Abstract
While screening for genes affected by cold, we found that Early Responsive to Dehydration 10 (ERD10) was induced by cold treatment. To further investigate the physiological functions of ERD10, we analyzed the T-DNA insertion mutant of ERD10 as well as the expression of ERD10 in response to various stress conditions. The erd10 mutant showed reduced stress tolerance relative to wild-type plants. Activation of the CBF/DREB1 genes by cold stress did not occur in the erd10 mutant, indicating that an increased level of ERD10 is required to subsequently activate the CBF/DREB1 genes and their downstream target genes during treatment with cold stress in Arabidopsis. In addition, we showed that accumulation of the ERD10 transcript in developing seeds was necessary for completion of seed development. Erd10 mutant seeds were abnormally shaped and showed reduced germination. These results suggest that ERD10 can play roles both in protection of the plants from various stresses, including cold and dehydration, and also in seed development and germination.
Similar content being viewed by others
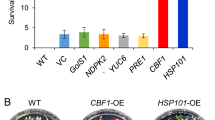
Avoid common mistakes on your manuscript.
Introduction
During their life span, plants are exposed to a variety of environmental changes, some favorable and others harmful. Plants adopt several intricate strategies to overcome the environmental changes that threaten their growth and development. First, plants allocate more metabolic energy for defending themselves, resulting in reduced growth (Zhu 2002). The cell walls are reinforced and the concentrations of several protective solutes in the cytoplasm increase (Huang et al. 2008; Chinnusamy et al. 2007). These processes occur through the actions of specific genes, known as stress-responsible genes. Recently, through comprehensive microarray analyses combined with bioinformatics, there have been numerous reports of enhanced or reduced stress-responsive genes with different kinetics in response to specific stresses (Zhu 2002; Shinozaki et al. 2003; Fowler and Thomashow 2002). Not all stress-inducible genes respond to each specific stress, such as cold, dehydration, heat, high soil salinity and nutrient starvation. For example, only 10% of cold-inducible genes overlapped with genes induced by dehydration or high salt (Yamaguchi-Shinozaki and Shinozaki 2005), indicating that different subsets of genes respond to different environmental stresses. However, little is known about the physiological function of stress-responsive genes. The fluctuating expression levels of these genes may just be symptomatic of metabolic changes caused by stress, or perhaps the proteins encoded by these genes perform various roles, from transcriptional activation of downstream genes to being final effector molecules, so the plant can survive under harsh conditions.
The genes of the ERD family were identified collectively as genes that are rapidly induced by dehydration (Kiyosue et al. 1994a). Since then, some of the specific ERD genes have been further studied. ERD1 encodes an ATP-dependent chloroplast protease (Soitamo et al. 2008). A mitochondrial proline dehydrogenase (ProDH) and a sugar transporter are encoded by ERD5 and ERD6, respectively (Nakashima et al. 1998; Kiyosue et al. 1998). In addition, the proteins encoded by ERD9 and ERD13 belong to the glutathione-S-transferase (GST) family (Kiyosue et al. 1993). We previously reported that ERD13, AtGSTF10, a GST in the plant-specific phi class, is a BAK1-interacting protein. BAK1 is a co-receptor that forms the brassinosteroid (BR) receptor complex with BRI1, mediating BR signaling in Arabidopsis. Overexpression of AtGSTF10 resulted in plants with higher salt tolerance. In contrast, the down-regulation of AtGSTF10 produced by RNA interference caused reduced tolerance to abiotic stress and the accelerated senescence of transformants (Ryu et al. 2009). These findings suggest that modulation of ERD13/AtGSTF10 is likely to alter the stress responsiveness of the plants, in which BR signaling may be involved through the interaction of BAK1 with AtGSTF10.
ERD10 and ERD14 have been reported to have chaperone activity that might help protect the proteins in response to stress (Kovacs et al. 2008). ERD12 encodes a protein similar to allene oxide cyclase (Gutiérrez et al. 2004). These results imply that although all of the ERD genes are inducible by dehydration, each gene may be involved in various functional processes, which might be necessary to coordinate the metabolic changes caused by dehydration. Other than the examples mentioned herein, few reports of specific functions for most of the ERD genes are available.
Here, we provided in vivo evidence that ERD10 can play roles both in the protection of plants against various stresses, including cold and dehydration, and also in seed development and germination.
Materials and methods
Plant growth
Arabidopsis thaliana Columbia (Col-0) was used as the wild-type line in all experiments. BRI1-GFP transgenic and bri1-9 mutant plants are in the same ecotype background. Mutant seeds for ERD10 (SALK_087789) were obtained from the Arabidopsis Stock Center. Sterilization of seeds and growth conditions were as previously described (Ryu et al. 2009).
Stress treatments and assessment
In all cases, 10-day-old seedlings grown on 1/2 MS plates were used for the stress treatments, excluding only drought treatment. For cold treatment, seedlings were incubated for 24 h in a 4°C-growth chamber under the long-day condition (16 h light/8 h dark). To isolate RNA, tissues were collected immediately after cold treatment and then frozen in liquid nitrogen. To assess the phenotypic changes caused by cold, the plates were moved back to 22°C. After 2 days, we counted the proportion of damaged leaves out of the total number of leaves. For dehydration treatment, 10-day-old seedlings grown on 1/2 MS plates were placed onto Whatman 3MM filter paper in the laminar hood for the indicated time. For the UV treatment, seedlings were exposed to UV light at 20 J/s for 4 h as described by Ryu et al. (2009). For drought treatment, we used 3-week-old soil-grown plants and did not irrigate them for 12 days before re-watering them. After 2 days resumption of growth was observed.
Determination of germination
Wild-type and erd10 mutant seeds sown on 1/2 MS were cold-stratified at 4°C for 2 days in the dark and then incubated at 22°C under the long-day condition for 5 days. Each day germinated seeds with protruding radicles were counted (Oh et al. 2006).
Expression analysis by RT-PCR and northern blot
Total RNA was isolated from 10-day-old seedlings grown on 1/2 MS or from various tissues of 4-week-old soil-grown plants. For northern hybridization, 10 μg of total RNA was separated in a 1% formaldehyde agarose gel, transferred to a Hybond-N+ nylon membrane (GE Healthcare Life Sciences), and hybridized with ERD10 cDNA in a solution of 50% formamide (v/v), 10% SDS (w/v), 1 M NaCl and 10% dextran sulfate (w/v) at 42°C. For RT-PCR analysis, first-strand cDNA synthesis was performed with RNase-free RQ1 DNases (Promega)-treated RNA using the SuperscriptIII-MMLV reverse transcriptase (Invitrogen) and oligo d(T15) as a primer. The same amount of first-strand cDNA was used as a template in the second step of the polymerase chain reaction for 26 cycles with ERD10-specific primers (forward 5′-gcagaagagtacaagaacaccgt-3′, reverse 5′-ctccagtggtcttggcgtgataac-3′). The primer sequences of CBF1, CBF2, CBF3, Cor15a, and Cor47 have been described in a previous paper (Kim et al. 2009). The expression of the tubulin gene (forward 5′-atgcgtgagattcttcacatcc-3′, reverse 5′-tgggtactcttcacggatcttag-3′) was used to normalize the data.
Results
ERD10 was selected as a gene that is highly induced by cold
In our previous studies to test stress responses of BR-related mutants and transgenic plants, we observed that the bri1-9 mutant showed a much higher tolerance to cold than the wild-type or BRI1-overexpressor (Kim et al. 2009). To examine which genes might respond differently to cold in wild-type versus the bri1-9 mutant, we performed microarray analysis with RNA from wild-type and bri1-9 plants treated with cold for 24 h and from untreated corresponding plants. Of the 22,810 probe sets of the Affymetrix array, cold treatment resulted in 1,297 genes up-regulated and 1,611 genes down-regulated at least twofold in wild type compared to the non-stressed plant, while only 507 and 768 genes were up-, and down-regulated, respectively, in bri1-9 under the same treatment. We will provide detailed microarray results in a future publication.
Since we revealed that ERD13 is involved in the stress response connected with BR signaling (Ryu et al. 2009), we attempted to elucidate the physiological function of each member of the ERD family. Therefore, we extracted all microarray data, regardless of whether they met the twofold cutoff criterion, for the expression of each member of the ERD family in response to cold treatment in the wild type and bri1-9 plants (Fig. 1). ERD4, ERD7, ERD10, and ERD14 showed a highly inducible pattern in cold-treated wild-type plants, while the expression of ERD13 and ERD15 was repressed by cold. The fluctuation of ERD gene expression in response to cold in bri1-9 plants was not as dramatic as that in wild-type plants. We were more interested in ERD10 because it was the ERD gene that was most induced by cold in this analysis (Fig. 1a). We confirmed by northern blot analysis that ERD10 was greatly induced by cold treatment (Fig. 1b).
Expression of the ERD gene family in response to cold treatment. Microarray analysis was performed in duplicate using the Affymetrix GeneChip® Arabidopsis ATH1 Genome Array X 4 with the total RNAs from wild-type and bri1-9 plants treated with or without cold for 24 h. The image was acquired using an Affymetrix GeneChip® Scanner 3000 7G, and the data were extracted and normalized with Affymetrix GCOS software and the RMA (Robust Multi-array Average) Normalization method (DNA Link Inc., Seoul, Korea). a Endogenous expression of 14 ERD genes in wild-type and bri1-9 plants with and without cold treatment are shown in the upper panel, and the expression levels of each ERD gene from all four samples are shown as a relative accumulative graph in the lower panel. b ERD10 was highly inducible by cold in wild-type and bri1-9 plants, as confirmed by northern blot analysis
Lack of ERD10 resulted in reduced tolerance to abiotic stresses
No information on stable transformants overexpressing ERD10 is available. All reports about ERD10 were based on either recombinant proteins generated in E. coli for biochemical studies (Kovacs et al. 2008) or on transient expression in tobacco leaf tissues (Abu-Abied et al. 2006). Therefore, we attempted to generate transgenic plants expressing ERD10 under the native promoter or the 35S CaMV constitutive promoter. However, we repeatedly were unable to select transformed plants whose growth went beyond cotyledon formation to the development of the true first leaf. This result suggests that overexpression of ERD10 may be lethal to the postembryonic development of Arabidopsis. In an alternative approach to detect the function of ERD10, we analyzed the T-DNA insertion knock-out line for ERD10. The T-DNA insertion site was 300 base pairs upstream from the annotated first methionine codon of the ERD10 gene. We confirmed the lack of transcript message of ERD10 from this knock-out line by northern blot analysis (Fig. 2a).
The erd10 knock-out mutant showed a reduced tolerance to low temperature. a Schematic feature of the ERD10 gene with the T-DNA insertional site. No transcriptional message was detected in the erd10 mutants due to the T-DNA insertion in the 5′UTR. b Seedling phenotypes of erd10 mutant compared to wild-type treated with/without cold for 24 h were observed after 48 h at normal temperature. Typical cold-damaged leaves are magnified in the inset. The outer shape of cold-damaged leaves for both wild-type plants and erd10 mutants were similar. Scale bars denote 2 mm. c Cold responsiveness was quantitatively determined in the erd10 mutant by the proportion of damaged leaves after cold treatment. Experiments were repeated three times, and more than 50 seedlings per line were tested in each experiment. Bars denote the standard deviation. d The expression levels of the CBF1, CBF2, CBF3, Cor15a, and Cor47 genes in response to cold were determined in erd10 mutants and wild-type plants by semi-quantitative RT-PCR analysis. Total RNA was immediately prepared from the seedlings treated with/without cold treatment for 24 h and amplified with gene-specific primers
Although we did not observe any noticeable phenotypic changes of erd10 mutant plant under normal growth conditions, the erd10 mutant showed reduced stress tolerance relative to wild-type plants. We treated the erd10 mutant and wild-type plants with cold by placing them at 4°C for 24 h. After 2 days of recovery time under normal conditions, we compared their phenotypic changes. Cold-damaged leaves are typically dry, fragile, bleached and compactly curl (Fig. 2b). We counted the number of leaves in which more than 50% of the leaf blade area was damaged, from each cold-treated sample. As shown in Fig. 2c, about 40% of the wild-type leaves were damaged by cold treatment. In comparison, 70% of the erd10 mutant leaves showed damage, a much higher rate than that observed for wild-type plants. This result immediately led us to check the expression of several characterized cold stress-inducible genes upon cold in erd10 mutant and wild-type plants. We performed semi-quantitative RT-PCR analysis for the expression levels of CBF1/DREB1B, CBF2/DREB1C, and CBF3/DREB1A, well-known transcription factors that are critical for activation of the expression of COR genes, which are involved in cold acclimation (Chinnusamy et al. 2007). As expected, activation of the CBF/DREB1 genes by cold stress was remarkable in wild-type plants, while basal expressions levels of these genes were barely detectable in the absence of cold. In contrast, cold inducibility of any of these genes was not distinguishable in the erd10 mutant. The expression levels of Cor15a and Cor47 were not highly induced in erd10 compared to wild-type plants in response to cold treatment (Fig. 2d). These results, combined with the result in Fig. 1c showing that the expression of ERD10 dramatically increased by cold treatment, imply that ERD10 is required to activate the CBF/DREB1 genes and their downstream target genes in response to cold stress in Arabidopsis.
In addition, the erd10 mutant showed reduced tolerance to drought stress relative to wild-type plants. While about 10% of wild-type plants dehydrated for 12 days turned green again after re-watering, erd10 mutants barely recovered from the same stress. Both BRI1-GFP and bri1-9 plants demonstrated a higher survival capacity than wild-type plants in response to the same stress (Fig. 3a). ERD10 expression increased continuously in response to dehydration (Fig. 3b); however, exposure to UV did not affect the expression of ERD10 (Fig. 3c). These results suggest that the accumulation of ERD10 is involved in the tolerance to subsets of abiotic stresses in Arabidopsis, and the lack of ERD10 leads to greater plant susceptibility to these stresses.
Erd10 mutants showed a decreased tolerance to dehydration. a Phenotypes of erd10 mutants were compared to the bri1-9 mutants, BRI1-GFP transgenic plants, and wild-type plants after dehydration for 12 days as described in “Materials and methods”. Similar results were obtained from experiments performed twice with different batches of plants. The responses of ERD10 expression to dehydration (b) and to UV treatment (c) in wild-type plant were analyzed by RT-PCR analysis
Seed germination was delayed in the erd10 mutant
As mentioned before, after germination, the erd10 mutant showed no defective growth patterns under normal conditions. However, seed germination itself was affected by the lack of ERD10. The germination rate of the erd10 mutant over 5 days after imbibition was lower than that of wild-type plant (Fig. 4a). More than 80% of wild-type seeds germinated within 2 days. However, only 25 and 45% of erd10 seeds had germinated at 2 and 3 days after imbibitions, respectively.
Seed germination was delayed in the erd10 mutant. a Delayed germination was determined over 5 days after imbibition in erd10 mutant seeds compared to wild-type seeds. Experiments were repeated three times, and more than 100 seeds per line were tested in each experiment. Bars denote the standard deviation. b The accumulation of ERD10 transcripts was determined in developing seeds by RT-PCR analysis. Total RNA was prepared from the green seeds (G), yellow seeds (Y), fully dried seeds (D), and germinating seeds (GS) of wild-type plants. c The different appearance of erd10 mutant seeds compared to wild-type seeds. The region marked by the arrow heads in each seeds indicates the groove that is formed between the two folded-cotyledons and radicle
ERD10 can be classed with group II LEA proteins based on its amino acid sequence homology to Cor47, a representative late embryogenesis-abundant (LEA) protein. Plant-specific Group II LEA proteins, also known as dehydrins, are expressed in late stages of seed maturation (Wise and Tunnacliffe 2004). Therefore, we hypothesized that a lack of accumulation of ERD10 in late stages of seed maturation resulted in the reduction of germination of erd10 mutant seeds. To investigate this possibility, we further analyzed the expression of ERD10 by dissecting the stages of seed maturation in wild-type plants (Fig. 4b): green seeds (G), yellow seeds (Y), dried-brown seeds (D), and germinating seeds (GS). We found that transcript levels of ERD10 gradually accumulated during the seed maturation from incipient green seeds to mature dried-brown seeds. Interestingly, transcript levels of ERD10 decreased after germination. Because we observed that the ERD10 gene was expressed during seed development but its expression level dropped sharply after germination (Fig. 4b), we investigated whether the lack of ERD10 affects seed development in the erd10 mutant. Compared to wild-type seeds, erd10 seeds are a little smaller, and the groove that is formed between the two folded-cotyledons and the radicle is not distinct in erd10 seeds (Fig. 4c). These results suggest that the accumulation of ERD10 over time is required for seed maturation and germination in Arabidopsis.
Discussion
The multigene ERD family contains 27 individual cDNAs that display induced expression after 1 h of dehydration (Kiyosue et al. 1994a). Different subsets of ERD family members have been shown to be up-regulated or down-regulated by various other environmental stimuli, such as cold (Yamaguchi-Shinozaki and Shinozaki 2005), light (Soitamo et al. 2008), excessive arsenate (Abercrombie et al. 2008) or a transient increase in cytoplasmic Ca2+ (Kaplan et al. 2006). However, despite their responsiveness to various stresses, the mechanism by which ERD proteins exert their functional roles in plants has been unclear. Here, we provide experimental evidence that ERD10 is likely to be essential for cellular protection from abiotic stresses, especially dehydration and cold (Figs. 2, 3) and further to be involved in seed development (Fig. 4).
The expression of ERD10 was increased eightfold in wild-type plants in response to cold treatment (Fig. 1). The erd10 mutant was less tolerant to a 24-h cold treatment than wild-type plants. We found that the critical transcription factors CBF/DREB1 were not activated in response to cold in erd10 mutant. This result suggests that ERD10 is necessary for the accumulation of cold-inducible transcription factors and their downstream targets including Cor15a and Cor47. However, it is not clear how ERD10 is involved in this process. There are two possibilities to explain how ERD10 functions in plant cells. ERD10, along with ERD14, is known to be a protein that shows severe bias in its amino acid composition (Kiyosue et al. 1994b). This feature results in an intrinsically disordered conformation of ERD10 protein (Mouillon et al. 2006). Therefore, beyond the threshold amount of ERD10 protein itself resulting from the highly increased level of ERD10 by the certain stress can be a non-specific cellular signal to trigger the activation of stress-specific inducible genes. Another possibility is that ERD10 protects the transcripts of a subset of cold-inducible genes, rather than directly activating gene expression. In previous reports, intrinsically disordered proteins (IDPs) have been shown to have diverse functions from a high ion-binding capacity (Alsheikh et al. 2005) to stabilizing membrane structure (Koag et al. 2003), and exerting chaperone activity on both RNA and proteins (Tompa and Csermely 2004). Recently, recombinant ERD10 proteins induced in E. coli were revealed to be more resistant to a protease than the globular bovine serum albumin (BSA), which is one of the characteristics of IDPs. Recombinant ERD10 also prevented the heat-induced aggregation of various substrates and bound to acidic phospholipid vesicles in vitro (Kovacs et al. 2008). However, none of these functions has been confirmed in intact plant systems. In another line of research, ERD10 was identified as a cytoskeleton-interacting protein by its localization to actin stress fibers protecting the cytoskeleton from latrunculin-mediated disruption (Abu-Abied et al. 2006). These results, together with our data, strongly suggest that ERD10 has both chaperone activity and the capacity to stabilize membrane structures. These cognate protective roles of ERD10 as a versatile chaperone can be manifested not only under stress conditions but also during seed maturation. The mature seeds of Arabidopsis are surrounded by two layers: a thin inner endosperm layers and an outer testa layer, called the seed coat (Vaughan et al. 1971; Nguyen et al. 2000). Seed germination begins with the uptake of water by dehydrated mature seeds and finishes when the radical has emerged by two successive ruptures of the endosperm and testa layers (Koorneef et al. 2002; Müller et al. 2006). Although in our current experiment we did not differentiate these two sequential processes, we showed that the germination rate of erd10 mutant seeds based on radical protrusion declined dramatically compared to wild-type seeds (Fig. 4a).
Moreover, we noticed that when we plated the transgenic seeds of the T1 generation, very few seeds had not germinated at the time of cotyledon emergence (only 23 seeds had not germinated out of 4,057 transgenic seeds). We could not analyze the kinetics of the germination of ERD10-overexpressing transgenic plants as we did with the erd10 mutants, because we could not obtain mature transformants from the transgenic plants expressing ERD10 under either the native promoter or the 35S CaMV constitutive promoter, as described in the “Results” section. Most of the ERD10-overexpressing transgenic plants died shortly after cotyledon formation. It remains to be confirmed, via transformation of the DNA construct leading to the overexpression of ERD10 into the erd10 mutants, whether or not the reduced germination rate of the erd10 mutant can be rescued. However, based on the current observations, inducible promoter-driven overexpression of ERD10 in the wild-type as well as the erd10 mutant background may be a possible approach to elucidate the functions of ERD10 in germination and in seed maturation. It has been reported that ERD10 is not expressed in dry seeds (Kiyosue et al. 1994b). However, we detected the ERD10 transcript in dry seeds. Moreover, we showed that the accumulation of ERD10 gradually increased from green seeds to fully mature seeds. This expression pattern is more plausible for explaining the protective roles of ERD10 in seed maturation, which particularly requires the desiccation step after the synthesis of the seed reservoir, just before germination.
Abbreviations
- ERD:
-
Early Responsive to Dehydration
- CBF/DREBs:
-
C-repeat binding factor/dehydration-responsive element binding
- COR:
-
Cold-related
- LEA:
-
Late embryogenesis-abundant
References
Abercrombie JM, Halfhill MD, Ranjan P, Rao MR, Saxton AM, Yuan JS, Stewart CN (2008) Transcriptional responses of Arabidopsis thaliana plants to As (V) stress. BMC Plant Biol 8:87. doi:10.1186/1471-2229-8-87
Abu-Abied M, Golomb L, Belausiv E, Huang S, Geiger B, Kam Z, Staiger CJ, Sadot E (2006) Identification of plant cytoskeleton-interacting proteins by screening for actin stress fiber association in mammalian fibroblasts. Plant J 48:367–379
Alsheikh MK, Svensson TJ, Randall SK (2005) Phosphorylation regulated ion-binding is a property shared by the acidic subclass dehydrins. Plant Cell Environ 28:1114–1122
Chinnusamy V, Zhu J, Zhu JK (2007) Cold stress regulation of gene expression in plants. Trends Plant Sci 12:444–451
Fowler S, Thomashow MF (2002) Arabidopsis transcriptome profiling indicates that multiple regulatory pathways are activated during cold acclimation in addition to the CBF cold response pathway. Plant Cell 14:1675–1690
Gutiérrez RA, Green PJ, Keegstra K, Ohlrogge JB (2004) Phylogenetic profiling of the Arabidopsis thaliana proteome: what proteins distinguish plants from other organisms? Genome Biol 5(8):R53. doi:10.1186/gb-2004-5-8-r53
Huang D, Wu W, Abrams SR, Cutler AJ (2008) The relationship of drought-related gene expression in Arabidopsis thaliana to hormonal and environmental factors. J Exp Bot 59:2991–3007
Kaplan B, Davydov O, Knight H, Galon Y, Knight MR, Fluhr R, Fromm H (2006) Rapid transcriptome changes induced by cytosolic Ca2+ transients reveal ABRE-related sequences as Ca2+-responsive cis elements in Arabidopsis. Plant Cell 18:2733–2748
Kim SY, Kim BH, Lim CJ, Lim CO, Nam HK (2009) Constitutive activation of stress-inducible genes in a bri1 (brassinosteroid-insensitive 1) mutant results in higher tolerance to cold. Physiol Plant. doi:10.1111/j.1399-3054.2009.01304.x
Kiyosue T, Yamaguchi-Shinozaki K, Shinozaki K (1993) Characterization of two cDNAs (ERD11 and ERD13) for dehydration-inducible genes that encode putative glutathione S-transferases in Arabidopsis thaliana L. FEBS Lett 335:189–192
Kiyosue T, Yamaguchi-Shinozaki K, Shinozaki K (1994a) Cloning of cDNAs for genes that are early-responsive to dehydration stress (ERDs) in Arabidopsis thaliana L.: identification of three ERDs as HSP cognate genes. Plant Mol Biol 25:791–798
Kiyosue T, Yamaguchi-Shinozaki K, Shinozaki K (1994b) Characterization of two cDNAs (ERD10 and ERD14) corresponding to genes that respond rapidly to dehydration stress in Arabidopsis thaliana. Plant Cell Physiol 35:225–231
Kiyosue T, Abe H, Yamaguchi-Shinozaki K, Shinozaki K (1998) ERD6, a cDNA clone for an early dehydration-induced gene of Arabidopsis, encodes a putative sugar transporter. Biochim Biophys Acta 1370:187–191
Koag MC, Fenton DR, Wilkens S, Close TJ (2003) The binding of maize DHN1 to lipid vesicles: gain of structure and lipid specificity. Plant Physiol 131:309–316
Koorneef M, Bentsink L, Hilhorst H (2002) Seed dormancy and germination. Curr Opin Plant Biol 5:33–36
Kovacs D, Kalmar E, Torok Z, Tompa P (2008) Chaperon activity of ERD10 and ERD14, two disordered stress-related plant proteins. Plant Physiol 147:381–390
Mouillon JM, Gustafsson P, Harryson P (2006) Structural investigation of disordered stress proteins: comparison of full-length dehydrins with isolated peptides of their conserved segments. Plant Physiol 141:638–650
Müller K, Tintelnot S, Leubner-Metzger G (2006) Endosperm-limited Brassicaceae seed germination: abscisic acid inhibits embryo-induced endosperm-weakening of Lepidium sativum (cress) and endosperm rupture of cress and Arabidopsis thaliana. Plant Cell Physiol 47:864–877
Nakashima K, Satoh R, Kiyosue T, Yamaguchi-Shinozaki K, Shinozaki K (1998) A gene encoding proline dehydrogenase is not only induced by proline and hypoosmolarity, but is also developmentally regulated in the reproductive organs of Arabidopsis. Plant Physiol 118:1233–1241
Nguyen H, Brown RC, Lemmon BE (2000) The specialized chalazal endosperm in Arabidopsis thaliana and Lepidium virginicum (Brassicaceae). Protoplasma 212:99–110
Oh E, Yamaguchi S, Kamiya Y, Bae G, Chung WI, Choi G (2006) Light activates the degradation of PIL5 protein to promote seed germination through gibberellins in Arabidopsis. Plant J 47:124–139
Ryu HY, Kim SY, Park HM, You JY, Kim BH, Lee JS, Nam KH (2009) Modulations of AtGSTF10 expression induce stress tolerance and BAK1-mediated cell death. Biochem Biophys Res Comm 379:417–422
Shinozaki K, Yamaguchi-Shinozaki K, Seki M (2003) Regulatory network of gene expression in the drought and cold stress responses. Curr Opin Plant Biol 6:410–417
Soitamo AJ, Piippo M, Allahverdiyeva Y, Battchikova N, Aro E (2008) Light has a specific role in modulating Arabidopsis gene expression at low temperature. BMC Plant Biol 8:13. doi:10.1186/1471-2229-8-13
Tompa P, Csermely P (2004) The role of structural disorder in the function of RNA and protein chaperones. FASEB J 18:1169–1175
Vaughan JG, Whitehouse FLS, Whitehouse JM (1971) Seed structure and taxonomy of the Cruciferae. Bot J Linn Soc 64:383–409
Wise MJ, Tunnacliffe A (2004) POPP the question: what do LEA proteins do? Trends Plant Sci 9:13–17
Yamaguchi-Shinozaki K, Shinozaki K (2005) Organization of cis-acting regulatory elements in osmotic- and cold-stress responsive promoters. Trends Plant Sci 10:88–94
Zhu JK (2002) Salt and drought stress signal transduction in plants. Annu Rev Plant Biol 53:247–273
Acknowledgments
This work was supported by the Korean Science and Engineering Foundation (grant # R01-2007-000-20074-0 to K.H.N.) and by the SRC Research Center for Women’s Diseases of Sookmyung Women’s University (2008).
Author information
Authors and Affiliations
Corresponding author
Additional information
Communicated by R. Reski.
Rights and permissions
About this article
Cite this article
Kim, S.Y., Nam, K.H. Physiological roles of ERD10 in abiotic stresses and seed germination of Arabidopsis. Plant Cell Rep 29, 203–209 (2010). https://doi.org/10.1007/s00299-009-0813-0
Received:
Revised:
Accepted:
Published:
Issue Date:
DOI: https://doi.org/10.1007/s00299-009-0813-0