Abstract
A quantitative characterization of growth-related parameters for larval and juvenile Antarctic krill, Euphausia superba (Dana), is essential to understanding the early life history of this key species in the Southern Ocean ecosystem. To this end, instantaneous growth rate experiments were conducted with larval and juvenile krill to determine growth increments and molting frequency in situ. All experiments were carried out during fall and winter months (April through September) on nine separate cruises west of the Antarctic Peninsula between 1987 and 1999. A consistent seasonal pattern across years was observed: growth rates decreased during fall (April/May), were minimal in early winter (June), and increased to maximum rates by late winter (September). Habitat-specific differences (water column vs under-ice) in growth rates of larvae collected on the same cruise were not observed in early winter (June 1993; within-year comparison). However, in a between-year comparison, larvae from the under-ice habitat (June/July 1987) had significantly higher growth increments than larvae from the open-water habitat (July 1989). The difference between these two comparisons may be a function of the degree of contrast in food availability in the water column and the sea ice at different times in the winter. Daylength at the time of collection explained 74% of the variation in larval and juvenile growth rates. This correlation may be an indirect effect of the influence of diel cycles on krill behavior and/or primary production in both the water column and ice.
Similar content being viewed by others
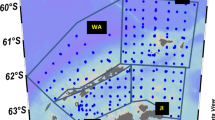
Avoid common mistakes on your manuscript.
Introduction
Antarctic krill, Euphausia superba, plays a key role in the structure and function of the Southern Ocean ecosystem, serving as both an important grazer (Quetin et al. 1996) and a critical prey item for the reproductive success of seals and seabirds (Croxall et al. 1999; Everson 2000). The effects of variations in the life cycle, annual production (growth), and recruitment success in Antarctic krill can be traced throughout the ecosystem.
The life cycle of Antarctic krill is keyed to the seasonal cycles of production in the Southern Ocean. E. superba release their embryos during the austral summer, and the larvae develop through a series of naupliar, calyptopis, furcilia, and juvenile stages throughout the following 11 months (Fraser 1936; Ikeda 1984; Quetin et al. 1994). During the first year of life, there may be two periods critical for larval survival. The first is immediately after developing to the first feeding stage, when a larva must find adequate food within 10–14 days to survive (Ross and Quetin 1989). The second is during the first winter when larvae must feed (Ross and Quetin 1991; Quetin et al. 1996), but food in the water column is scarce (Smith et al. 1996).
Only a few studies have addressed the question of growth in situ of larval Antarctic krill during winter (Daly 1990; Ross and Quetin 1991). Ross and Quetin (1991) showed that winter growth in larval krill varied interannually, and correlated the differences with the availability of the under-ice habitat. In laboratory and field studies, development and growth clearly vary with environmental conditions such as temperature and food availability (Ikeda 1984; Ross et al. 1988; Elias 1990), leading to both direct and indirect development (Brinton and Townsend 1984; Brinton et al. 1986). Although larval development continues throughout the fall, in winter months late furcilia stages consistently dominate the larval population (Fraser 1936; Daly 1990; Frazer et al. 2002), suggesting that development may slow down in winter. Variations in growth rates in early life history have been correlated with year class strength in fishes such as cod, haddock, and herring (see Houde 1987; and also Ottersen and Loeng 2000). Several investigators (Daly 1990; Ross and Quetin 1991) have suggested a similar mechanism may hold for Antarctic krill. Thus, variations in growth and development of larval krill, especially during periods of low primary production in the fall and winter, may increase our understanding of the role of larval growth in the life cycle and population dynamics of this ecologically important species.
Several lines of evidence have converged over the past two decades to suggest that larval krill survive the winter by exploiting food resources associated with annual sea ice. Larval and juvenile krill have frequently been observed feeding on the under-ice surfaces in winter (e.g. Guzman 1983; Marschall 1988; Stretch et al. 1988; Daly 1990; Quetin et al. 1994; Frazer 1996; Frazer et al. 1997a, 2002). Also Ross and Quetin (1991) found that larval krill from the pack-ice habitat exhibited higher growth rates, higher condition factors, and higher lipid concentrations than larvae collected from open water. Thus, both behavioral observations and differences in physiological condition suggest that larvae feed on the sea ice microbial community (SIMCOs) and experience better nutritional conditions in the under-ice habitat than larvae living in open water. In addition, Siegel and Loeb (1995) and Quetin and Ross (2003), in two time-series from different regions west of the Antarctic Peninsula, have demonstrated a strong correlation between recruitment success of krill and the timing and extent of sea ice formation during the winter. These correlations provide support for the assumption that SIMCOs are an essential food resource for winter survival (Daly 1990; Smetacek et al. 1990; Ross and Quetin 1991; Quetin et al. 1994, 1996). Under this scenario, winter growth of larvae will depend on the dynamics of the seasonal sea ice and the availability of the SIMCOs.
In the present study, we document seasonal and interannual variability in growth rates in larval Antarctic krill from April through September, a time period of low food availability in the water column (Smith et al. 1996). Recently, Ross et al. (2000) demonstrated that growth rates of larval and juvenile krill during the austral spring and early summer (November through mid-January) were a function of the abundance and composition of the phytoplankton community in the water column. Additional environmental factors may impact larval growth in winter, however. In particular, the presence and availability of SIMCOs associated with sea ice and whether they are available to the grazing larvae may be an important determinant of growth.
During a series of nine cruises west of the Antarctic Peninsula in fall and winter months, we conducted instantaneous growth rate (IGR) experiments (see Ross et al. 2000) with larval and juvenile krill to determine growth increments and molting frequency in situ. In addition, we characterized the stage composition of larvae and their molts to determine the percentage of individuals exhibiting direct or delayed development during winter. Multiple cruises in the same year (1991 and 1993) and in the same season (June in 3 years, September in 2 years) allowed us to document: (1) patterns in seasonal growth of the same year class, and (2) interannual variability in growth at the same time of year. Chlorophyll a (chl a) concentrations were used as an index of food availability in the water column, and the presence of seasonal sea ice during specific cruises was used as an indicator of SIMCOs and their potential availability as a food resource for larval krill. On three of the nine cruises, chl a associated with the under-ice surfaces was quantified. In addition, the molting frequency of larvae maintained in the laboratory under different food regimes was monitored to investigate variation in molting frequency of the same population sampled at various times in the molt cycle. These experiments were undertaken to help us understand the dynamics of molting frequency under controlled conditions, and allowed us to evaluate the concept of synchronous molting and to interpret in situ molting frequency results.
Materials and methods
Research cruises
The nine research cruises reported all took place in fall and winter months, aboard the M.V. "Polar Duke" or the R.V.I.B. "Nathaniel B. Palmer", in six of the years between 1987 and 1999. The study region was west of the Antarctic Peninsula, from the southern Bransfield Strait to just south of Marguerite Bay, and the majority of the collection sites were seaward of Adelaide Island (Fig. 1). Experimental dates each year were between late April and late September, although no experiments were conducted in August (Table 1). Three time periods during the fall and winter were defined: fall, April through May; early winter, June through mid-July; late winter, September.
Study region west of the Antarctic Peninsula. Numbers refer to locations of larval collections for growth experiments conducted on board ship in June/July 1987 (1), July 1989 (2), May 1991 (3), September 1991 (4), April/May 1993 (5), June 1993 (6), September 1993 (7), June/July 1994 (8), and June 1999 (9)
Larvae of Euphausia superba (Dana) used in experiments were from the water column and the under-ice habitats, and were collected with nets or by SCUBA divers, depending on ice and weather conditions (Table 1). Several different nets were used. Either a 1-m Bongo net or a 1-m ring net, both with 505-µm mesh, were used for all cruises except the austral fall cruise in 1993 when a 2-m-square, fixed-frame net with ~700-µm mesh was used. Tows were short (generally <15 min) so the larvae were in good physiological condition when they arrived at the surface. Larvae were collected from under the ice by SCUBA divers with fine-meshed (~680-µm mesh) aquarium nets. Half the experiments with larvae from the water column were in the fall prior to the seasonal advance of sea ice. However, during the early winter of 1989 all experiments were with larvae from the water column since there was no ice habitat in the study region. During early winter of 1993, half of the experiments were with larvae from the water column and half with larvae from under the sea ice (Table 1) to compare the growth of larvae in the same year class from the two habitat types. Results are presented both as a composite and as a single year for 1993 when experiments were conducted throughout the fall and winter on three separate cruises.
Shipboard instantaneous growth rate experiments
Immediately after collection the contents of the cod end or the aquarium net were gently placed into large buckets of seawater at in situ temperatures. Larvae from each collection were randomly selected for subsequent growth experiments. The IGR method was briefly described as a method to measure field growth rates for krill by Quetin and Ross (1991). Nicol et al. (1992) demonstrated, in subsequent work, that the underlying premise that maintenance conditions do not affect the length increment at ecdysis is valid for experimental durations of up to 7 days at no food and 0°C. Further details of the experimental protocol were described by Ross et al. (2000). Briefly, IGR experiments were started within a few hours of collection and lasted 4 days. Between 35 and 100 randomly selected larvae were individually placed in separate containers filled with 1.2-µm-filtered seawater. The volume of the experimental containers was 125 or 500 ml depending on the size and stage of the larvae. Larvae were held at ambient seawater temperatures in the dark, except for 30-min exposures to low light every 12 h when all containers were inspected for molts. Upon molting, the larva and its molt were preserved in 10% buffered formalin. At the end of the experiment all non-molters were preserved together.
The total length (TL) from the tip of the rostrum to the end of the uropods of each larva in the experiment was measured under a dissecting microscope (standard length 1; Mauchline 1980), and the developmental stage determined according to Fraser (1936). In addition, the telson length was measured for both the molter and its molt, and the stage of the molt determined. Molting frequency was calculated as the percent of larvae molting per day, and the inter-molt period (IMP) estimated as the inverse of the molting frequency. The growth increment was calculated as the difference between the post- and pre-molt telson lengths, divided by the pre-molt telson length, and reported as the percent linear growth. The number of krill for which the growth increment could be calculated ranged between 6 and 38 in most of the experiments (41 of 52). In the remainder of the experiments, from 2 to 5 molters (6 of 52) or 0 or 1 molter (5 of 52) were measured. Mean growth increment (percent IMP−1) and SE were calculated for each experiment. In addition, we calculated the percentage of molters that advanced to the next larval stage for each stage for each cruise to illustrate whether development was direct (advancing a stage at a molt) or delayed (remaining at the same stage at a molt). A developmental stage index (DSI) was calculated that allowed us to use a single value to characterize the stage composition of each experimental population. Each furcilia stage was assigned a number, 1 for furcilia 1, 2 for furcilia 2, ...6 for furcilia 6, and 7 for juveniles, and the DSI was calculated as: DSI=Σ(number larvae in stage i×i)/(total number of larvae), where i is 1 through 7.
Environmental variables
Environmental variables included daylength, temperature, on-site observations of ice cover at the time of collection, concentrations of chl a in the water column, and chl a in samples from under-ice surfaces. Daylength was calculated for the day of the year and the year at the collection location for each experiment. Water temperature at the surface (0–5 m) at the time of collection of the experimental krill was either measured or assumed to be −1.8°C when krill were in the under-ice habitat.
Sampling protocols for determining chl a concentrations available to the larvae in the water column varied. Therefore, we used these data only as an index of the general food quantity available to larval krill in open water and in the water column below the ice. Discrete water samples were taken either from water bottles triggered during a CTD cast or from water bottles triggered by a diver 1–5 m under the ice. Fluorometer calibration and sample analysis were done according to Smith et al. (1981). For all but two of the cruises, glass fiber filters (Whatman GF/C, pore size 0.45 µm) were used to collect and concentrate algal material for chl a analysis. The pore size of the filters was close to the 1-µm sieve size of the feeding appendages of the larvae (Marschall 1985). In April/May and September 1993, 0.45-µm Millipore membrane filters were used. Filters were placed in 10 ml of 90% acetone, and the vials placed in a refrigerator or freezer for 24 h. The extract was then read on a Turner Designs fluorometer. The chl a and phaeopigment concentrations were calculated according to the most recent fluorometer calibration, the volume of water filtered, filter blanks, and dilution factors. The concentrations were expressed as milligrams of chl a per cubic meter.
Although water was always sampled from the upper 40 or 50 m, the depths were not the same on all cruises (see Table 2). For the five WinCruise cruises and the May 1991 cruise, sampling depths were either surface (0–5 m), 20 m, or the average of samples from 0, 20, and 40 m. For the LTER cruises, chl a was integrated over the upper 50 m. However, during fall and winter, the upper layer is generally well mixed and homogeneous (Smith et al. 1996), so all sampling depths in the upper 50 m were assumed to be representative of the upper water column.
Samples for chl a analysis from the under-ice habitat were taken either with a fine-meshed aquarium net (May 1991) or by SCUBA divers with suction samplers, as during the September 1993 and June 1994 cruises. The suction samplers pulled loose material from under-water ice surfaces at a constant rate until a 600-ml plastic cylinder was full. In order to compare concentrations at the ice surface with concentrations in the water column we standardized chl a in the suction samplers to the 600-ml sample volume of the samplers. Samples were taken from upward-, downward-, and side-facing (vertical) ice surfaces. Depending on the hardness of the ice, the sampler removed 0–5 mm of the ice surface. Ice was characterized as hard if the suction tube penetrated <1 mm; otherwise it was characterized as soft.
Laboratory experiments
Data on the molting frequency of larval Antarctic krill maintained at winter temperatures in the laboratory were collected during the carbon and nitrogen turnover experiments reported by Frazer et al. (1997b). Larval krill were held during the winter of 1993 in the laboratory at Palmer Station at −1.5°C and kept in the dark except when they were checked, fed, or the water changed under red light. Larvae were either starved for 2 months or fed for 2.5 months. The fed group was started with 30–33 larvae each in ten 2-l glass jars placed on a roller stirrer. A mixture (1:1 by weight) of two commercial larval shrimp feeds was used as a food source: (1) AP100 formulated by Zeigler Brothers (P.O. Box 95, Gardners, PA 17324) and (2) A250 formulated by BioKyowa (P.O. Box 1550, Cape Girardeau, MO 63702-1550). Daily food ration was approximately 25% of the total body carbon of all larvae in a 2-l jar. Water in the jars was changed every 2 days. The starved group was started with 100 larvae in separate 0.5-l jars to prevent cannibalism, and water in the jars was not stirred. The water in the jars was changed at 2-week intervals. Larvae were counted and molts from each jar in both treatments collected and counted daily.
For each day of the experimental period the molting frequency of the larvae in the starved and fed treatments was calculated by dividing the sum of the molts collected by the number of krill in a treatment the previous day. Data on daily molting frequency were used to simulate the molting frequency of this larval population if placed in experiments initiated at 4-day intervals over the winter. We calculated the molting frequency and IMP for "4-day experiments" by summing the molts and experimental numbers for sequential 4-day intervals.
Results
Shipboard instantaneous growth rate experiments
The number of experiments per cruise varied from two to ten (Table 1), for a total of 52 experiments, of which one had zero molters. Two additional experiments only provided information on molting frequency as growth increments could not be determined due to damaged telsons. Thus we have 51 estimates of molting frequency and 49 estimates of the average growth increment and proportion of the molters advancing a stage during the experiment.
During the fall and winter the larval DSI increased from an average of slightly >4 (furcilia 2 to furcilia 5) in April and May to between 5 and 6 in June (primarily furcilia 4 to furcilia 6) (Fig. 2). The DSI remained at about 6 (primarily furcilia 6 with a few furcilia 5 and juveniles) for the remainder of the winter. This pattern was similar for the fall and winter of 1993 (three cruises) and all cruises combined. The exception was experiments from 1989, when the DSI averaged <4 in July. The lower DSI compared to other early winters could be an indication of delayed development or late spawning and slowed development. In June 1993 the DSI for the five experiments with larvae collected from the under-ice habitat was not significantly different from that for larvae collected from open water (ANOVA, F=0.41, P=0.54). The mean DSIs were 5.56 and 5.45, respectively.
In general, the average total length of larvae in a given experiment increased through the fall, but remained relatively unchanged throughout the winter (Fig. 3). Larvae collected in July 1989, however, were significantly smaller (6 mm) than larvae collected earlier in the season in other years (7–11 mm). Average total length varied more widely in space and time than the DSI. First, in June 1993 the average total length of larvae collected from under the ice was significantly greater than that of larvae from open water further north (ANOVA, F=22.9, P=0.001), in contrast to the lack of significant difference in the DSI. Second, there was a clear separation between the average total lengths of larvae collected in September, with larvae in 1993 smaller than those collected in 1991, and, again, no difference in the DSI. These results suggest that the temporal developmental progression was less variable than growth rate.
Euphausia superba. Average total length with standard error of larvae in growth experiments throughout the fall and winter. Circles are 1993, with filled circles for under-ice habitat and open circles for open-water habitat. All other years are crosses. Experiments from July 1989 are encircled crosses
Adequate numbers of molters were found in four larval stages to characterize the percent of larvae at a stage advancing to the next stage at a molt during the fall and winter (Table 3). In general the majority of larvae advanced to the next stage at molting (direct development) until they reached furcilia 6. In the fall, half of the furcilia 3 advanced to furcilia 4 at molting; this percentage increased to >85% in early winter. Over two-thirds of the furcilia 4 advanced to furcilia 5 at molting, with a somewhat higher percentage of individuals advancing in the early winter period. For furcilia 5, over three-quarters advanced to furcilia 6 at molting in the fall and early winter, whereas less than two-thirds advanced in late winter. The pattern for furcilia 6 larvae was different. With the exception of the June/July 1987 cruise, very few furcilia 6 larvae advanced to juvenile (delayed development).
The IMP for the experiments was generally 10–50 days (Fig. 4), with seven experiments resulting in significantly higher IMPs (1 with zero molters, 2 ~180 days, and 4 between 64 and 96 days). All the high IMPs were observed in either fall or early winter, including all three IMPs determined on the July 1989 cruise (Fig. 4). During fall, early winter, and late winter the median IMPs were 23.4, 30.5, and 23.0 days, respectively. However, there was no statistical difference in the IMP among the three seasons (ANOVA, F=1.59, P=0.21), even when the two IMPs >100 days were eliminated (F=2.48, P=0.09). Likewise, there was no significant difference in the IMP for larvae from the under-ice habitat when compared with those collected from the open-water habitat in June 1993 (ANOVA, F=0.75, P=0.42).
Euphausia superba. Intermolt period from growth experiments conducted with larvae in fall and winter. Circles are 1993, with filled circles for under-ice habitat and open circles for open-water habitat. All other years are crosses. Experiments from July 1989 are encircled crosses. Two values were >100 days: 120th day of the year, 188 days; 182nd day of the year, 184 days
The percent growth per IMP (i.e. growth increment) of larval krill in fall and winter decreased through the fall and early winter, then increased in late winter, when growth increments were more than twice those of rates in early winter (Fig. 5). Seasonal differences were greater than interannual differences, as shown by the clusters of growth increments. Average growth increments in the first cluster were 0–3% IMP−1 and were represented by data collected during four early winter cruises (1987, 1993, 1994, 1999). Average growth increments in the second cluster (represented by data from the late winter cruises in 1991 and 1993) were 10–12% IMP−1. Average growth increments ≤0 were observed only during the early winter period, e.g. for three of the ten experiments conducted during the June 1993 cruise, and for all experiments during the July 1989 and June 1999 cruises. The growth increments for larvae in early winter 1993 from the under-ice (n=66 individuals) and open-water (n=62) habitats were not significantly different (ANOVA, F=0.064, P=0.80), 0.90% vs 0.73%, respectively.
Euphausia superba. Percent growth per IMP (growth increment) with standard error for larvae in growth experiments in fall and winter. Circles are 1993, with filled circles for under-ice habitat and open circles for open-water habitat. All other years are crosses. Experiments from July 1989 are encircled crosses. The horizontal line represents zero growth
Multiplying the growth increment by the IMP yielded growth rates expressed in millimeters per day, which can be compared to results for cohort analysis. For this study we used the median IMP of 26.7 days because there were no significant differences in the IMP among seasons, and the large variability seen in experimentally determined IMPs was believed to be due to synchronous molting (see below). The linear growth rates showed the same pattern as the growth increment, with low or negative growth rates observed for some experiments conducted between mid-June and late July (Fig. 6). Linear growth rates were at their maximum in September, namely 0.04 and 0.055 mm day−1.
Environmental variables
The pattern of seasonal variation in the growth increment for larval krill was evident. Two possible abiotic parameters affecting the growth increment include environmental temperature and daylength. Food availability was a possible biotic factor. In this context we viewed ice cover as a proxy for the availability of SIMCOs (algae in particular) as food for larval krill. Environmental temperatures in the surface water, i.e. 0–5 m, were near zero ranging down to −1.8°C in this study (Table 2). Under-ice temperatures were assumed to be −1.8°C. During the June 1993 cruise, half of the experiments were conducted with larval krill from under the ice and the other half with larval krill from waters at −1.05°C. As previously shown, there was no significant difference in growth increment for these two groups of larvae. Some of the lowest growth increments (negative) were actually found in larvae collected from water at −0.5°C to −0.6°C.
Another seasonal environmental variable was daylength. Daylength at the time of collection accounted for 74% of the variability in the average growth increment of larval krill in the fall and winter (Fig. 7): % growth IMP−1=0.984 (daylength in h)−0.868.
Euphausia superba. Percent growth per IMP (growth increment) versus daylength at the location where larvae were collected. Circles are 1993, with filled circles for under-ice habitat and open circles for open-water habitat. All other years are crosses. Experiments from July 1989 are encircled crosses. The gray horizontal line represents zero growth. The bold line represents the linear regression: growth increment=0.984 (daylength in h)−0.868, r 2=0.74, n=49
The contrast between the perceived effect of habitat within a year (June 1993 cruise) versus environmental conditions between years (June/July 1987 and July 1989 cruises) was striking (Figs. 2, 3, 4, 5). In June 1993 (early winter) there was no significant difference in DSI, IMP, or growth increment between larvae from open-water versus the under-ice habitats. Yet between years and a few weeks later in early winter, larvae from the under-ice habitat (June/July 1987) had significantly higher growth increments than larvae from the open-water habitat (July 1989), as first reported by Ross and Quetin (1991).
Chl a concentrations in the water column and in the under-ice surface yielded additional information about food availability. During the fall, chl a concentrations were low (0.3–0.7 mg m−3), but two to three times those found in winter (Table 2). Concentrations in early winter usually averaged about 0.10 mg m−3, but on occasion concentrations were >0.20 mg m−3. In late winter, chl a concentrations in the water column were slightly higher, averaging 0.13–0.21 mg m−3 (Table 4). Concentrations of chl a available to the grazing krill in the ice were only determined on three cruises. Chl a in the ice scoop in late May were ten times higher than in the water column, but still quite low (0.01 mg m−3). The suction samples from early winter showed concentrations of about 0.10 mg m−3, similar to concentrations in the water column. However, suction samples taken from the under-ice surface in September showed chl a concentrations 4–15 times those in the water column, depending on the hardness of the ice (Table 2).
Laboratory experiments
The daily molting frequency of larvae held at winter temperatures (−1.5°C) under different feeding conditions over a 2.5-month period showed distinct peaks that varied in number and amplitude with feeding conditions (Fig. 8a). The mean molting frequency for fed larvae was 4.4% day−1 (SD=1.53) and that for starved larvae was 1.5% day−1 (SD=1.4). The peaks in molting frequency were more distinct for the fed larvae than for the starved larvae, as illustrated by molting frequencies of >6% day−1 on 11–13 July, 1–4 August, 18–19 August, and 23–24 August (arrows on Fig. 8a). A significant autocorrelation in molting frequency for the fed larvae illustrates that under some conditions molting synchrony occurs. Molting frequency for fed larvae was positively correlated with its lagged value (r=0.31, P=0.02, n=55), whereas, the correlation for starved larvae was not significant (r=0.16, P=0.40, n=31).
Euphausia superba. Molting frequency of starved and fed larvae held at −1.5°C in the laboratory at Palmer Station in the winter of 1993. a Daily molting frequency over the experimental period. Arrows and horizontal bars indicate two or more of three sequential days with elevated molting frequency. b Frequency distribution of simulated IMP for sequential 4-day experiments calculated on the data in panel a (black line for fed treatment; gray line for starved treatment)
Although the majority of the IMPs simulated with these data were below 50 days, as for the ship-based IGR data, there were some estimates in the 50- to 170-day range, primarily for the starved larvae (Fig. 8b). The median IMP for starved larvae was significantly longer (61.6 days) than that of fed larvae (26.0 days) (Table 4). The median IMP for fed larvae was in the same range as those reported for larvae in situ during the fall and winter.
Discussion
Most estimates of growth rates in euphausiids in general, and Euphausia superba in particular, have depended on the analysis of sequential length-frequency distributions (Siegel and Nicol 2000). Such analyses have claimed to demonstrate phenomena ranging from shrinkage of adults in winter (McClatchie et al. 1991) to high growth rates of larval krill in winter (Daly 1990). Recently Reid (2001) used sequential length-frequency distributions from krill in penguin stomachs to estimate summer growth rates in adult krill near South Georgia. The length-frequency distributions and the inferred growth rates have also been used to drive models of growth in Antarctic krill to predict the rates of ingestion required in winter (Hofmann and Lascara 2000). However, there are critical disadvantages to the analysis of length frequencies as a technique to measure growth in situ. First, one has to assume that the same population is sampled over time; second, that mortality is not size dependent; and lastly, that the length of the sampling interval is not a factor (Nicol 2000; Ross et al. 2000). With the IGR method, first outlined by Quetin and Ross (1991), short-term experiments with freshly collected krill are likely to reflect the natural growth rate of individuals in the field (Nicol et al. 1992; Nicol 2000). Assumptions regarding population constancy and size-independent mortality do not have to be made. In addition, growth can be measured in relation to environmental factors over small temporal and spatial scales impossible for length-frequency distribution analysis. For example, Ross et al. (2000) demonstrated that 70% of the variability in the growth increment of juvenile Antarctic krill in austral spring can be explained by short-term variation in food quantity (chl a) and quality (phytoplankton community composition).
In the present study the IGR method was used to measure growth rates of larval Antarctic krill in the field during the fall and winter when primary productivity in the water column is low (Smith et al. 1996). Interannual and within-season variation in growth rates of larval krill in the fall and winter and the effect of habitat were examined. The study by Ross et al. (2000) and the present study compared the growth increment (% growth IMP−1), not growth in millimeters per day. The advantages of the growth increment are twofold: (1) the growth increment does not increase with total length as is true of the growth rate and (2) single estimates of the intermolt period in populations of krill in situ are uncertain due to possible synchrony in molting.
Variability in estimates of the IMP was large in this study, as Ross et al. (2000) also found during the spring. However, the IMP did not show any pattern in variation over the fall and winter, leading to a single estimate, ~4 weeks, for the IMP of larval krill in fall and winter, longer than the 18.3-day estimate for young-of-the year Antarctic krill in spring. The somewhat prolonged IMP during fall and winter months was a result of the known effects of temperature on the IMP (reviewed by Quetin et al. 1994). Prior to this study, data on IMPs of larval krill in winter were limited to a single estimate of 20 days (Daly 1990), which is within the range of the majority of the estimates of the IMP in this study.
Growth data for larval krill under fall or winter conditions are limited (reviewed by Quetin et al. 1994), and range from 0.07 mm day−1 from length-frequency distributions taken 40 days apart (Daly 1990) to 0.02 mm day−1 for larval krill held in the laboratory under low food regimes (Elias 1990). For comparison, Ikeda (1984) estimated larval growth rates in the laboratory with unlimited food from 0.07 to 0.10 mm day−1. In the present study, growth rates ranged from ~0.005 mm day−1 in early winter to ~0.05 mm day−1 in late winter. Thus, we estimated that total length could increase from 0.45 to 4.5 mm over the 90 days from mid-June to mid-September. The length-frequency data suggested that the increase in total length was <2 mm, e.g. 8–9 mm in mid-June to 10 mm in mid-September in the composite plot. Thus neither the minimum nor the maximum growth rate from the IGR experiments would yield reasonable increases in total length if applied uniformly over the entire winter. The observed change in total length supports the concept of growth rates varying significantly over the winter months.
Synchronicity in molting has been discussed to provide context for molt stage distributions in sampled populations (Buchholz et al. 1989), to explain simultaneous molting observed by divers (Hamner et al. 1983), and to provide a mechanism for the observed distributions of molting frequency of krill in IGR experiments (Ross et al. 2000). In the present study we have shown that synchronicity in molting was maintained in laboratory populations of fed krill over a 2-month period, but that synchronicity was not maintained in starved krill. However, the experiment with starved krill only lasted a month. The shorter experiment (~1 month) combined with the longer mean IMP for starved krill (~2 months) would not allow detection of a significant autocorrelation. Of interest is the fact that extremely long IMPs were only found in krill in the starved treatment. The similarity in the distribution of the simulated IMPs from these data and that of the IMPs of larval krill in IGR experiments in fall and winter was striking and supported the existence of synchronicity in molting in the field in larval krill.
Contrasting IMPs for larval krill under known feeding conditions and those from the field allowed us to better interpret the field results. The median IMP for larval krill in the fall and winter was similar to those found in fed larval krill in the laboratory at winter temperatures, and over twice those of starved larval krill. However, in a few experiments in the fall and early winter, the IMPs fell within the range of laboratory-derived IMPs found only in starved larval krill. Half of the extremely long IMPs were from experiments with larvae collected in open water during the July 1989 cruise, when sea ice advance was delayed (Stammerjohn and Smith 1996). Both the negative growth increment and the extremely long IMPs of these larvae from July 1989 suggested these larvae were starving. The IMP data from our study imply that in most years larval krill in the fall and winter were not starving, or at least had not been without food for long enough to affect the IMP. However, under extreme conditions when sea ice formation is delayed and areal coverage is reduced, such as found during the winter of 1989, the impact of the low food availability can be seen in both the growth increment and the IMP.
The growth increment was strongly correlated with daylength at the time of collection. Several possible mechanisms may underlie this correlation. First larval euphausiids migrate vertically in the summer and fall (Fraser 1936; Nast 1978; Quetin and Ross, unpublished data), but not in the spring (Quetin and Ross, unpublished data). The larvae shift to the under-ice habitat in the early winter, and are usually found either coupled to the under-ice surface or in the water column immediately below when ice is present (Frazer et al. 2002). We do not know if krill larvae exhibit a reverse vertical migration in the early winter, dropping down from the ice–water interface at dark and decreasing the time spent feeding on SIMCOs. Such light-driven behavioral differences would impact total ingestion and, as a consequence, growth.
Alternatively, if food intake in early and late winter is the same, the larvae may allocate the ingested food differently in early versus late winter. Again, daylength may be the cue. If a shortening daylength signals that winter and a low food period is approaching, one strategy might be to invest assimilated matter in storage for reserves instead of using it to grow. Similarly, the increasing daylength in late winter might be a cue to shift the allocation of assimilated food resources to somatic growth. In many organisms, daylength is an environmental cue controlling their developmental patterns. For example, the onset of increasing daylength is the cue for ovarian development in crayfish (Daniels et al. 1994), spiny lobster (Matsuda et al. 2002), and American lobster (Nelson 1986; Waddy and Aiken 1991), and, also, for growth and maturation of salmon (Berg et al. 1994). The existence of this strategy for Antarctic krill could be tested experimentally.
A third possibility is that the correlation between daylength and growth in the fall and winter was an indirect result of the effect of daylength on primary production, both in the water column and the sea ice. The longer the day, the higher the primary production, whether in the water column or in the sea ice. In the fall, when there is no ice cover, primary production in the water column is thought to be light limited. Tilzer and Dubinsky (1987) determined that the break-even daylength for phytoplankton growth ranged from about 2–8 h depending on the water temperature. Thus, in late fall and early winter in our study region, even at near-freezing temperatures, phytoplankton cannot grow. In the winter, primary production will take place primarily in the sea ice, as the sea ice prevents the light from penetrating into the water column. The pattern of accumulation of chl a in the sea ice over the season supports the concept of primary production in sea ice also being light limited. Garrison and Buck (1991) state that conditions favorable for algal growth in sea ice develop in spring as a result of increasing daylength and solar irradiance. Dieckmann et al. (1998) showed a clear minimum in seasonally varying chlorophyll concentrations in sea ice in June, with little increase until November. This alternative receives additional support from the difference in the chl a concentrations at the ice–water interface estimated from the suction samples taken in early winter and late winter, i.e. between a very short daylength to near 10 h light:14 h dark days. This difference may be the result of light limitation on primary production and increase in the standing stock of SIMCOs (algae in particular) in late winter. If this were the case, the synergistic effect of "food availability–daylength" would be due to the effect of daily irradiance on primary production, not the effect of light on behavior leading to variation in ingestion rate as found in larval fish (Boeuf and LeBail 1999).
One last consideration is whether larvae need high concentrations of food to grow. Growth rates in the austral spring were correlated with food concentration, but growth rates of >4% IMP−1 were found at chl a concentrations <1 mg m−3 (Ross et al. 2000). Elias (1990), in a study of growth rates of larvae at winter temperatures and very low food concentrations (0.5 µg chl a l−1), also found slow but positive growth. Thus, it is possible that the SIMCOs are not readily available to krill larvae in early winter during ice formation. Only as the sea ice begins to soften while melting in the late winter and spring would SIMCOs become easily available to the larvae. Some support for this alternative comes from the difference in chl a concentrations between hard and soft surfaces during the same time period.
In an earlier publication (Ross and Quetin 1991), the better physiological condition (growth rates, lipid content, condition factor) of larvae in the winter of 1987 than in the winter of 1989 was attributed to differences in the availability of the sea ice as a winter grazing ground. These two winters, for which krill growth rates were determined in the present study, are examples of extremes in seasonal sea ice dynamics (Stammerjohn et al. 1997). In 1987, sea ice advanced early, and by June the entire region west of the Antarctic Peninsula was covered with ice (Ross and Quetin 1991). In 1989, sea ice extent in the region was the lowest in the satellite record, and the advance was delayed by several months. The contrast was perceived as one of habitat, e.g. larvae from an under-ice habitat in 1987 and an open-water habitat in 1989. These results led to the hypothesis that an "enhancement in winter-over survival of larval krill is mediated by the presence of pack ice".
One of the objectives of the present study was to test whether this difference in physiological condition in larval krill from the two habitats also existed within years, and earlier in the seasonal cycle of sea ice dynamics. In early winter of 1993, sea ice extent and timing were close to the mean pattern for the 21-year satellite record (Stammerjohn et al. 1997), and we were able to collect larvae from ice-covered waters and open waters 100–200 km from the advancing ice edge. Sea ice had not advanced to the region south of Anvers Island by June, but had been present in the location for the "ice-covered" habitat west of Adelaide Island for close to a month. Larval krill from ice-covered waters only differed from larval krill from the open water in their total lengths. However, the increased length of larval krill from the ice habitat could be attributed to that group coming from an earlier spawning or a population with higher growth rates in the summer.
The absence of enhanced growth rates in larvae from the under-ice habitat early in the winter was found during several other early winter cruises. In fact, we did not observe elevated growth increments under the ice until late winter. Thus, the presence of sea ice alone is not likely the unique factor influencing growth rates of larval krill. Also sea ice alone does not appear to be a good proxy for the presence and/or availability of SIMCOs for larval ingestion and growth. At this point in our understanding of larval krill growth in winter, daylength is our best proxy for food availability.
Does this mean that we no longer think the presence of sea ice is important for the growth and survival of larval krill? No, we do not suggest that the concept of SIMCOs in winter sea ice as one food source for larval krill be abandoned, only that we need to incorporate the potential complexities in sea ice–SIMCO–krill interactions into testable mechanisms. The comparison of groups of larvae early in the winter (June) and mid-winter (July) suggests that the contrast in food availability in the water column and under-ice habitats is important because that contrast determines the relative benefits of the two habitats. Not only are there seasonal differences in the concentration of SIMCOs in the sea ice due to the timing of ice formation (C. Fritsen, personal communication) and the level of in situ primary production, but there also may be seasonal differences in whether the SIMCOs in the sea ice are available to grazing krill, whether due to krill behavior or ice dynamics. In fact one might predict that the minimum habitat contrast and absolute abundance are likely to coincide in early winter, when primary production is low and ice is just forming. The contrast will be greatest in early spring when primary production in sea ice is high and open-water blooms are lagging.
In order to understand how sea ice mediates the well-documented correlation between krill recruitment and sea-ice timing and extent (Siegel and Loeb 1995; Quetin and Ross 2003), we need to learn what environmental conditions create an under-ice habitat for krill in which they thrive versus one in which they just barely survive. The results of this study suggest the answers may lie in seasonal comparisons. We need to know how the overriding seasonal pattern in larval growth rates is influenced or driven by such environmental variation as the timing of ice formation and its effect on the growth of the SIMCOs, and the effect of daylength or photoperiod on larval krill behavior and/or physiology.
Conclusion
We observed a strong seasonal pattern in development and growth of larval krill in the fall and winter months. Larvae continued to develop throughout the fall, and were primarily furcilia 5 and 6 in early winter. Growth and development throughout the winter were slow, with growth near zero in early winter. By late winter, growth rates had increased to over twice those in the fall. An unexpected result was that sea ice did not appear to be a good proxy for the presence and/or availability of SIMCOs as food for the larvae. This seasonal pattern in growth held both within a year and between years, and was strongly correlated with daylength. The mechanism underlying the correlation is likely an indirect effect of daylength on either larval krill behavior or primary production in the sea ice.
References
Berg A, Stefansson S, Hansen T (1994) Effect of reduced daylength on growth, sexual maturation and smoltification in Atlantic salmon (Salmo salar) underyearlings. Aquaculture 121:294
Boeuf G, LeBail PY (1999) Does light have an influence on fish growth? Aquaculture 177:129–152
Brinton E, Townsend AW (1984) Regional relationships between development and growth in larvae of Antarctic krill, Euphausia superba from field samples. J Crustac Biol 4:224–246
Brinton E, Huntley M, Townsend AW (1986) Larvae of Euphausia superba in the Scotia Sea and Bransfield Strait in March 1984–Development and abundance compared with 1981 larvae. Polar Biol 5:221–234
Buchholz F, Morris DJ, Watkins JL (1989) Analyses of field moult data: prediction of intermoult period and assessment of seasonal growth in Antarctic krill, Euphausia superba Dana. Antarct Sci 1:301–306
Croxall J, Prince P, Reid K (1999) Diet, provisioning and productivity responses of marine predators to differences in availability of Antarctic krill. Mar Ecol Prog Ser 177:115–131
Daly KL (1990) Overwintering development, growth and feeding of larval Euphausia superba in the Antarctic marginal ice zone. Limnol Oceanogr 35:1564–1576
Daniels WH, Dabramo LR, Graves KF (1994) Ovarian development of female red swamp crayfish (Procambrus clarkii) as influenced by temperature and photoperiod. J Crustac Biol 14:530–537
Dieckmann GS, Eicken H, Haas C, Garrison DL, Gleitz M, Lange M, Nothig E-M, Spindler M, Sullivan CW, Thomas DN, Weissenberger J (1998) A compilation of data on sea ice algal standing crop from the Bellingshausen, Amundsen and Weddell Seas from 1983 to 1994. In: Lizotte MP, Arrigo KR (eds) Antarctic sea ice: biological processes, interactions and variability. Antarct Res Ser 73:85–92
Elias MC (1990) Effects of photoperiod, phytoplankton level and temperature on the growth, development and survival of larval Euphausia superba (Dana). Master of Arts in Biology, University of California, Santa Barbara
Everson I (2000) Role of krill in marine food webs: the Southern Ocean. In: Everson I (ed) Krill: biology, ecology and fisheries. Blackwell, Oxford, pp 194–201
Fraser FC (1936) On the development and distribution of the young stages of krill (Euphausia superba). Discov Rep 14:1–192
Frazer TK (1996) Stable isotope composition (δ13C and δ15N) of larval krill, Euphausia superba, and two of its potential food sources in winter. J Plankton Res 18:1413–1426
Frazer TK, Quetin LB, Ross RM (1997a) Abundance and distribution of larval krill, Euphausia superba, associated with annual sea ice in winter. In: Battaglia B, Valencia J, Walton DWH (eds) Antarctic communities: species, structure and survival. Cambridge University Press, Venice, Italy, pp 107–111
Frazer TK, Ross RM, Quetin LB, Montoya JP (1997b) Turnover of carbon and nitrogen during growth of larval krill, Euphausia superba Dana: a stable isotope approach. J Exp Mar Biol Ecol 212:259–275
Frazer TK, Quetin LB, Ross RM (2002) Abundance, sizes and developmental stages of larval krill, Euphausia superba, during winter in ice-covered seas west of the Antarctic Peninsula. J Plankton Res 24:1067–1077
Garrison DL, Buck KR (1991) Surface-layer sea ice assemblages in Antarctic pack ice during the austral spring: environmental conditions, primary production and community structure. Mar Ecol Prog Ser 75:161–172
Guzman O (1983) Distribution and abundance of Antarctic krill (Euphausia superba) in the Bransfield Strait. In: Schnack SB (ed) On the biology of krill Euphausia superba. Alfred-Wegener-Insitute for Polar Research, Bremerhaven, pp 169–190
Hamner WM, Hamner PP, Strand SW, Gilmer RW (1983) Behavior of Antarctic krill, Euphausia superba: chemoreception, feeding, schooling and molting. Science 220:433–435
Hofmann EE, Lascara CM (2000) Modelling the growth dynamics of Antarctic krill Euphausia superba. Mar Ecol Prog Ser 194:219–231
Houde ED (1987) Fish early life dynamics and recruitment variability. Am Fish Soc Symp 2:17–29
Ikeda T (1984) Development of the larvae of the Antarctic krill (Euphausia superba Dana) observed in the laboratory. J Exp Mar Biol Ecol 75:107–117
Marschall H-P (1985) Structural and functional analyses of the feeding appendages of krill larvae. In: Siegfried WR, Laws RM, Condy PR (eds) Antarctic nutrient cycles and food webs. Springer, Berlin Heidelberg New York, pp 346–354
Marschall H-P (1988) The overwintering strategy of antarctic krill under the pack-ice of the Weddell Sea. Polar Biol 2:245–250
Matsuda H, Takenouchi T, Yamakawa T (2002) Effects of photoperiod and temperature on ovarian development and spawning of the Japanese spiny lobster Panulirus japonicus. Aquaculture 205:385–398
Mauchline J (1980) Measurement of body length of Euphausia superba Dana. BIOMASS handbook no. 4, SCAR/SCOR/IABO/ACMRR
McClatchie S, Rakusa-Suszczewski S, Filcek K (1991) Seasonal growth and mortality of Euphausia superba in Admiralty Bay, South Shetland Islands, Antarctica. ICES J Mar Sci 48:335–342
Nast F (1978) Vertical distribution of larval and adult krill (Euphausia superba Dana) on a time station south of Elephant Island, South Shetlands. Meeresforschung 27:103–118
Nelson K (1986) Photoperiod and reproduction in lobsters (Homarus). Am Zool 26:447–457
Nicol S (2000) Understanding krill growth and aging: the contribution of experimental studies. Can J Fish Aquat Sci 57:168–177
Nicol S, Stolp M, Cochran T, Geijsel P, Marshall J (1992) Growth and shrinkage of Antarctic krill (Euphausia superba Dana) from the Indian sector of the Southern Ocean during summer. Mar Ecol Prog Ser 89:175–181
Ottersen GB, Loeng H (2000) Covariability in early growth and year-class strength of Barents Sea cod, haddock and herring: the environmental link. ICES J Mar Sci 57:339–348
Quetin LB, Ross RM (1991) Behavioral and physiological characteristics of the Antarctic krill, Euphausia superba. Am Zool 31:49–63
Quetin LB, Ross RM (2003) Episodic recruitment in Antarctic krill, Euphausia superba, in the Palmer LTER study region. Mar Ecol Prog Ser (in press)
Quetin LB, Ross RM, Clarke A (1994) Krill energetics: seasonal and environmental aspects of the physiology of Euphausia superba. In: El-Sayed S (ed) Southern Ocean ecology: the BIOMASS perspective. Cambridge University Press, Cambridge, pp 165–184
Quetin LB, Ross RM, Frazer TK, Haberman KL (1996) Factors affecting distribution and abundance of zooplankton, with an emphasis on Antarctic krill, Euphausia superba. In: Ross RM, Hofmann EE, Quetin LB (eds) Foundations for ecological research west of the Antarctic Peninsula. American Geophysical Union, Washington, DC, pp 357–371
Reid K (2001) Growth of Antarctic krill Euphausia superba at South Georgia. Mar Biol 138:57–62
Ross RM, Quetin LB (1989) Energetic cost to develop to the first feeding stage of Euphausia superba Dana and the effect of delays in food availability. J Exp Mar Biol Ecol 133:103–127
Ross RM, Quetin LB (1991) Ecological physiology of larval euphausiids, Euphausia superba (Euphausiacea). Mem Queensl Mus 31:321–333
Ross RM, Quetin LB, Kirsch E (1988) Effect of temperature on developmental times and survival of early larval stages of Euphausia superba Dana. J Exp Mar Biol Ecol 121:55–71
Ross RM, Quetin LB, Baker KS, Vernet M, Smith RC (2000) Growth limitation in young Euphausia superba under field conditions. Limnol Oceanogr 45:31–43
Siegel V, Loeb V (1995) Recruitment of Antarctic krill (Euphausia superba) and possible causes for its variability. Mar Ecol Prog Ser 123:45–56
Siegel V, Nicol S (2000) Population parameters. In: Everson I (ed) Krill: biology, ecology and fisheries. Blackwell, Oxford, pp 103–149
Smetacek V, Scharek R, Nothig E-M (1990) Seasonal and regional variation in the pelagial and its relationship to the life history cycle of krill. In: Kerry KR, Hempel G (eds) Antarctic ecosystems: ecological change and conservation. Springer, Berlin Heidelberg New York, pp 103–114
Smith RC, Baker KS, Dustan P (1981) Fluorometer techniques for measurement of oceanic chlorophyll in the support of remote sensing. Scripps Institution of Oceanography, University of California, ref. 81-17, La Jolla
Smith RC, Dierssen H, Vernet M (1996) Phytoplankton biomass and productivity in the western Antarctic Peninsula region. In: Ross RM, Hofmann EE, Quetin LB (eds) Foundations for ecological research west of the Antarctic Peninsula. American Geophysical Union, Washington, DC, pp 333–356
Stammerjohn SE, Smith RC (1996) Spatial and temporal variability of western Antarctic Peninsula sea ice coverage. In: Ross RM, Hofmann EE, Quetin LB (eds) Foundations for ecological research west of the Antarctic Peninsula. American Geophysical Union, Washington, DC, pp 81–104
Stammerjohn SE, Baker KS, Smith RC (1997) Sea ice indexes for Southern Ocean regional marine ecology studies. Scripps Institution of Oceanography, University of California, ref. 97-01, La Jolla
Stretch JJ, Hamner PP, Hamner WM, Michel WC, Cook J, Sullivan CW (1988) Foraging behavior of antarctic krill Euphausia superba on sea ice microalgae. Mar Ecol Prog Ser 44:131–139
Tilzer MM, Dubinsky Z (1987) Effects of temperature and daylength on the mass balance of Antarctic phytoplankton. Polar Biol 7:35–42
Waddy SL, Aiken DE (1991) Egg production in the American lobster, Homarus americanus. In: Wenner A, Kuris A (eds) Crustacean egg production. Balkema, Rotterdam, pp 267–290
Acknowledgements
These experiments were conducted over an extended period of time. We wish to gratefully acknowledge the help of the many individuals on the nine research teams and the captains and crews of the research vessels during the fall and winter cruises. This study would have been impossible without their assistance. This material is based upon work supported by the National Science Foundation under award nos. DPP-8518872, DPP-8820589, and OPP-9117633 (for the WinCruises), and award nos. OPP-9011927 and OPP-9632763, and by the Regents of the University of California and the University of California at Santa Barbara through the LTER cruises. This is Palmer LTER contribution no. 231. The experiments complied with the current laws of the USA.
Author information
Authors and Affiliations
Corresponding author
Additional information
Communicated by J.P. Grassle, New Brunswick
Rights and permissions
About this article
Cite this article
Quetin, L.B., Ross, R.M., Frazer, T.K. et al. Growth of larval krill, Euphausia superba, in fall and winter west of the Antarctic Peninsula. Marine Biology 143, 833–843 (2003). https://doi.org/10.1007/s00227-003-1130-8
Received:
Accepted:
Published:
Issue Date:
DOI: https://doi.org/10.1007/s00227-003-1130-8