the LC director average orientation that was parallel to the grating vector, as shown in Fig. 4b, d. Figure 4b is not as clear as Fig. 4a because the surface relief [22] is shallow (several nanometers) and the SEM cannot capture the image clearly, uncovering the stable configuration with low volume shrinkage for the LC director average orientation parallel to the grating vector [23]. The morphology imaged by SEM indicated that the grating should possess high diffraction efficiency and low scattering in the LC director average orientation parallel to the grating vector, as shown in Fig. 3.
3.3 Grating electric switching performance
The HPDLC grating can be switched using external electric fields because the positive nematic LC director average orientation is sensitive to electric fields. The critical field E c is expressed as [1]:
where a, \( \sigma_{\text{LC}} \), \( \sigma_{\text{P}} \), k 33, \( \ell \), and \( \Delta \varepsilon \) are the length of LC semi-major axis, the LC low frequency conductivity, the polymer matrix low frequency conductivity, the LC pure bend elastic force constant, the aspect ratio of LC, and the LC dielectric anisotropy, respectively.
Figure 5a shows the grating-normalized diffraction efficiency as a function of the driving electric field. Both the switching curves decreased with the external switching electric field and finally stabilized. The threshold electric field E 90 and saturated electric field E 10 are defined as the electric fields where the diffraction efficiency decreases to 90 and 10% of the initial value. The E 90 values are 1.2 and 11 V/μm for the LC director average orientations that were perpendicular and parallel to the grating vector, respectively, whereas the saturated electric field values were 3.0 and 22.1 V/μm for the LC director average orientation that were perpendicular and parallel to the grating vector, respectively. The gratings tended to be switched easily when the LC director average orientation was perpendicular to the grating vector. Moreover, the grating was insensitive to the external switching electric field when the LC director average orientation was parallel to the grating vector.
Switching experiments. a Normalized diffraction efficiency as a function of the driving electric field. Schematic illustration of HPDLC grating diffraction, switching off with application of electric field and orientation of LC for LC director average orientation b parallel and c perpendicular to the grating vector
Figure 5b, c show the schematic illustration of the grating diffraction and the switching properties by applying external electric field for LC director average orientation parallel and perpendicular to the grating vector, respectively. The grating regime is classified by the Klein–Cook parameter Q [1, 24]:
where λ p is the probe wavelength in vacuum, L is the thickness, n ave is the average refractive index, and Λ is the period of the grating. When Q ≫ 1, the grating is the Bragg regime whereas the grating is the Raman–Nath regime for Q ≪ 1. For the grating diffraction experiments, λ p was 632.8 nm, L was 9 μm, n eff was ~1.54, and Λ was 600 nm. Substituting the parameter values into Eq. (5), the Q was ~64; thus, the gratings belong to the Bragg regime.
For the Bragg regime grating, the grating diffraction is determined by the Bragg equation [25]:
where m is the diffraction order and θ Bragg is the Bragg angle of the grating. The value of m corresponds to 1 or −1. For m = 1, there is the 1st order diffraction light and the 0th transmittance light when the incident light matches the Bragg angle. As for m = −1, there is the −1st order diffraction light and the 0th transmittance light when the incident light matches the Bragg angle. Nevertheless, they were symmetrical and experimental results for diffraction efficiency were identical. Moreover, the incident light was effectively diffractive when the incident angle matched the Bragg angle. In the meantime, the diffraction angle equals the Bragg angle, as shown in Fig. 5b, c. For the LC director average orientation that was parallel to the grating vector, the grating was sensitive to p-polarized incident light and insensitive to s-polarized incident light, as shown in Fig. 5b. As for the LC director average orientation that was perpendicular to the grating vector, the grating was sensitive to s-polarized incident light and insensitive to p-polarized incident light, as shown in Fig. 5c. The grating was switched off when it was subjected to the electric field because the LC director average orientation was along the direction of the external electric field (n o ≈ n p); furthermore, the refractive index modulation to convert the incident light to diffraction light was insufficient [24]. The diffraction efficiency of the first-order diffraction light to probe the wavelength for the LC director average orientation that was parallel or perpendicular to the grating vector are summarized in Table 1. The diffraction efficiency decreased with the probe wavelength for p-polarization probe light. However, for s-polarization probe light, the diffraction efficiency increased with probe wavelength.
3.4 HPDLC DFB laser characterization
For the HPDLC DFB laser application [26,27,28,29], the dye-doped HPDLC gratings were fabricated via a one-step holographic technique [15, 25]. The HPDLC DFB laser device configuration is shown in Fig. 2d. The HPDLC DFB laser was transversely excited and the lasing emission was collected from the sample edge. According to the coupled-wave theory [24] of DFB lasers, the feedback is provided by the backward Bragg scattering [30]. The lasing wavelength is expressed as [9]:
where n eff is the effective refractive index of the lasing mode and m is the diffraction order. The full width at half maximum (FWHM) for the broad photoluminescence spectra of the laser dye DCM (F-7000FL, Hitachi) is 100 nm, as shown in Fig. 6a. The excitation wavelength was set to 480 nm to match the maximum absorbance of the laser dye DCM to obtain the fluorescence. The position of the amplified spontaneous emission (ASE), which was collected from the DCM doped polymer-dispersed liquid crystal (PDLC, the LC with random orientation in the polymer matrix) sample edge, possessed the largest net gain with a FWHM at 9 nm, as shown in Fig. 6a [31, 32]. Figure 6a shows the lasing spectra for the HPDLC DFB laser with the LC director average orientation that was parallel to the grating vector. The FWHM for the lasing spectra, centered at 610 nm, was 0.6 nm. Therefore, the narrowing of the spectra from 100 to 0.6 nm confirmed the mode selection and spectra compression for HPDLC. The HPDLC configuration has properties, such as cost-effectiveness, ease of fabrication, and mass production, that exhibit significant advantages over the complicated and expensive imprinting and molding of the DFB configuration fabrication process [33, 34].
Figure 6b shows the emission-pulse intensity as a function of excitation-pulse energy with crossed excitation polarizations for the HPDLC DFB laser with LC director average orientation that was parallel or perpendicular to the grating vector. The central wavelength was 610 nm for the LC director average orientations that were both parallel and perpendicular to the grating vector. The effective refractive indexes were 1.535 and 1.547 for the HPDLC DFB laser with LC director average orientations that were parallel and perpendicular to the grating vector, respectively. The diffraction order was of the third order. Moreover, the grating periods were set to 596 and 591.5 nm to obtain the lasing at 610 nm for the HPDLC DFB laser with LC director average orientations that were parallel and perpendicular to the grating vector, respectively. The lasing threshold values were 3.5 and 3.8 μJ/pulse when the s- and p-polarized excitations for LC director average orientation that was parallel to the grating vector, respectively. Moreover, the lasing threshold values were 4.5 and 6.5 μJ/pulse when the s- and p-polarization excitations for LC director average orientation that was perpendicular to the grating vector, respectively. Therefore, the experimental results indicated that the lasing threshold is decreased with s-polarized excitation for the HPDLC DFB laser with LC director average orientations both parallel and perpendicular to the grating vector. Furthermore, the lasing threshold was lower with the LC director average orientation that was parallel to grating vector than the LC director average orientation that was perpendicular to the grating vector. The lasing threshold was weakly sensitive to polarized excitation with the LC director average orientation that was parallel to the grating vector. However, the lasing threshold showed strong polarized excitation dependence with the LC director average orientation that was perpendicular to the grating vector, as shown in Fig. 6b. Nevertheless, the experimental results confirmed that the HPDLC with LC director average orientation that was parallel to the grating vector is suitable as an oscillation cavity.
3.5 Electrically tunable HPDLC DFB laser
Figure 7 shows the electrically tunable HPDLC DFB laser with the LC director average orientation that was parallel to the grating vector due to an applied external electric field. The LC director average orientation was reoriented gradually to the direction of the electric field when the electric field was applied, as shown in Fig. 5b. As a result, the refractive index modulation between the polymer and LC layers gradually decreased with the electric field. The lasing intensity decreased with external electric field, as shown in Fig. 7a. The narrow peaks confirmed the good lasing. The lasing wavelengths were 610, 610, 609.4, and 608.3 nm with electric fields at 0, 8.3, 16.7, and 20.8 V/μm, respectively. The decrease of the effective refractive index of the lasing mode was attributed to the blue-shifted lasing [35]. The tuning range reached as high as 1.7 nm. Moreover, the lasing threshold values were 3.5, 3.9, 4.7, and 6.4 μJ/pulse with electric fields at 0, 8.3, 16.7, and 20.8 V/μm, respectively. The reason for the increasing lasing threshold was the decrease of the coupling strength when an external field was applied [30]. The tunability, ease of fabrication, and mass production make the HPDLC DFB laser suitable as a smart laser source for spectroscopy and communication [36, 37].
4 Conclusions
In summary, the average orientation of the LC director to the grating formation, its morphology, and the switching properties of the HPDLC grating were systematically investigated. The experimental results indicated that the gratings possessed high diffraction efficiency and low scattering with the LC director being parallel to the grating vector. SEM imaging confirmed that the morphology was well-defined with the LC director being parallel to the grating vector. The grating was easy to switch off with the LC director being perpendicular to the grating vector. Moreover, the polarization excitation was performed to investigate the polarization dependence behavior of the HPDLC DFB laser. The results confirmed that the lasing threshold was reduced with LC director that was parallel to the grating vector. Finally, the 1.7 nm tuning range was obtained for the HPDLC DFB laser by applying an external electric field.
References
T.J. Bunning, L.V. Natarajan, V.P. Tondiglia, R.L. Sutherland, Annu. Rev. Mater. Sci. 30, 83 (2000)
Y.J. Liu, H.T. Dai, X.W. Sun, J. Mater. Chem. 21, 2982 (2011)
L. Sio, L. Ricciardi, S. Serak, M. La Deda, N. Tabiryan, C. Umeton, J. Mater. Chem. 22, 6669 (2012)
H.Y. Peng, M.L. Ni, S.G. Bi, Y.G. Liao, X.L. Xie, Rsc Adv. 4, 4420 (2014)
R.L. Sutherland, V.P. Tondiglia, L.V. Natarajan, T.J. Bunning, W.W. Adams, Appl. Phys. Lett. 64, 1074 (1994)
J. Qi, G.P. Crawford, Displays 25, 177 (2004)
S. Yeralan, J. Gunther, D. Ritums, R. Cid, M. Popovich, Opt. Eng. 41, 1774 (2002)
H.W. Ren, Y.H. Fan, S.T. Wu, Appl. Phys. Lett. 83, 1515 (2003)
M.H. Liu, Y.G. Liu, G.Y. Zhang, Z.H. Peng, D.Y. Li, J. Ma, L. Xuan, J. Phys. D Appl. Phys. 49, 465102 (2016)
M. Fasanella, E. Castriota, L.De Cazzanelli, R. Sio, C. Caputo, Umeton. Mol. Cryst. Liq. Cryst. 558, 46 (2012)
R. Caputo, L. De Sio, A. Veltri, C. Umeton, Opt. Lett. 29, 1261 (2004)
W. Huang, Y. Liu, Z. Diao, C. Yang, L. Yao, J. Ma, L. Xuan, Appl. Opt. 51, 4013 (2012)
M. Drevensek-Olenik, M.A. Fally, Ellabban. Phys. Rev. E 74, 021707 (2006)
K. Takeshi, T. Yoshinao, Jpn. J. Appl. Phys. 36, 6388 (1997)
W. Huang, Z. Diao, Y. Liu, Z. Peng, C. Yang, J. Ma, L. Xuan, Org. Electron. 13, 2307 (2012)
J. Cornil, D. Beljonne, J.P. Calbert, J.L. Bredas, Adv. Mater. 13, 1053 (2001)
T. Manaka, M. Iwamoto, Light Sci. Appl. 5, e16040 (2016)
C. Sánchez, M.J. Escuti, C. van Heesch, C.W.M. Bastiaansen, D.J. Broer, J. Loos, R. Nussbaumer, Adv. Funct. Mater. 15, 1623 (2005)
T.J. Bunning, L.V. Natarajan, V. Tondiglia, R.L. Sutherland, D.L. Vezie, W.W. Adams, Polymer 36, 2699 (1995)
R.L. Sutherland, V.P. Tondiglia, L.V. Natarajan, T.J. Bunning, Appl. Phys. Lett. 79, 1420 (2001)
R.L. Sutherland, V.P. Tondiglia, L.V. Natarajan, P.F. Lloyd, T.J. Bunning, J. Appl. Phys. 99, 123104 (2006)
C.M. Leewis, A.M. de Jong, L.J. van Ijzendoorn, D.J. Broer, J. Appl. Phys. 95, 4125 (2004)
E. Hata, Y. Tomita, Opt. Lett. 35, 396–398 (2010)
H. Kogelnik, Bell Syst. Tech. J. 48, 2909 (1969)
R. Jakubiak, T.J. Bunning, R.A. Vaia, L.V. Natarajan, V.P. Tondiglia, Adv. Mater. 15, 241 (2003)
C. Vannahme, M. Dufva, A. Kristensen, Light Sci. Appl. 4, e269 (2015)
S. Mhibik, D. Forget, G. Ott, I. Venus, L. Divliansky, S. Glebov, Chenais. Light Sci. Appl. 5, e16026 (2016)
Y.R. Fang, M.T. Sun, Light Sci. Appl. 4, e294 (2015)
A. Llobera, J. Juvert, A. Gonzalez-Fernandez, B. Ibarlucea, E. Carregal-Romero, S. Buttgenbach, C. Fernandez-Sanchez, Light Sci. Appl. 4, e271 (2015)
H. Kogelnik, C.V. Shank, Appl. Phys. Lett. 18, 152 (1971)
M.D. McGehee, R. Gupta, S. Veenstra, E.K. Miller, M.A. Diaz-Garcia, A.J. Heeger, Phys. Rev. B 58, 7035 (1998)
J.H. Son, B. Cho, S. Hong, S.H. Lee, O. Hoxha, A.J. Haack, L.P. Lee, Light Sci. Appl. 4, e280 (2015)
E.B. Namdas, M. Tong, P. Ledochowitsch, S.R. Mednick, J.D. Yuen, D. Moses, A.J. Heeger, Adv. Mater. 21, 799 (2009)
S. Hermann, R.C. Shallcross, K. Meerholz, Adv. Mater. 26, 6019 (2014)
Z. Diao, L. Kong, L. Xuan, J. Ma, Org. Electron. 27, 101 (2015)
T. Woggon, S. Klinkhammer, U. Lemmer, Appl. Phys. B Lasers Opt. 99, 47 (2010)
J. Clark, G. Lanzani, Nat. Photonics 4, 438 (2010)
Acknowledgements
This work is supported by the National Natural Science Foundation of China (61377032 and 61378075).
Author information
Authors and Affiliations
Corresponding author
Rights and permissions
About this article
Cite this article
Liu, M., Liu, Y., Peng, Z. et al. Self-orientation effect of liquid crystals on holographic polymer-dispersed liquid crystal and distributed feedback lasers. Appl. Phys. B 123, 208 (2017). https://doi.org/10.1007/s00340-017-6786-8
Received:
Accepted:
Published:
DOI: https://doi.org/10.1007/s00340-017-6786-8