Abstract
A novel conical output coupler for positive-branch unstable resonators has been proposed to improve the output beam quality. With the application of an inner cone annular scraper and an outer cone reflector, a realization of the output beam from unstable resonator with solid core is introduced. The feasibility of this conical output coupler with solid beam is examined numerically and experimentally with the help of a commercial fast transverse flow (FTF) CO2 laser. The characteristics of the output coupling beam in near field and far field are discussed. Under the definition of 86.5 % power content, the M 2pc factors of original annular beam and improved solid beam are 5.22 and 1.16 in simulation. In experiment, the M 2pc factor of the original annular beam from the commercial FTF CO2 laser is more than 10, while the M 2pc factor of the improved solid beam is 3.82. Both simulation and experimental results show that the beam quality can be improved a lot by introducing this output coupling configuration.
Similar content being viewed by others
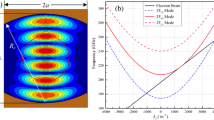
Avoid common mistakes on your manuscript.
1 Introduction
Unstable optical resonators have been applied in high power lasers, from a wide variety of continuous wave and pulse lasers, for its property of large mode volumes even in very short cavity, adjustable diffraction output coupling and good high-order modes discrimination [1]. Compared to stable resonators, the coupling output beam of conventional unstable resonators leaks around instead of passing through the output coupler. Due to the hard edge of the output coupler, the output beam is in a hard-edged ring shape, and this results many side lobes around the central intensity peak in the far field. The annular output coupling is not suitable for the large-bore, low-gain lasers such as CO2 lasers and chemical oxygen iodine lasers. Since the coupling is defined solely by the thickness of the ring, the resultant thin ring of low-gain lasers yields poor beam quality. Meanwhile, the output coupling beam depends on the resonator magnification M [2], which is defined by the radius of curvature of the back and the output mirror. By increasing the magnification M, more power is provided in the main lobe of the far field and therefore a better beam quality is obtained. However, this behavior results in a considerable decrease in output power [3]. In this case, conventional unstable resonators are not well suited for the use in high power lasers when good beam quality is necessary.
In order to improve the far-field performance of lasers with unstable resonator, many configurations have been developed, expecting to obtain near-diffraction-limited beam output [4–9]. Among these experimental setups, the relatively successful methods are unstable resonator with graded-reflectance mirrors [4, 5], unstable ring resonator with a 90° beam rotation [6, 7], hybrid resonator in a double-pass configuration [8] and unstable resonator with reduced output coupling [9]. The unstable resonator with graded-reflectance mirrors is helpful to improve the beam quality, but it is difficult to manufacture high damage threshold variable reflectivity dielectric films for high power optical devices. As far as the unstable ring resonator with a 90° beam rotation is concerned, the complexity and sensitivity of the optical setup increase. The output coupler of hybrid resonator in a double-pass configuration is off-axis rectangular incision, and the output beam is of rectangular cross section, which brings difficulties in practical applications. And an unstable resonator with reduced output coupling is effective in improving the beam quality in far field, while the output device is not so flexible.
In order to remove the central hole of the annular intensity distribution of the output beam from a stable resonator with annular gain medium, a transmitted axicon telescope was proposed [10] to improve the beam quality. However, this transmitted system is not suitable for the unstable resonator considering the aspect of high power. In this paper, we propose a novel output coupling configuration for the unstable resonator. This configuration consists of an inner cone annular scraper (ICAS) and an outer cone reflector (OCR). The key novelty of this method is to improve the beam quality outside the conventional unstable resonator, rather than changing the architecture of the resonator. And the basic principle of this method is using reflective optical elements to realize the reconstruction of central dark spot in the annular beam of the unstable resonator. In this literature, the original annular beam of the conventional positive-branch confocal unstable resonator is defined as the direct output beam, and the improved solid beam from the device is named as the coupling output beam.
This paper consists of several sections: In Sect. 2, the proposed output coupling configuration with positive-branch unstable resonator is described in detail. In Sect. 3, the simulation model and results of the proposed configuration are given. In Sect. 4, the fabrication of ICAS and OCR is described, and the experimental results of the proposed output coupling with positive-branch unstable resonator in commercial CO2 laser are discussed. Finally, the summary is given in the Sect. 5.
2 Design of the coupling configuration
A positive-branch setup is chosen here as this structure is the most widely approbatory and applied. The sketch of the output coupling configuration with a positive-branch confocal unstable resonator is shown in Fig. 1. The concave mirror M1 and the convex mirror M2 compose the positive-branch confocal unstable resonator. In front of M2, there is an inner cone annular scraper (ICAS) M3 with a certain axial section vertex, whose rotational symmetry axis is at an angle of 45° with the unstable resonator optical axis. The outer cone reflector (OCR) M4 is placed exactly under the M3, and the axial section vertex is the same as M3. The line O 3 O 4 between the center of M3 and the center of M4 is perpendicular to the unstable resonator optical axis O 1 O 2 . And S is defined as the vertical distance between the center line of M3 and the center line of M4.
In a conventional positive-branch confocal unstable resonator, the beam is expanded by a factor M (geometric magnification) times propagating from M1 to M2. The edge part of the expanding beam is reflected and coupled out by the ICAS when it transmits forward. And the remaining central part of the beam is coupled to the resonator and continues oscillating. Due to the convergence effect which the apex angle θ contributes to the beam, the direct output annular hollow beam turns to the direction perpendicular to the optical axis of the unstable resonator and gradually focuses on the position of the axis where the center of ICAS is located. The outer cone reflector M4 is parallel installed with the inner cone annular scraper, and the beam near the central axis O 3 O 4 is redirected in order to get the output beam with parallel to the unstable resonator axis direction. Circular solid laser beam can be obtained by adjusting the distance S between O 3 and O 4 precisely.
The sizes of the ICAS and OCR are much longer than the wavelength of the CO2 laser beam. There is a good enough approximation in geometrical optics. Based on the geometrical optics, we can make the conclusion that the beams on the input reference plane and the output reference plane have the same optical path difference, as shown in Fig. 2. The surface of the upper section of the ICAS AO 3 is parallel to the one of the OCR CO 4, while the surface of the lower section of the ICAS O 3 B is parallel to the one of the OCR O 4 D. Here, we define the input optical field of the coupling system as E 3(r 3, θ 3), and the output optical field as E 4(r 4, θ 4). In the approximation of geometrical optics, the transformation between any input ray (r 3, θ 3) in the input reference plane and its corresponding output ray (r 4, θ 4) in the output reference plane is given by
where R in is the inner radius of the ICAS. The dealing method of the conversion of the coupling system is the same as that in [11, 12]. The output beam E 4(r 4, θ 4)is given by
L OPD is the optical path difference between the two reference planes, j is the unit imaginary number, and Θ () is the Heaviside step function. For the purpose to transform the annular hollow beam to the circular solid beam, the vertical distance S between the center of ICAS and OCR is given by
where θ is the outer apex angle of the axis section in the ICAS and the inner apex angle of the axis section in the OCR, R in is the inner radius of the ICAS, as shown in Fig. 2.
3 Modeling and simulation
In order to analyze the improvement of beam quality in unstable resonators by introducing output coupling ICAS and OCR, this section is organized as follows. We first numerically simulate the beam transmission property of the output modes in conventional positive-branch confocal unstable resonator. In this part of simulation, we first investigate the diffraction integral equation in the positive-branch confocal unstable resonator from Collins formula [13].
For the case of circular lens, the aperture effect of the convex mirror M1 is ignored. The initial optical field on the inner surface of M2 is defined as E 2(x 0, y 0), and this field becomes E 2(x 1, y 1) after a round-trip transmission in the resonator. Under the self-reproduction condition [14], E 2(x 1, y 1) can be expressed by
where λ is the wavelength, γ is the loss factor, Σ is the integral region of the circular aperture on M2, and ABCD is the transmission matrix of rays’ round-trip in the positive-branch confocal unstable resonator, which is written as
where g 1 = 1 − L/R 1, g 2 = 1 − L/R 2, and the geometric magnification M = −R 1/R 2. By the coordinate transformation ξ 1 = x 1/a 2, η 1 = y 1/a 2, ξ 0 = x 0/a 2 and η 0 = y 0/a 2, Eq. (4) can be changed to be
where C is the integral region of unit circle, N c is the collimated Fresnel number [15]. And the equivalent Fresnel number N eq can be calculated by
The eigenvector method [16, 17] is exploited to simulate the optical field in the positive-branch confocal unstable resonator. And MATLAB [18] software is used for this simulation.
To compare the numerical results with the experimental ones, the parameters for all calculations were fixed as listed in Table 1.
As shown in Fig. 3a, the intensity distribution of annular mode (the direct output beam), which is coupling from the edge of the convex mirror M2 is given. And the intensity distribution at the position Y = 0 mm of the field is in Fig. 3b.
The direct output annular beam from the conventional unstable resonator is shaped to be a circular solid beam by this coupling output system. Considering the actual size of the optical components, the outer apex angle of the axis section in the ICAS and the inner apex angle of the axis section in the OCR are chosen to be 175.2°. The inner radius of ICAS R in is =15.5 mm and the outer radius R out of ICAS is =30 mm. Besides, the radius of OCR R 4 is =20 mm. Then, the central distance S is calculated to be 185.23 mm. Utilizing this transformation, the intensity distribution of the coupling output beam is obtained, as shown in Fig. 3c, and the corresponding curve at Y = 0 mm is shown in Fig. 3d. This simulation results show that the annular mode can be coupled to be the solid beam.
The propagation characteristics of the simulation output beam are discussed. In the evaluation of the beam quality methods, the M 2 factor based on the second-order intensity moment [19] and the M 2pc factor based on 86.5 % power content [20] are widely used in applications. The M 2 factor based on the second-order intensity moment can exhibit the characteristics of the near-field and far-field performance of the actual beam and possesses many advantages. However, there are some difficulties in measuring the beam which is not circular solid, because it is hard to obtain the size of the beam waist. In the process of assessing the beam quality of the output beam from the confocal unstable resonator, it is hard to measure due to the divergence problem [21]. Here, we adopt the 86.5 % power content method to assess the output beam of the coupling system of the confocal unstable resonator. The beam waist radius of the direct output annular laser beam is 20.01 mm. The intensity distributions of the direct output beam at different propagation distances are shown by red solid curves in Fig. 4a1–a6. Z R is the Rayleigh distance of the ideal Gaussian beam. The distances in Fig. 4a1–a6 are z = 0.1 Z R, 0.5 Z R, Z R, 5 Z R, 10 Z R, 20 Z R. In comparison, intensity distributions of the Gaussian beam with the same beam waist are shown by black dotted curves in Fig. 4a1–a6, while the beam waist radius of the coupling output beam is 4.54 mm, and intensity distributions of the coupling output beam are shown by red solid curves in Fig. 4b1–b6. Dark dotted curves in Fig. 4b1–b6 represent the intensity distributions of the Gaussian beam with the same power and beam waist radius as the improved beam. At different propagation distances z, the spot radius of the coupling output beam is compared with the spot radius of the ideal Gaussian beam with the same power and beam waist radius under the definition of 86.5 % power content, as shown in Fig. 5a, b.
a Intensity distributions of the direct output beam (red solid curve) and Gaussian beam (black dotted curve) with the same power and the 86.5 % power content radius, a1 z = 0.1Z R, a2 z = 0.5Z R, a3 z = Z R, a4 z = 5Z R, a5 z = 10Z R, a6 z = 20Z R; b intensity distributions of the coupling output beam (red solid curve) and Gaussian beam (black dotted curve) with the same power and the 86.5 % power content radius, b1 z = 0.1Z R, b2 z = 0.5Z R, b3 z = Z R, b4 z = 5Z R, b5 z = 10Z R, b6 z = 20Z R
According to the Fourier transform of lens [22], the distribution of the far-field intensity can be acquired at the rear focal plane by exploiting the ideal aberration-free lens to transform the coupling output solid beam. The far-field divergence angle of the coupling solid beam is 0.863 mrad, as shown in Fig. 6. The M 2pc factor of the 86.5 % power content is described as
where r pc and θ pc are the 86.5 % power content beam radii and far-field divergence angle. Thus, the M 2pc factor of the coupling output beam is 1.16. As shown in Fig. 5a, the far-field divergence is calculated to be 0.88. Then, the M 2pc factor of the original annular beam is calculated to be 5.22. In comparison, the coupling M 2pc factor of output beam has been improved 4.5 times with the original annular beam.
In conclusion, the beam quality of positive-branch unstable resonator is improved a lot by introducing this novel output coupling configuration, which consists of ICAS and OCR. The simulation result shows that the beam quality of the coupling output beam can reach up to 1.16 under the definition of 86.5 % power content.
4 Experimental results
Both mirrors are fabricated by processing the surface of copper mirror by the numerical-controlled lathe. The physical pictures of ICAS and OCR are shown as Fig. 7b, c, respectively. The experiments have been performed with a commercial fast transverse flow (FTF) CO2 laser (Goldsky, GS-TFL3000W). The initial resonator of the commercial FTF CO2 laser was of plane-concave structure. In order to measure the output characteristic of the coupling system, we modified this resonator to be a positive-branch confocal unstable resonator. The structural parameters of this modified resonator are shown in Table 1. The M 2pc factor of the original annular beam from the commercial modified FTF CO2 laser is more than 10. And the experimental setup is laser and is fixed on the cover of the laser cavity with the convex described in Fig. 7. The ICAS is located inside the FTF CO2 mirror, and the OCR is placed outside the laser. By adjusting the manual stages, the distance S between the ICAS and OCR can be changed flexibly.
We first investigated the performance of the confocal unstable resonator with a 45˚ plane reflector and ICAS. Limited by the experimental conditions, the spots of the output beam were measured by ablation. The experimental spot of the annular beam at the propagation distance Z = 600 mm is shown in Fig. 8a. The annular beam appeared to be a bit elliptical, not a perfect ring as expected. The reason for this might be the thermal lens effect introduced by the different temperatures at the gas flow direction of the FTF CO2 laser. Next, the experiments on the proposed output coupling configuration with unstable resonator were carried out. The ICAS was fixed in the laser, while the OCR was placed on the manual stage. By adjusting the lateral and axial position, the output coupling beam was solid. In Fig. 8b, the experimental spot of the coupling output beam was measured at the corresponding transmission distance Z = 430 mm.
And the curve of the spot diameter of the output beam was measured, as shown in Fig. 9. After calculation, the far-field divergence of the output beam with this coupling configuration was 4.7 mrad (full-width). And the M 2pc factor was calculated to be 3.82, which means that there exists a significant improvement on beam quality by this coupling configuration compared with the original annular beam.
5 Summary
In this paper, we proposed a novel conical output coupler for the positive-branch confocal unstable resonator. This output coupling configuration consists of an inner cone annular scraper and an outer cone reflector. The OCR was placed just under the ICAS, and the axis line between the two mirrors was perpendicular to the optical axis of the resonator. The outer apex angle of the axis section in the ICAS and the inner apex angle of the axis section in the OCR were the same, in order to keep the two parts of the two mirrors to be parallel. Analysis of the two mirrors, which constitute the output coupling configuration, was given in geometrical optics.
The feasibility of this output coupling configuration with solid beam is examined numerically and experimentally with the help of a commercial FTF CO2 laser. With the eigenvector simulation method in the unstable resonator, the behavior of the annular output beam was investigated. The simulation results show that the annular beam of the conventional positive-branch confocal unstable resonator can be coupled to be a solid core beam. Under the 86.5 % power content, the numerical beam waists of the annular beam and solid core beam were 20.01 and 4.54 mm, respectively. In this case, the M 2pc factors were calculated to be 5.22 and 1.16 individually. This indicates an improvement of 4.5 times. With the application of a commercial FTF CO2 laser, experimental results showed that the coupling configuration could convert the annular beam into a solid one. The M 2pc factor of the original annular beam from this multi-transverse modes laser was more than 10. After installing the designed conical output coupler, the M 2pc factor of the improved beam was 3.82. This represents an improvement in beam quality. Meanwhile, this configuration has the advantage of easy implement. And this output configuration has potential to be applied in chemical oxygen iodine lasers and other lasers with unstable resonators.
References
A.E. Siegman, Appl. Opt. 13, 353 (1974)
M.E. Smithers, T.R. Ferguson, Appl. Opt. 23, 3718 (1984)
L.H. Min, K. Vogler, Opt. Commun. 74, 79 (1989)
G. Duplain, P.G. Verly, J.A. Dobrowolski, A. Waldorf, S. Bussiere, Appl. Opt. 32, 1145 (1993)
S. Makki, J. Leger, IEEE J. Quantum Electron. 37, 80 (2001)
A.H. Paxton, W.P. Latham Jr, Appl. Opt. 25, 2939 (1986)
Y. Jin, B. Yang, F. Sang, D. Zhou, L. Duo, Q. Zhuang, Appl. Opt. 38, 3249 (1999)
C. Pargmann, T. Hall, F. Duschek, K.M. Grünewald, J. Handke, Appl. Opt. 47, 6644 (2008)
C. Pargmann, T. Hall, F. Duschek, K.M. Grünewald, J. Handke, Appl. Opt. 51, 4219 (2012)
N. Hodgson, H. Weber, Laser Resonators and Beam Propagation (Springer, New York, 2005), pp. 653–654
D. Ehrlichmann, U. Habich, H. Plum, J. Phys. D Appl. Phys. 26, 183 (1993)
M. Endo, M. Sasaki, R. Koseki, J. Opt. Soc. Am. A 29, 507 (2012)
J.S.A. Collins, J. Opt. Soc. Am. 60, 1168 (1970)
A.G. Fox, T. Li, Bell Syst. Tech. J. 40, 453 (1961)
K.E. Oughstun, Prog. Opt. 24, 165–387 (1987)
C. Yuanying, W. Youqing, H. Jin, L. Jiarong, Opt. Commun. 234, 1 (2004)
D. Wang, Y. Qin, X. Tang, L. Xiao, Opt. Commun. 285, 2682 (2012)
The Language of Technical Computing. http://www.mathworks.com/
A.E. Siegman, in OE/LASE'90, 14–19 Jan., Los Angeles, CA (International Society for Optics and Photonics, 1990), pp. 2–14
H. Weber, Opt. Quantum Electron. 24, S861 (1992)
Y. Peng, L. Liu, V.S. Egorov, Z. Cheng, M. Zhang, Opt. Commun. 281, 705 (2008)
J.W. Goodman, Introduction to Fourier optics (Roberts and Company Publishers, Cincinnati, 2004), pp. 97–108
Acknowledgments
We acknowledge support by Educational Commission of Hubei Province of China (No. 2012FFB02207) and the Fundamental Research Funds for the Central Universities, HUST: No. CXY13Q012.
Author information
Authors and Affiliations
Corresponding author
Rights and permissions
About this article
Cite this article
Hu, A., Chen, P., Wang, Y. et al. Improvement of beam quality in unstable resonators by a conical output coupler. Appl. Phys. B 118, 335–341 (2015). https://doi.org/10.1007/s00340-014-5985-9
Received:
Accepted:
Published:
Issue Date:
DOI: https://doi.org/10.1007/s00340-014-5985-9