Abstract
The THz radiation emission of Au-coated nanogratings (fused silica substrate, 30 nm Au layer thickness, 500 nm grating constant) upon fs laser irradiation (785 nm, 150 fs, 1 kHz, ≤1 mJ/pulse) is observed in both directions along the laser beam axis (forward and backward) and for both, illumination of the Au/air or the Au/silica interface. THz radiation along the laser beam propagation is emitted in a narrow solid angle of about 15° fwhm independent on the laser pulse fluence, the angle of incidence and the nanograting profile. The bar width and groove depth of the nanograting as well as the angle of laser beam incidence strongly affect the THz radiation yield. The energy of single THz light pulses is measured absolutely (2 fJ in the 0.3–0.38 THz range) using a highly sensitive and fast superconducting transition edge sensor. The bi-directional emission of THz radiation is in agreement with the model assumption of surface plasmon polaritons propagating simultaneously on both Au layer interfaces (Au/air and Au/silica).
Similar content being viewed by others
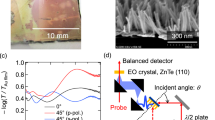
Avoid common mistakes on your manuscript.
1 Introduction
Electromagnetic radiation in the 0.1–50 Terahertz (THz) region (1 THz = 33 cm−1 = 4.1 meV) has attracted growing interest due to its large variety of demonstrated and potential applications (cf. e.g. [1, 2]). These applications range from biological [3], medical [4] and pharmaceutical sciences over semiconductor applications [5], security [6], information and communication technology [7], earth and space science [8] to basic research. The major units of a THz research system are the THz radiation source and the appropriate radiation detector. For both units detailed contributions are presented in the following paper.
THz radiation sources can be classified by their generation process. Conventional thermal incoherent sources like a blackbody at 2,000 K provide about 2 μW/cm2 at 1 THz per 10 GHz bandwidth. Direct coherent sources like free electron lasers (FEL) [9] or gas lasers can emit THz radiation with pulse energies up to 1 μJ, but are complex and expensive. Quantum cascade [10, 11] and p-germanium lasers [12], on the other hand, are compact and cost efficient but require cryogenic cooling. Quantum cascade lasers emit at fixed wavelengths and can achieve peak powers up to 20 mW. Lasers based on p-Germanium are tunable and yield an output power up to 10 W in the 1–4 THz region.
A very promising class of THz radiation sources, pushing the fast development of THz technology during the past two decades, relies on the use of optical energy sources. The underlying process may either be optical parametric generation, optical rectification [13], difference frequency generation or photomixing. Rectification in laser-induced plasmas is considered as a promising source of high-power THz radiation [14, 15]. An alternative approach uses electro-optic conversion in photoconductive switches under fs laser pulse irradiation [16, 13], an extremely powerful approach for THz spectroscopic applications. Recent photo-mixing technologies use cost effective diode lasers for the generation of continuous wave THz radiation which, however, achieved a THz emission power of about 100 pW only, by far too low for many applications [17]. Several research groups used fs laser irradiation of metal surfaces to generate THz radiation, most of them applying thin gold films typically deposited onto glass substrates [18–23].
The detection of THz radiation can be based on incoherent and coherent concepts. Common incoherent detectors are bolometers, golay cells and pyroelectric sensors. These are among the most sensitive THz sensors since they are free from the quantum noise of coherent detectors. However they do not provide information on the phase of the THz radiation. Coherent detectors are typically based on heterodyne or homodyne concepts [24]. THz emission setups using pulsed illumination sources are exceptional since they can use the inverse scheme of THz radiation generation for its phase sensitive detection. Electro-optical sampling of THz pulses by fs laser pulses uses laser beam splitting with one part for the generation and the other part for the detection of the THz emission. Since the generated THz pulse is roughly ten times longer than the fs laser pulse, it is possible to sample the temporal THz pulse shape by varying the time delay between both fs laser pulses. Subsequent Fourier transformation provides the frequency spectrum of the THz pulse. This method can, however, not provide the absolute energy of the THz radiation pulse.
The following paper describes both, (1) the generation of THz radiation on a Au-coated nanograting by fs laser irradiation and (2) the absolute energy measurement of single THz radiation pulses. This method of THz pulse generation was first described by Welsh et al. [19, 18] showing THz emission along the propagation direction of the fs laser beam upon irradiation of the grating’s Au/silica interface. Our recent rapid communication [22] showed new effects, THz emission in both directions along the laser beam axis (forward and backward to beam propagation, bi-directional emission) and for both cases, illuminating the Au/air or the Au/silica interface. These new findings are described in this paper in more detail, particularly investigating the angular distribution of the THz emission, the influence of the angle of incidence of the fs laser beam for various grating profiles (bar widths and groove depths) as well as measurements and finite element method simulations of the optical reflection, transmission, and absorption of fs laser light by the Au nanograting. The findings are discussed in view of the proposed THz generation mechanism [22] based on propagating surface plasmon polaritons (SPP) [25]. Furthermore, the energy of individual THz pulses was registered absolutely without further calibration using a superconducting transition edge sensor (TES).
2 Experimental setup
The experimental setup for the generation and detection of THz radiation is shown in Fig. 1. A regeneratively amplified Ti:sapphire laser (Coherent Mira 900 seed laser, Quantronix Titan amplifier) provides 150 fs pulses of ≤1 mJ energy centered around 785 nm wavelength at a repetition rate of 1 kHz. A λ/2 wave plate and a polarizing beam splitter are applied to regulate the average power of the linearly p- or s-polarized laser beam, which is collimated by a telescope to a beam of 5 mm diameter on the gold-coated grating. THz radiation is detected by a home-built calorimeter consisting of a superconducting TES and a filter set which transmits between 0.30 and 0.38 THz and blocks all other optical frequencies.
Experimental setup for the generation and detection of THz radiation using three different configurations: solid lines standard configuration of THz radiation detection in forward direction of fs laser beam propagation; broken lines optional configurations for detection of THz radiation a from the surface emitter (on the left) and b from the gold-coated grating emitted reversely to the laser beam (center of scheme); the setup configurations include front or rear side laser irradiation of the grating at a variable angle θ of laser beam incidence onto the gold-coated grating and variable angle φ for detection of THz radiation emission in the plane of laser irradiation; fs laser, λ/2 wave plate (WP), polarizing beam splitter (BS), optical chopper (OC), telescope (TC), adjustable mirrors, gold-coated grating, and calorimeter (TES)
The experiments can be performed in several laser irradiation and THz detection configurations (Fig. 1): laser irradiation of the rear (fused silica) or front (gold layer) surface of the gold-coated grating is possible at variable angle of incidence θ (\(\Updelta\theta = \pm 0.5^\circ\)) by rotating the substrate. The angular distribution (emission angle \(\varphi, \Updelta\varphi_0=\pm 0.5^\circ, \Updelta\varphi=\pm 0.25^\circ\)) of the THz radiation pulses propagating in laser beam direction is measured in the plane of incidence by rotating the TES calorimeter. THz radiation propagating in opposite direction of the laser beam can be reflected by an indium tin oxide (ITO) coated dichroic mirror (reflective to THz radiation but transparent at optical frequencies) onto the TES calorimeter in its optional alternative position. For comparison a semiconductor surface emitter (InAs, 5 mm × 10 mm area,1 mm thickness) can be used under θ = 45° fs laser irradiation. The TES then detects the emitted THz pulse in the direction of the reflected laser beam.
The gold-coated nanogratings (5 mm × 5 mm area) are fabricated in UV grade fused silica by reactive ion etching (grating constant 500 nm, bar widths between 240 and 320 nm, etching depths between 24 and 180 nm) and standard thermal deposition of a 30 nm thick Au layer. Their geometry is measured by an atomic force microscope (AFM, Park Scientific M5) in contact mode or a scanning electron microscope (SEM, Jeol JSM 6700F). The gold layer is examined using a focused ion beam, provided by a Carl Zeiss NTS Crossbeam Workstation NEON 60 and a Hitachi S4800II SEM (Fig. 2). The parameters of the gratings are listed in Table 1.
THz radiation is detected by a calorimeter using a superconducting TES [26] at 3 cm minimum distance from the Au nanograting. The TES absorbs the incident radiation in an appropriate antenna and transforms it into heat (Fig. 3). The heat is measured by a superconductor operated at its transition edge to normal conductivity with an effective resistance R TES. An applied bias voltage U BIAS induces intrinsic heating proportional to U 2BIAS /R TES and enables an electrothermal feedback [26]. Thus radiative heating of the detector is compensated by decreased intrinsic heating due to the increased temperature of the resistor R TES. This decrease of intrinsic heating is determined by a current measurement using a superconducting quantum interference device (SQUID), which acts as a cryo-cooled amplifier with an output voltage proportional to the TES signal [8]. This measurement technique enables an absolute power measurement of the absorbed radiation. In case of pulsed radiation the absolute energy of single pulses can be determined without additional calibration. The TES is shielded against optical frequencies by a filter set providing about 50 % transparency in the 0.30–0.38 THz frequency range.
Scheme of superconducting Transition Edge Sensor (TES) [8] (cf. text)
For gratings B1 and I5 fs laser transmission and specular reflection have been measured for different angles of incidence θ by conventional laser power meters with an accuracy of ±0.5 % for laser fluences of about 1.5 mJ/cm2. THz transmission has been measured on a BrukerOptics VERTEX 80v Fourier transform spectrometer.
3 Results
A typical TES signal is shown in Fig. 4 with \(T_{\rm{OC}}\) being the period of the optical chopper and \(T_{\rm{L}}=1\,{\rm{ms}}\) corresponding to the 1 kHz repetition rate of the fs laser. The time integral for an individual THz pulse (area A1 ≈ A2, cf. Sect. 4) is adequate to its energy and amounts to 0.68 fJ in this case.
Typical TES signal obtained with grating I4 (\(\theta_{\rm{rear}}=11,\) p-polarized 1.1 mJ/cm2): a \(T_{\rm{OC}}\), chopper period; b expanded detail showing resolved THz pulses (\(T_{\rm{L}}=1\,{\rm{ms}}\)) and area A1 ≈ A2 corresponding to absorbed THz pulse energy of 0.68 fJ, filled square measured signal, line simulated signal (cf. text)
Figure 5 illustrates the TES signal increase upon unblocking the p-polarized laser beam by the chopper blade within time \(\Updelta t_{\rm OC}={\rm 13\,ms}.\) The simulation includes a two-dimensional intensity distribution and an illumination area changing in time. The increase of the background is caused by the finite relaxation time of the TES. Hence, the THz pulse energy grows linearly with the laser pulse energy impinging onto the Au grating (cf. Sect. 4). The offset voltage \(\Updelta U\) evolves from the incomplete TES relaxation between the laser pulses (cf. Sect. 4).
TES signal onset (cf. Fig. 4a) showing increase of THz pulse energy while chopper unblocks p-polarized laser beam in \(\Updelta t_{\rm{OC}}\,=\,13\,{\rm{ms}}\) with THz pulse energy being proportional to the incident fs laser pulse energy. filled square measured signal, line simulated signal (cf. Sect. 4)
The THz pulse energy is determined as a function of the THz emission angle φ using the setup configuration in Fig. 1 at the optimum angle of laser beam incidence \(\theta_{\rm{max}}.\) The angular THz emission distribution obtained for grating I4 at three p-polarized laser pulse fluences is displayed in Fig. 6. Within the error limits the angular distribution in the plane of incidence is equal for the applied fluences.
Using p-polarized laser irradiation the angular distribution has been investigated for different grating geometries as well as for the two irradiation configurations (rear and front side, cf. Fig. 1). The optimum angle of incidence θ differs depending on the grating geometry and the irradiation configuration (as presented later in this paper). The peak of the angular THz emission distributions is observed in the direction of laser beam propagation φ = 0° for both gratings B1 and D1 (Fig. 7a).
Normalized THz pulse energy as a function of the THz emission angle φ (p-polarized laser irradiation): a grating D1 filled square rear (θ rear = 12°) and open circle front side (θ front = 30°) irradiated with \(0.76\,{\rm{mJ/cm^2}}, \Updelta\varphi\approx 15\) (fwhm); Inset: angular THz radiation distribution over measurement range \(\Updelta\varphi=\pm60\) obtained by rear side irradiation of grating D1. b Filled square grating B1 and open circle grating D1 both with rear side irradiation at θ rear = 11° with \(0.76\,{\rm{mJ/cm^2}}, \Updelta\varphi\approx 15 {\rm{(fwhm)}}; \times\) surface emitter \((2.55\,{\rm{mJ/cm^2}}) \Updelta\varphi\approx 10 {\rm{(fwhm)}}\)
Irradiation of either the rear or the front side of the Au nanograting yields a similar angular distribution of the THz radiation emission (Fig. 7b), although the optimum angle of incidence differs considerably (\(\theta_{\rm{rear}}=12^\circ, \theta_{\rm{front}}=30^\circ\)). In contrast, the THz radiation emitted from the semiconductor surface follows the reflected laser beam direction (cf. Figs. 1, 7b).
The THz pulse energy increases with the incident p-polarized laser pulse fluence and appears to saturate for high fluence values (Fig. 8). The THz pulse energy values strongly depend on the Au grating profile (Fig. 8). The measurements were performed at the optimal angle of incidence for each grating. Typically THz radiation measured for both conditions, laser irradiation of the rear and of the front side of the Au nanograting, provided a THz pulse energy ratio of about 1:0.75 (shown for grating B3 in Fig. 8) [22]. For comparison Fig. 8 displays the results obtained with the semiconductor surface emitter under otherwise identical conditions.
The generated THz pulse energies using gratings D1, D3 and D4 with different bar widths (225, 240 and 310 nm) but constant groove (etching) depth (180 nm) are depicted as a function of the incident p-polarized laser pulse fluence in Fig. 9, normalized to the related values of grating D1. The ratio of the THz pulse energies obtained for different bar widths is found to be independent of the laser pulse fluence.
THz pulse energy as a function of p-polarized laser pulse fluence for gratings D1, D3 and D4 (bar widths 310, 240, 230 nm; constant groove depth of 180 nm) all rear side irradiated at \(\theta_{\rm{rear}}=10^\circ,\) normalized to the values of D1 (310 nm, cf. Fig. 8)
Furthermore, the THz pulse energies recorded at a p-polarized laser pulse fluence of 1.25 mJ/cm2 for various gratings with bar widths ranging from 225–320 nm and depths from 70 to 180 nm are shown in Fig. 10. All these gratings originate from the same substrate wafer to ensure comparability (Fig. 10). The THz pulse energies are normalized to the maximum value of grating A1 (1.5 × 10−15 J) and increase with the bar width (Fig. 10a). This increase appears to be strongest for the smallest applied groove depth, being as high as one order of magnitude. The influence of the etching depth on the THz emission yield (Fig. 10b) shows a decrease with increasing depth for a large bar width (≈310 nm) and a slight increase with increasing depth for a small bar width (≈245 nm).
THz pulse energy obtained with p-polarized rear side irradiation at ≈1.25 mJ/cm2 laser fluence as a function of a the bar width for different grating depths b the depth for bar widths of ≈320 and 245 nm, angle of incidence \(\theta_{\rm{rear}}\) adjusted for maximum THz emission for each single grating; normalized to the maximum THz pulse energy of grating A1 (1.5 × 10−15 J in Fig. 8)
The generated THz pulse energy depends on the angle of laser beam incidence θ (Fig. 11a,b) as measured with the THz detector oriented in direction of the laser beam (ϕ = 0°; cf. Fig. 1). Optical power meters allow to evaluate the transmission (T 0) and specular reflection (R 0) of the p-polarized fs laser pulses. Representative results obtained for both, rear and front side irradiation of grating B1, are plotted in Fig. 11c, d. In addition, related simulations using a finite element method for the laser wavelength 785 nm are displayed in Fig. 11e, f.
Experiments with p-polarized fs laser irradiation of the Au/silica interface (left) and of the Au/air interface (right) of grating B1: THz pulse energy (parts a, b) as well as transmission T 0 and specular reflection R 0 of the p-polarized fs laser beam (parts c, d) as a function of the external angle of incidence θ; for comparison finite element method simulations of T, R and absorption A = 1 − T − R in parts e and f
THz radiation has neither been detected for irradiation of a discontinuously coated grating structure (cf. Fig. 2c) nor for irradition of a flat gold-coated substrate for any angle of laser beam incidence θ and THz emisson φ.
Finally the THz transmission spectra of a Au-coated nanograting and a 1 mm thick fused silica substrate (as used for the gratings) are displayed in Fig. 12. The Au-coated grating shows a very low transmission (<1 %) in the examined frequency range and the substrate a typical transmission behavior of a dielectric silica slab (\(n_{\rm{THz}({silica})}\,=\,1.95\)) allowing to determine its average transmission of \(\bar{T}\,=\,0.8\,\pm\,0.1\) between 300 and 380 GHz (average absorption coefficient \(\bar{\alpha} = (2.72\,\pm\,0.03)\,{\rm{cm}^{-1}}\)).
4 Discussion
One experimental novelty of our work ([22] and present paper) refers to the measurement of the THz pulse energy. Using the TES as a THz radiation detector allows to determine the THz pulse energy absolutely in the sensor defined spectral and spatial ranges without additional calibration.
Looking at Figs. 4 and 5, the slow response and relaxation of the TES evidently result in a background signal. The TES signal of the THz pulse train (Fig. 4b) can, however, be deconvoluted by (1) simulating the pulse train signal and obtain area A1 and (2) cutting the pulse train signal at the TES signal minima to yield area A2. The TES signal I(t) of a single THz pulse (pulse number 1 in Fig. 4b) is simulated by \(I(t)=I_{0}\left(1-{\rm e}^{\ -t/\tau_{1}}\right){\rm e}^{\ -t/\tau_{2}}\) with the signal amplitude I 0, the signal rise time τ1 and the signal decay time τ 2. The TES signal of a THz pulse train is the superposition of I(t) obtained from the individual pulses with a constant 1 ms delay between the pulses. The pulse train simulation in Fig. 4b is based on I 0 = 0.015 V, τ1 = 65 μs and τ2 = 520 μs. It nicely fits the experimental TES signal curve after the first two pulses which build up the TES background signal. The integral \(A1 = \int I(t) {\rm d}t = 0.00993\,{\rm {Vms}}\) of the first pulse represents the THz pulse energy and coincides with the experimentally determined value A2 = 0.01016 ± 0.00015 Vms within 2 %. A1 ≈ A2 ≈ 0.01 Vms correspond to 0.68 fJ per THz radiation pulse absorbed by the TES detector element.
An analogous procedure allows to simulate the TES signal increase upon unblocking the fs laser beam by the chopper blade (Fig. 5). In addition to the TES relaxational processes discussed above the spatial intensity profile of the fs laser beam has to be accounted for and is assumed to be Gaussian. At the beginning and the end of unblocking the laser beam the change of fs pulse energy impinging on the Au grating is minimal. The largest change occurs when the chopper blade edge passes across the laser beam axis (intensity maximum). On this basis the simulated curve nicely fits the experiment (Fig. 5) and yields a linear relation between the emitted THz and the absorbed fs laser pulse energies. The small discrepancies between simulation and experiment are attributed to laser beam deviations from a pure Gaussian profile and pulse to pulse fluctuations.
Taking into account the known attenuation of about 50 % by filters and antenna mismatch the incident THz pulse energy is estimated to 1.36 fJ. The total emitted THz pulse energy can be evaluated by the following way: the TES is sensitive to THz radiation in the 0.3–0.38 THz frequency range. The total spectrum of the emitted THz radiation is assumed to be very similar to that shown in the inset of Fig. 3 of ref. [18] obtained with a very similar Au nanograting under comparable fs laser illumination conditions. This spectrum ranges from zero to about 2 THz with its maximum around 0.7 THz. Numerical integration over the total spectrum \(I_{\rm{tot}}\) and over the filter’s bandwidth \(I_{\rm{filt}}\) yields a ratio of \(R_{\rm{spec}} = I_{\rm{tot}}/I_{\rm{filt}} \approx22.\) Furthermore, the above measurements revealed the angular distribution of THz emission to be concentrated along the laser beam axis (Fig. 7), irrespective of the angle of incidence and the fluence of the laser pulse. This angular distribution in the plane of incidence is assumed to be rotationally symmetric around the laser beam axis. Then one calculates approximately a ratio of \(R_{\rm{spat}} = E_{\rm{tot}}/E_{\rm{sense}}\,\approx\,3\) between the THz pulse energy \(E_{\rm{tot}}\) emitted into all forward directions (1/2 full solid angle) and that impinging onto the sensor \(E_{\rm{sense}}.\) Together with the known attenuation of about 50 % the total emitted THz pulse energy in forward direction of the laser beam can be estimated to 0.1 pJ corresponding to an average THz pulse power of 0.1 W for a pulse duration of 1 ps. The conversion efficiency of the incident fs laser pulse energy to the emitted THz pulse energy lies in the order of \(E_{\rm{THz}}/E_{\rm{Laser}}\,\approx\,10^-9\).
The most prominent new observations of our experimental studies ([22] and present paper) in comparison to the initial papers [19, 18] are (i) the bi-directional THz emission along and opposite to the exciting fs laser beam and (ii) the yield of THz radiation emission upon fs laser irradiation of either side of the Au-coated nanogratings (fused silica substrates) as briefly described in our rapid communication [22].
Prior to the discussion of the mechanism generating THz radiation it appears useful to first summarize the above described additional findings and the related conclusions: (iii) The THz pulse energy is proportional to the fs laser energy impinging onto the Au grating (Fig. 5). (iv) THz radiation observed on each side of the Au nanograting is selectively generated on the related Au layer surface, i.e. at the Au/air interface or at the Au/silica interface. Transmission of THz radiation through the Au grating layer is excluded (cf. Fig. 12). (v) The angular distribution of the THz emission has its maximum on the axis of the exciting fs laser beam and a fwhm value of \(\Updelta\varphi\approx15^\circ\) independent on the incident fs laser pulse fluence (Fig. 6) and independent on the direction of fs laser beam excitation (front or rear side irradiation at different angles of incidence, cf. Fig. 7). This fwhm value is larger than that obtained with the same setup when irradiating a semiconductor surface THz emitter \(\Updelta\varphi\approx10^\circ\) (fwhm) (Fig. 7). (vi) The THz pulse energy at first increases with the incident fs laser fluence but appears to saturate at high fluence values (Fig. 8). (vii) The dependence of the THz pulse energy on the incident fs laser fluence is the same for front and rear side illumination of the Au nanograting (Fig. 8). The lower THz pulse energy upon front side irradiation reflects the attenuation of THz radiation by about 15 % on passing through the fused silica substrate (Fig. 8, grating B3 and Fig. 12). (viii) The dependence of the THz pulse energy on the incident fs laser fluence differs by a constant factor only for Au nanogratings of different bar widths but otherwise identical dimensions like grating period and groove depth (Fig. 9). (ix) The maximum THz pulse energy depends strongly on the grating geometry (gratings A1, B1, and B3). Obviously, only a relatively small increase of the bar width (about 40 %) can yield an enhancement of the THz emission by a factor of 5. The relations between the THz pulse energy and bar width and groove depth appear to be complex (Fig. 10). (x) No THz radiation is observed upon fs laser irradiation of the Au stripe array of Fig. 2c or a smooth Au layer on a planar substrate. (xi) The THz pulse energy as a function of the angle of incidence of the fs laser in the above experiments is asymmetric with respect to the vertical irradiation. This is contrasted by the previous findings [19] as well as the experimental and the calculated angle dependences of the laser beam transmission and reflection (and absorption) showing a symmetric behaviour (Fig. 11). (xii) Absorption of fs laser radiation by the Au nanograting is a necessary but not a sufficient condition to generate THz radiation. Laser energy absorption can be quantified only by complete full solid angle detection of the reflected, diffracted and scattered laser light.
In general more than one mechanisms are possible for the generation of THz radiation by fs laser irradiation of thin Au layers. It depends on the experimental details like e.g. irradiation of flat Au layers with natural roughness [20, 21] or percolation [23] or of intentionally prepared Au nanogratings [18, 19, 22] or layer thicknesses ranging from a few nanometers [23] to several 100 nm [20, 21]. Furthermore, the various research groups applied different laser irradiation and THz detection geometries like e.g. THz detection in the direction of specular reflection [20, 21, 23] or in the original propagation direction of the exciting fs laser beam [18, 19, 22]. One special feature of the present study consists of the detailed angle variations, i.e. s- and p-polarization of the exciting laser beam as well as stepwise scanning of the angles of fs laser beam incidence θ (external angle) and of THz emission φ including φ = 180°.
All models of THz radiation generation are composed of two essential steps, the absorption of fs laser radiation by the Au nanograting and its transformation to THz radiation. The first step of fs laser energy input coupling by grating control must fulfill the requirements of k-vector matching (momentum conservation) and leads to propagating SPPs along the grating vector [18, 19, 27]. SPP excitation was studied in this work e.g. by checking the resonance angle of incidence where laser reflection is strongly reduced and absorption increased. In case of shallow gratings this resonance angle \(\theta_{\rm{SP}}\) can be derived using the well known phase matching condition \(\sin\theta_{\rm{SP}} + N \lambda/\Uplambda = \Re (n_{\rm{SP}}),\) where λ is the wavelength of the incident light and \(\Uplambda\) the grating period [28]. The complex refractive index relevant for the SPP is given by \(n_{\rm{SP}} = \sqrt{\epsilon_{\rm{d}}\epsilon_{\rm{m}}/ (\epsilon_{\rm{d}}+\epsilon_{\rm{m}})},\) where \(\epsilon_{\rm{d}}\) and \(\epsilon_{\rm{m}}\) are the permittivities of the dielectric and the metal of the interface, respectively. In case of front side irradiation (air \(\epsilon_{\rm{d}}\,=\,1;\) gold \(\epsilon_{\rm{m}}\,=\,-22\,+\,1.6i\) around 785 nm [28]) the resonance angle of incidence is calculated to \(\theta_{\rm{SP}}=33^\circ\) (Table 2). This value is verified by the measurements and simulations for the shallow grating I5 with 40 nm deep grooves in Fig. 13. The measured resonance profile is broader and less pronounced than the calculated curves. This is attributed to the imperfect Au grating (e.g. inhomogeneous gold layer; cf. Fig. 2b) and the broad emission spectrum of the fs laser pulse relative to the monochromatic light in the FEM simulation. It is pointed out that the FEM calculations include the effects of reflection, scattering etc. since the total transmitted and reflected parts of the incident radiation are calculated by evaluating the scatter parameters provided by the FEM simulation.
Gratings with deep grooves show a complex optical behavior: their resonance angles are less distinct than those of shallow gratings (cf. Fig. 11c–f). Taking into account the idealizations of the FEM model, the results of the simulation and measurement agree, however, sufficiently well in their main features. The formation of SPP cavity modes in grooves of 10 to 100 nm size in depth and width in the metal layer might be a possible explanation. The cavity modes induce reflection and transmission of the propagating SPP strongly dependent on the groove geometry [25].
The asymmetric angular dependence of the laser beam transmission and specular reflection by the Au nanogratings (Fig. 11) observed in this work differs from the symmetric behaviour reported previously [19], [18] and is attributed to the defects (voids) of the present Au grating layers (cf. e.g. Fig. 2b). This asymmetry demonstrates the high sensitivity of THz emission to the layer and grating structure, particularly its continuity. As extreme cases, no THz emission is observed upon fs laser irradiation of the Au stripe pattern depicted in Fig. 2c or of a flat Au layer or a very shallow Au nanograting of 24 nm groove depth and 260 nm bar width. Evidently the influence of the groove depth on THz emission cannot be interpreted independent from the bar widths of the grating (Fig. 10). Gratings with wide bars and smooth coatings appear to allow efficient THz emission.
Energy conservation requires optical absorption for THz emission. Comparing THz emission energy and optical absorption as a function of the angle of incidence, low absorption around 35 in Fig. 11d coincides with low THz emission (same angle in Fig. 11b). High optical absorption, on the other hand, without excitation of propagating SPPs, is not sufficient for effective THz emission (around +10 in Fig. 11b, d). Moreover, measurements of reduced specular reflection and transmission need not be related to increased absorption, but may indicate increased scattering and/or higher order diffraction of the laser beam at the gold grating [29–31]. In addition, the propagation of SPPs along the nanograting vector depend on the grating parameters due to reflection and confinement of the SPPs in the grooves [25, 31, 32] influencing both, fs laser absorption and frequency conversion. Therefore it is not surprising to find a complex dependence of the THz emission yield as a function of the groove depth with opposite trends for different bar widths (Fig. 10b).
Looking at statements (ii), (iv), and (vii) it is assumed that the generation of THz radiation follows essentially the same mechanisms on both Au layer surfaces, i.e. at the Au/air and Au/silica interfaces. Furthermore, a coupling between the processes at each Au layer surface is indicated by the maximum of THz radiation efficiency around the selected Au layer thickness of about 30 nm.
In the recently published model [19, 18, 27] SPPs are thought to enhance the local electric field and by this way promote electron emission out of the Au layer into the air. The acceleration of the emitted electrons is believed to provide the origin of the THz emission . This model is, however, not compatible with the above statements (i), (ii), (iv), and (vii), i.e. e.g. bi-directional THz emission from both Au layer surfaces following very similar mechanisms upon fs laser irradiation of either side of the Au nanograting: it is hard to believe that electron emission and the acceleration of the emitted electrons by fs laser irradiation is identical for laser irradiation of the Au/air and the Au/silica interfaces. This identity, however, is the basis for our interpretation of the findings in Fig. 7b and 8 for grating B3 [also cf. statement (vii)]. Therefore we propose a different model [22] to explain THz emission by use of SPPs propagating along the grating vector: a transient current perpendicular to the grooves of the grating is built up, which emits THz pulses directly (Fig. 14). In metal films of a thickness around the skin depth of the electric field a strong coupling of the SPPs in both interfaces is possible [33, 30], resulting in simultaneous THz emission from both sides of the grating. The lifetime of such SPPs can be estimated to several picoseconds using a two-temperature model for electrons and phonons [34]. Taking into account, that the electric field of a THz pulse generated by a time dependent current density j can be expressed in the far field as \({\rm{E}}_{\rm{THz}} \propto \partial j/\partial t\) [35], a SPP lifetime of several picoseconds corresponds well to the previously measured THz pulse length of 1ps [19].
Electric charges are assumed to move on the Au layer surfaces along the nanograting vector similar to the operation of photoconductive semiconductor devices but without the need of an external electric field (voltage supply by electrical contacts). The build-up time of the surface currents is determined by the exciting fs laser pulse duration. Their decay time is related to their characteristic propagation length finally limited by electron-phonon coupling. This model is sufficient to explain a large part of the above experimental findings. In particular it is consistent with (i) the bi-directional THz emission, (ii) the yield of THz radiation emission upon fs laser irradiation of either side of the Au-coated nanogratings, (iii) the proportionality of the THz pulse energy to the impinging fs laser pulse energy, (iv) the simultaneous generation of THz radiation on each side of the Au nanograting, (v) the angular distribution of the THz emission with its maximum on the axis of the exciting fs laser beam, and (vi) the THz pulse energy dependence on the incident fs laser fluence, being the same for front and rear side illumination of the Au nanograting, (vii) Furthermore, this model is consistent with the THz emission efficiency having a maximum around the applied Au layer thickness of about 30 nm. As a first approximation the dependence of the THz pulse energy on the fluence of the incident fs laser radiation (vi and vii) is considered to be semi-quantitatively the same as that of the photoconductive THz sources [1] or that achieved by lateral photo-Dember currents [36]. The directionality of the THz emission along the axis of the exciting fs laser beam in the far field can be explained by the positive interference of THz emission originating from the nanoscopic THz sources periodically arranged along the Au nanograting vector.
5 Conclusion
Following our previous short paper [22] new experimental findings of bi-directional THz emission upon NIR fs laser iradiation of Au-coated nanogratings were presented in detail and summarized in 12 statements [cf. (i)–(xii) in Sect. 4]. THz emission in both directions along the laser beam axis and its angular distribution were detected using a superconducting TES. Its operation in a calorimeter mode enabled the direct measurement of the absolute energy of the THz pulses without additional calibration.
The new experimental findings are found incompatible with the model previously presented for the interpretation of experimental results obtained with a very similar setup [19, 18, 27]. Our alternative approach assumes THz emission by the transient surface currents along the grating vector related to propagating SPPs in analogy to that by photoconductive THz sources [1] or lateral photo-Dember currents [36]. Further detailed experimental and theoretical work will be necessary to confirm or disprove this model.
Analyzing the yield of THz emission as a function of the various experimental parameters, a large potential for efficiency improvements is assumed particularly by optimizing the Au nanogratings (grating constant, bar width, groove depth, Au layer thickness, shape of grooves) and the operating parameters (fs laser wavelength, pulse duration, fluence, angle of incidence). In one case, the THz emission power obtained with a Au nanograting was even without optimization higher than that obtained with an equally sized InAs semiconductor surface emitter under equivalent experimental conditions (Fig. 8).
In comparison to the well-established semiconducting surface emitters it is pointed out that the presented Au nanogratings provide a bi-directional THz emitter (two coherent THz radiation pulses) with emission along the laser beam axis independent on the (optimized) angle of incidence, including front and rear side illumination. THz emission into the fs laser beam propagation direction provides very convenient sample irradiation conditions in the close neighborhood of the THz source. Furthermore, this THz radiation source can be operated remotely without any (electrical) contacts. These unique properties make further investigations and improvements highly rewarding.
References
M. Tonouchi, Cutting-edge terahertz technology. Nat. Photonics 1, 97–105 (2007)
C.A. Schuttenmaer, Exploring dynamics in the far-infrared with terahertz spectroscopy. Chem. Rev. 104, 1759–1779 (2004)
M. Brucherseifer, M. Nagel, P. Haring-Bolivar, H. Kurz, A. Bosserhoff, R. Büttner, Label-free probing of the binding state of DNA by time-domain terahertz sensing. Appl. Phys. Lett. 77, 4049–4051 (2000)
S. Hunsche, M. Koch, I. Brener, M.C. Nuss, THz near-field imaging. Opt. Commun. 150, 22–26 (1998)
E.J. Heilweil, J.E. Maslar, W.A. Kimes, N.D. Bassim, P.K. Schenck, Characterization of metal oxide nanofilm morphologies and composition by terahertz transmission spectroscopy. Opt. Lett. 34, 1360–1362 (2009)
T. May, S. Anders, V. Zakosarenko, M. Starkloff, H. G. Meyer, G. Thorwirth, E. Kreysa, A superconducting terahertz imager. In Proceedings of the SPIE, vol 6549, p. 65490D (2007).
T. Kleine-Ostmann, P. Dawson, K. Pierz, G. Hein, M. Koch, Room-temperature operation of an electrically driven terahertz modulator. Appl. Phys. Lett. 84, 3555 (2004)
T. May, V. Zakosarenko, R. Boucher, E. Kreysa, H.G. Meyer, Superconducting bolometer array with SQUID readout for submillimetre wavelength detection. Supercond. Sci. Technol. 16, 1430–1433 (2003)
B. Ferguson, X.C. Zhang, Materials for terahertz science and technology. Nat. Mater. 1, 26–33 (2002)
C. Sirtori, C. Gmachl, F. Capasso, J. Faist, D.L. Sivco, A.L. Hutchinson, A.Y. Cho, Long-wavelength (8–11.5 μm) semiconductor lasers with waveguides based on surface plasmons. Opt. Lett. 23, 1366–1368 (1998)
J. Alton, H.E. Beere, J. Fowler, E.H. Linfield, and D.A. Ritchie, Low-threshold superlattice quantum cascade laser emitting at λ = 103 μm and operating up to 70 k in continuous wave. In Proceedings of the SPIE, 5354, vol 5354, p.129 (2005).
E. Bründermann, D.R. Chamberlin, E.E. Haller, High duty cycle and continuous terahertz emission from germanium. Appl. Phys. Lett. 76, 2991–2993 (2000)
T. Löffler, T. Hahn, M. Thomson, F. Jacob, H. Roskos, Large-area electro-optic ZnTe terahertz emitters. Opt. Express 13, 5353–5362 (2005)
D.J. Cook, R.M. Hochstrasser, Intense terahertz pulses by four-wave rectification in air. Opt. Lett. 25, 1210–1212 (2000)
T. Bartel, P. Gaal, K. Reimann, M. Woerner, T. Elsaesser, Generation of single-cycle THz transients with high electric-field amplitudes. Opt. Lett. 30, 2805–2807 (2005)
D.H. Auston, K.P. Cheung, P.R. Smith, Picosecond photoconducting hertzian dipoles. Appl. Phys. Lett. 45, 284–286 (1984)
S. Hoffmann, M. Hofmann, E. Brundermann, M. Havenith, M. Matus, J.V. Moloney, A.S. Moskalenko, M. Kira, S.W. Koch, S. Saito, K. Sakai, Four-wave mixing and direct terahertz emission with two-color semiconductor lasers. Appl. Phys. Lett. 84, 3585–3587 (2004)
G.H. Welsh, K. Wynne, Generation of ultrafast terahertz radiation pulses on metallic nanostructured surfaces. Opt. Express 17, 2470–2480 (2009)
G.H. Welsh, N.T. Hunt, K. Wynne, Terahertz-pulse emission through laser excitation of surface plasmons in a metal grating. Phys. Rev. Lett. 98, 026803 (2007)
F. Kadlec, P. Kuzel, J.L. Coutaz, Optical rectification at metal surfaces. Opt. Lett. 29, 2674–2676 (2004)
F. Kadlec, P. Kuzel, J.L. Coutaz, Study of terahertz radiation generated by optical rectification on thin gold films. Opt. Lett. 30, 1402–1404 (2005)
F. Garwe, A. Schmidt, G. Zieger, T. May, K. Wynne, U. Hübner, M. Zeisberger, W. Paa, H. Stafast, H.G. Meyer, Bi-directional terahertz emission from gold coated nanogratings by excitation via femtosecond laser pulses. Appl. Phys. B 102, 551–554 (2011)
G. Ramakrishnan, P.C.M. Planken, Percolation-enhanced generation of terahertz pulses by optical rectification on ultrathin gold films. Opt. Lett. 36, 2572–2574 (2011)
P.Y. Han, X.C. Zhang, Free-space coherent broadband terahertz time-domain spectroscopy. Meas. Sci. Technol. 12, 1747–1756 (2001)
M. Kuttge, F.J. Garcíade Abajo, A. Polman, How grooves reflect and confine surface plasmon polaritons. Opt. Express 17, 10385–10392 (2009)
K.D. Irwin, An application of electrothermal feedback for high resolution cryogenic particle detection. Appl. Phys. Lett. 66, 1998–2000 (1995)
Y. Gao, M.K. Chen, C.E. Yang, Y.C. Chang, S. Yin, R. Hui, P. Ruffin, C. Brantley, E. Edwards, C. Luo, Analysis of terahertz generation via nanostructure enhanced plasmonic excitations. J. Appl. Phys. 106, 074302 (2009)
H. Raether, Surface plasmons on smooth and rough surfaces and on gratings, vol 111 of Springer Tracts in modern physics (Springer, Berlin, 1988)
J. Moreland, A. Adams, P.K. Hansma, Diffraction efficiency and electron microscopy of large amplitude holographic gratings. Opt. Commun. 45, 11–16 (1983)
I.R. Hooper, J.R. Sambles, Coupled surface plasmon polaritons on thin metal slabs corrugated on both surfaces. Phys. Rev. B 70, 045421 (2004)
E. Popov, N. Bonod, S. Enoch, Comparison of plasma surface waves on shallow and deep metallic 1d and 2d gratings. Opt. Express 15, 4224–4237 (2007)
J. Le Perchec, P. Quemerais, A. Barbara, T. Lopez-Rios, Why metallic surfaces with grooves a few nanometers deep and wide may strongly absorb visible light. Phys. Rev. Lett. 100, 066408 (2008)
E.N. Economou, Surface plasmons in thin films. Phys. Rev. 182, 539–553 (1969)
F. Garwe, U. Bauerschäfer, A. Csaki, A. Steinbrück, K. Ritter, A. Bochmann, J. Bergmann, A. Weise, D. Akimov, G. Maubach, K. König, G. Hüttmann, W. Paa, J. Popp, W. Fritzsche, Optically controlled thermal management on the nanometer length scale. Nanotechnology 19, 055207 (2008)
K. Sakai, (ed.), Terahertz optoelectronics, vol 97. In Topics in applied physics (Springer, Berlin, 2005).
G. Klatt, F. Hilser, W. Qiao, M. Beck, R. Gebs, A. Bartels, K. Huska, U. Lemmer, G. Bastain, M.B. Johnston, M. Fischer, J. Faist, T. Dekorsy, Terahertz emission from lateral photo-dember currents. Opt. Express 18, 4939–4947 (2010)
Acknowledgments
We thank Dr. J. Bergmann, M. Schubert, A. Reinhard and C. Schmidt from the Institute of Photonic Technology, Jena, Germany, for helpful discussions and technical support, respectively. We are grateful for the sample characterization conducted by D. Schelle from the University of Jena, Germany, using their focused ion beam facility and scanning electron microscope. The authors thank K. Wynne, University of Strathclyde, UK, for our fruitful cooperation. The authors acknowledge support by the Thuringian ministry of education, science and culture (TeLIGHT, B714-09059).
Author information
Authors and Affiliations
Corresponding author
Rights and permissions
About this article
Cite this article
Schmidt, A., Garwe, F., Hübner, U. et al. Experimental characterization of bi-directional terahertz emission from gold-coated nanogratings. Appl. Phys. B 109, 631–642 (2012). https://doi.org/10.1007/s00340-012-5230-3
Received:
Revised:
Published:
Issue Date:
DOI: https://doi.org/10.1007/s00340-012-5230-3