Abstract
Aims/hypothesis
Our earlier studies have established the role of 12/15-lipoxygenase (LO) in mediating the inflammatory reaction in diabetic retinopathy. However, the exact mechanism is still unclear. The goal of the current study was to identify the potential role of endoplasmic reticulum (ER) stress as a major cellular stress response in the 12/15-LO-induced retinal changes in diabetic retinopathy.
Methods
We used in vivo and in vitro approaches. For in vivo studies, experimental diabetes was induced in wild-type (WT) mice and 12/15-Lo (also known as Alox15) knockout mice (12/15-Lo−/−); ER stress was then evaluated after 12–14 weeks of diabetes. We also tested the effect of intravitreal injection of 12-hydroxyeicosatetraenoic acid (HETE) on retinal ER stress in WT mice and in mice lacking the catalytic subunit of NADPH oxidase, encoded by Nox2 (also known as Cybb) (Nox2−/− mice). In vitro studies were performed using human retinal endothelial cells (HRECs) treated with 15-HETE (0.1 μmol/l) or vehicle, with or without ER stress or NADPH oxidase inhibitors. This was followed by evaluation of ER stress response, NADPH oxidase expression/activity and the levels of phosphorylated vascular endothelial growth factor receptor-2 (p-VEGFR2) by western blotting and immunoprecipitation assays. Moreover, real-time imaging of intracellular calcium (Ca2+) release in HRECs treated with or without 15-HETE was performed using confocal microscopy.
Results
Deletion of 12/15-Lo significantly attenuated diabetes-induced ER stress in mouse retina. In vitro, 15-HETE upregulated ER stress markers such as phosphorylated RNA-dependent protein kinase-like ER-regulated kinase (p-PERK), activating transcription factor 6 (ATF6) and protein disulfide isomerase (PDI) in HRECs. Inhibition of ER stress reduced 15-HETE-induced-leucocyte adhesion, VEGFR2 phosphorylation and NADPH oxidase expression/activity. However, inhibition of NADPH oxidase or deletion of Nox2 had no effect on ER stress induced by the 12/15-LO-derived metabolites both in vitro and in vivo. We also found that 15-HETE increases the intracellular calcium in HRECs.
Conclusions/interpretation
ER stress contributes to 12/15-LO-induced retinal inflammation in diabetic retinopathy via activation of NADPH oxidase and VEGFR2. Perturbation of calcium homeostasis in the retina might also play a role in linking 12/15-LO to retinal ER stress and subsequent microvascular dysfunction in diabetic retinopathy.
Similar content being viewed by others
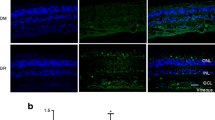
Avoid common mistakes on your manuscript.

Introduction
Diabetic retinopathy is the leading cause of vision loss in the working-age population worldwide [1]. It is characterised by an early inflammatory response that leads to disruption of the blood retinal barrier. This ultimately leads to diabetic macular oedema and retinal neovascularisation [2]. The mechanism of diabetic retinopathy has not yet been completely elucidated and the current therapies are limited by several side effects. Therefore, there is a need to investigate the underlying mechanism of diabetic retinopathy that could lead to identification of new therapeutic targets [3].
Upregulation of adhesion molecules and subsequent leucocyte adhesion to endothelial cells (leucostasis) play a crucial role in the pathogenesis of retinal hyperpermeability and neovascularisation in diabetic retinopathy [4, 5]. In addition to hyperglycaemia, dyslipidaemia and increased levels of bioactive lipids were considered major contributors to microvascular dysfunction seen in diabetic retinopathy [6]. 12/15 lipoxygenase (12/15-LO) is one of the lipoxygenase superfamily that incorporates two oxygen atoms at position 12 or 15 of arachidonic acid and related substrates, generating bioactive lipid metabolites [7]. Among these metabolites, 12- and 15-hydroxyeicosatetraenoic acids (HETEs) elicit pro-inflammatory and neutrophil chemotactic properties in various disease models [8, 9] including neuronal damage [10] and diabetic retinopathy [11]. Our previous studies showed significant increases in 12- and 15-HETE levels in the vitreous of individuals with diabetic retinopathy and in the retinas of Akita mice, a model of type 1 diabetes [12, 13]. In addition, we showed increased retinal permeability, inflammatory mediators such as intercellular adhesion molecule 1 (ICAM1) and vascular cell adhesion molecule 1 (VCAM1), and retinal neovascularisation in normal mice by intravitreal injection of 12-HETE [14]. In contrast, genetic deletion of 12/15-Lo (also known as Alox15) significantly reduced retinal permeability in diabetic mice and attenuated neovascularisation in the mouse model of oxygen-induced retinopathy [12, 15]. Consistent with our in vivo findings, inhibition of endothelial 12/15-LO in human retinal endothelial cells (HRECs) reduced high-glucose-induced leucostasis and ICAM-1 expression [14]. These findings indicate that 12/15-LO plays a causal role in diabetic retinopathy; however, the underpinning mechanism is yet to be determined.
Endoplasmic reticulum (ER) is the cell organelle responsible for proper protein folding, calcium homeostasis and lipid biosynthesis. Growing evidence suggests that ER stress acts as a major sensor that regulates cell energy metabolism, inflammation, redox status and cell survival under diabetic conditions [16,17,18,19,20]. Earlier studies have linked 12/15-LO signalling with the ER stress response [21]. There are three branches of transducer proteins that recognise ER stress, triggering a cellular response known as the unfolded protein response (UPR): RNA-dependent protein kinase-like ER-regulated kinase (PERK), inositol-requiring enzyme 1 (IRE1) and activating transcription factor (ATF) 6. Disturbance of any of the ER functions can trigger UPR. The first arm of UPR is the PERK pathway, which is initiated by PERK phosphorylation. Phospho-PERK inhibits phosphorylation of eukaryotic initiation factor 2 α (eIF2-α), which decreases the protein overload in the cell followed by the selective activation of ATF4. The second pathway of UPR includes IRE1, which homodimerises and trans-auto-phosphorylates; then, by using its endonuclease activity, it splices the mRNA of X-box binding protein 1 (XBP1) to form spliced XBP1 (XBP1s). ATF6 is the third signalling pathway in UPR. It is translocated to Golgi apparatus, where it is cleaved. Activation of UPR genes depends to a great extent on the duration of the insult and how long the cell is exposed to the stress. These pathways cause activation of transcription factors like ATF4, XBP1s or cleaved ATF6 to translocate to the nucleus, where they stimulate the transcription of UPR genes, such as calnexin and protein disulfide isomerase (PDI) [19,20,21].
Several reports showed that oxidative stress, as well as the perturbation of calcium homeostasis and elevated levels of cytosolic calcium, contribute to ER stress in different disease models [22, 23]. Previously, we have demonstrated NADPH oxidase (NOX) to be a major source of oxidative stress in retinas of diabetic rodents and have also demonstrated the existence of a crosstalk between 12/15-LO and the NOX system both in vitro and in vivo [13, 15, 24]. However, the interplay between ER stress, NOX and calcium homeostasis in 12/15-LO-mediated microvascular dysfunction during diabetic retinopathy remains unclear.
The goal of the current study was to investigate how 12/15-LO mediates microvascular dysfunction in diabetic retinopathy by portraying its causal relationship with ER stress and calcium homeostasis. Furthermore, we aimed to characterise the crosstalk between the two stress-response pathways—ER stress and NOX-mediated oxidative stress—in 12/15-LO-induced microvascular dysfunction using both genetic and pharmacological approaches.
Methods
Experimental animals
Animals were used in accordance with the Association for Research in Vision and Ophthalmology (ARVO) Statement for the Use of Animals in Ophthalmic and Vision Research and all procedures were approved by the Institutional Animal Care and Use Committee (IACUC) of Augusta University. We used 8-week-old male wild-type (WT), 12/15-Lo knockout mice (12/15-Lo−/−; B6.129S2-Alox15tm1Fun/J; stock no: 002778, Jackson Laboratories, Bar Harbor, ME, USA), or Nox2-deficient mice (Nox2−/−; B6.129S-Cybbtm1Din/J; stock No: 002365, Jackson Laboratories) on C57BL/6J background. Diabetes was induced by intraperitoneal injection of streptozotocin (STZ) (Sigma, St Louis, MO, USA) at 50 mg/kg for 3–5 days until the mice developed diabetes (random blood glucose ≥13.9 mmol/l). Intravitreal injections were performed in non-diabetic mice as previously described using 12-HETE (Cayman Chemical, Ann Arbor, MI, USA) [25, 26].
Culture of HRECs
HRECs were purchased from Cell Systems Corporation (Kirkland, WA, USA) and were grown at 37°C under 5% CO2 in Endothelial Basal Medium-2 (EBM-2) (Lonza, Walkersville, MD, USA) supplemented with 10% FBS (vol./vol.) (Atlantic Biological, Norcross, GA, USA) and 1% penicillin/streptomycin (vol./vol.) (Corning, Tewksbury, MA, USA). These cells were negative for mycoplasma and the majority were >95% positive for von Willebrand factor/factor VIII and CD31 (endothelial markers), whereas the contaminating astrocytes (stained with glial fibrillary acidic protein [GFAP]) and pericytes (stained with neural/glial antigen 2 [NG2]) were insignificant <1%. After HRECs reached 90% confluence, the medium was changed to be serum free, followed by different treatment with 15-HETE (0.1 μmol/l, Cayman Chemical) or vehicle (ethanol) in the presence or absence of 4-phenylbutyric acid (PBA, 30 μmol/l, Santa Cruz, Dallas, TX, USA), VAS2870 (10 μmol/l, Sigma), or apocynin (30 μmol/l, Calbiochem, La Jolla, CA, USA). Of note, species-specific divergence between orthologous LO isoforms has been reported for mouse 12-LO, which is arachidonate 15-LO in humans. This difference implies that caution should be taken if experimental data on LO activity are being translated from one species to others [27, 28]. Therefore, 12-HETE has been used in all mouse experiments while 15-HETE has been used in all human retinal endothelial cell culture experiments. The doses of 12- and 15-HETE were selected according to what was previously detected in the vitreous of individuals with diabetic retinopathy, 50 ng/ml (approximately 0.1–0.2 μmol/l) [12].
Western blot analysis and immunoprecipitation
Western blot analysis was conducted on retinal or cell lysates using antibodies for XBP1s, XBP1, p-PERK, PERK, p-eIF2α, eIF2α, ATF4, ATF6, PDI, gp91phox, P47phox, vascular endothelial growth factor receptor-2 (VEGFR2), β-actin and glyceraldehyde-3-phosphate dehydrogenase (GAPDH) (ESM Table 1) according to the previously described method [26]. To assess the phosphorylation of VEGFR2 in HRECs, immunoprecipitation was performed by the addition of an anti-VEGFR2 antibody and protein A agarose beads (Cell Signaling Technology, Beverly, MA, USA) per manufacturer’s instructions. This was followed by immunoblotting using anti-phosphotyrosine-PY20 antibody (BD Transduction Laboratories, San Diego, CA, USA).
Quantitative real-time RNA PCR
The mRNA levels of various ER stress markers were measured using TaqMan primers for Eif2a, Atf6, Chop (also known as Ddit3), Xbp1, Pdi (also known as Pdia3), calnexin and 18 s for mouse samples, or IRE1α, XBP1, PERK (also known as EIF2AK3), ATF4, ATF6, CHOP (also known as DDIT3), PDI (also known as PDIA2), BIP (also known as HSPA5) and calnexin for HREC samples (ESM Table 2) according to the previously described method [14].
ELISA
Vascular endothelial growth factor (VEGF) level in HREC conditioned media was estimated using VEGF ELISA kits (R&D Systems, Minneapolis, MN, USA) per the manufacturer’s instructions.
In vitro leucocyte adhesion assay
This assay was done according to our previous procedure [15] where adherent leucocytes were counted under an inverted fluorescence microscope (excitation/emission = 480/520 nm).
Superoxide measurement using dihydroethidium
HRECs were incubated with dihydroethidium (DHE) (20 μmol/l, Invitrogen, Eugene, OR, USA; catalogue no. D11347) in Earle’s balanced salt solution (EBSS) for 30 min. The medium was then replaced by EBSS containing NADPH (100 μmol/l) as an enzyme substrate for NOX followed by 15-HETE treatment in the presence or absence of VAS2870 (NADPH oxidase inhibitor, 10 μmol/l, Sigma) or PBA (30 μmol/l, Santa Cruz). A microplate reader (BioTek Instruments, Winooski, VT, USA) was used to detect the fluorescence intensity at different time intervals.
Live imaging of intracellular calcium
Intracellular calcium (Ca2+) mobilisations were detected by the use of Cal-520, AM (AAT Bioquest, Sunnyvale, CA, USA; catalogue no. 21130) according to manufacturer’s instructions. Measurements were performed using a Zeiss 780 Inverted Confocal microscope (Zeiss 780 Inverted Confocal, Imaging Core Facility, Augusta University) at (excitation/emission = 490/525 nm).
Statistical data analysis
The results are expressed as means ± SEM. Differences among different experimental groups were assessed by one-way ANOVA or two-tailed t test. Results were considered significant for p < 0.05.
Results
12/15-LO plays a causal role in diabetes-induced retinal endoplasmic reticulum stress
To explore the role of the 12/15-LO pathway in diabetes-induced retinal ER stress, we used two complementary approaches. The first approach was to test whether deletion of 12/15-Lo reduces retinal ER stress induced by diabetes. To achieve this, we induced diabetes in 12/15-Lo−/− mice and their matched congenic controls by STZ injection. After 12–14 weeks in diabetes, the animals were killed and retinas were collected to screen for ER stress markers by quantitative real-time RNA PCR (q-RT-PCR). Absence of the 12/15-Lo gene in the retina of 12/15-Lo−/− mice was confirmed by genotyping according to the protocol of the Jackson Laboratory (data not shown). Diabetes caused upregulation of the mRNA of many ER stress marker genes, including Xbp1 (p < 0.01), Eif2α (p = 0.06), Atf6, Pdi and Chop (p < 0.05) in retinas of diabetic WT mice compared with the non-diabetic littermates. These markers were significantly lower in retinas of diabetic 12/15-Lo−/− compared with diabetic WT mice, in particular, Xbp1 (p < 0.001), Atf6 (p < 0.05), Pdi (p < 0.01) and Chop (p < 0.01) (Fig. 1a).
Loss of 12/15-LO reduces diabetes-induced ER stress in mouse retina. (a) q-RT-PCR analysis of relative mRNA expression of ER stress markers in retinas of WT non-diabetic mice (white circles), WT diabetic mice (black squares), 12/15-Lo−/− non-diabetic mice (white triangles) and 12/15-Lo−/− diabetic mice (black triangles). Data represent individual data points with means ± SEM. (b) q-RT-PCR analysis of relative mRNA expression of ER stress markers in retinas of WT mice that received intravitreal injection of vehicle (white circles) or 12-HETE (0.1 μmol/l) (black squares). Data represent individual data points with means ± SEM. Representative western blots (c) and densitometry (d–k) of ER stress protein levels in retinas isolated from WT mice that received intravitreal injection of vehicle or 12-HETE (0.1 μmol/l). Data represent individual data points with means ± SEM; *p < 0.05, **p < 0.01, ***p < 0.001
Further evidence for the involvement of 12/15-LO in the induction of retinal ER stress was acquired from the second approach in which normal mice were injected intravitreally with the 12/15-LO-derived predominant murine metabolite 12-HETE. The effects of 12-HETE on retinal ER stress marker mRNA and proteins were examined by q-RT-PCR and western blot, respectively. Retinal levels of ER stress markers mRNA were upregulated 1 week after intravitreal injection of 12-HETE (0.1 μmol/l) including Eif2α (p < 0.01), calnexin (p < 0.05), Chop (p < 0.001) and Pdi (p = 0.06), compared with retinas of vehicle-injected animals (Fig. 1b). Moreover, western blot analysis revealed marked increases in p-PERK/PERK (Fig. 1c, g), PDI (Fig. 1c, j) and ATF4 (Fig. 1c, k) levels in retinas of 12-HETE-injected mice compared with the vehicle-injected controls, suggesting a causal relationship between 12/15-LO and ER stress induction in diabetic retinopathy.
12/15-LO-induced endothelial dysfunction is ER-stress-dependent
The aforementioned data provided a rationale to study in depth the direct relationship between 12/15-LO and ER stress in mediating endothelial dysfunction using HRECs. Initially, we sought to confirm whether 12/15-LO-derived bioactive lipids could induce ER stress in vitro in HRECs. For this purpose, HRECs were treated with 15-HETE (0.1 μmol/l) or vehicle and cell lysates were collected at different time points (1, 4 and 24 h). Western blot analysis showed an increase in the levels of PDI by 15-HETE starting after 1 h of treatment and reached a significant difference after 4 h (Fig. 2a, b). Concordantly, WB analyses for other ER stress markers (Fig. 2c) revealed that p-PERK/PERK (Fig. 2h) and ATF6 (Fig. 2j) showed significant increases in their levels compared with vehicle-treated cells. RT-PCR array of ER stress genes was utilised to assess UPR activation in HRECs treated with 15-HETE for 3 h. There was a significant upregulation of CHOP by 15-HETE compared with the vehicle-treated group (p < 0.05) (Fig. 2k).
15-HETE induces ER stress in HRECs. Representative western blots (a) and densitometry (b) for PDI protein level in HRECs treated with vehicle or 15-HETE (0.1 μmol/l) at different time points (0–24 h). Data represent individual data points with means ± SEM. Representative western blots (c) and densitometry (d-j) for ER stress proteins in HRECs treated with vehicle or 15-HETE (0.1 μmol/l) for 4 h. Data represent individual data points with means ± SEM. (k) q-RT-PCR of relative mRNA expression of ER stress markers in HRECs treated with vehicle (white circles) or 15-HETE (0.1 μmol/l; black squares) for 3 h. Data represent individual data points with means ± SEM; *p < 0.05, **p < 0.01 vs time 0 in (b), and as shown by brackets elsewhere
To functionally evaluate the impact of ER stress on 15-HETE-mediated endothelial dysfunction, we tested the effect of ER stress inhibition on 15-HETE-induced leucostasis in HRECs. Initial studies were conducted to confirm the contribution of ER stress in endothelial-leucocyte interaction using tunicamycin (Sigma), a known ER stress inducer. HRECs were treated with tunicamycin (5 μg/ml) for 24 h before adding fluorescently labelled human leucocytes for 90 min, followed by washing to remove the non-adherent leucocytes. As shown in Fig. 3, ER stress induction by tunicamycin led to a significant increase in the number of adherent leucocytes to the HRECs in comparison with the vehicle-treated cells (p < 0.001). To investigate the involvement of ER stress in 15-HETE-induced leucostasis, ER stress inhibitor (PBA, 30 μmol/l) was added to HRECs before 15-HETE treatment. PBA significantly reduced (p < 0.01) the number of adherent leucocytes induced by 15-HETE. At the concentration of PBA used, HREC viability was not affected (90 ± 6%) as assessed by (3-(4,5-dimethylthiazol-2-yl)-2,5-diphenyltetrazolium bromide) (MTT) assay, indicating that the decrease in the number of adherent leucocytes to 15-HETE-activated HRECs was due to the inhibition of ER stress, but not to cell death.
ER stress induction triggers endothelial leucocyte adhesion in HRECs, while ER stress inhibition abrogates 15-HETE-induced leucocyte adhesion. (a) In vitro leucocyte adhesion assay showing fluorescently labelled leucocytes adherent to HRECs under the influence of different treatments: control (vehicle), 15-HETE, lipopolysaccharide (LPS), tunicamycin (TM), VEGF, and 15-HETE in conjunction with the ER stress inhibitor PBA. (b) Quantification of the fold change of the mean number of the adherent leucocytes in each group showing a significant increase by the ER stress inducer tunicamycin, LPS, VEGF and 15-HETE compared with the vehicle-treated group. ER stress inhibition using PBA significantly reduced 15-HETE-induced endothelial leucocyte adhesion. Data represent individual data points with means ± SEM; *p < 0.05, **p < 0.01, ***p < 0.001; scale bar, 50 μm
Crosstalk between NADPH oxidase and ER stress
We previously reported that NADPH oxidase is implicated in 12/15-LO-induced retinal endothelial cell dysfunction [13, 15]. Here, the crosstalk between NADPH oxidase and ER stress in 12/15-LO-induced retinal microvascular dysfunction was also examined. HRECs were treated with 15-HETE with or without the NADPH oxidase inhibitor apocynin (30 μmol/l). The ER stress marker PDI was upregulated by 15-HETE in the presence or absence of apocynin (p = 0.008 vs control) (Fig. 4a, b). On the contrary, ER stress inhibition with PBA significantly reduced 15-HETE-induced expression of NOX2 (Fig. 4c, d) and P47phox (Fig. 4e, f), which are the catalytic and regulatory subunits of NADPH oxidase, respectively. Furthermore, 15-HETE significantly increased superoxide production after 15 min (p < 0.001) and 30 min (p < 0.01) vs control that declined at 45 min. ER stress inhibition by PBA significantly reduced 15-HETE-induced superoxide production (p < 0.05 vs 15-HETE treated HRECs) and this effect was comparable to the effect of NADPH oxidase inhibition (Fig. 4g).
Crosstalk between NADPH oxidase and ER stress in 15-HETE-treated HRECs. Representative western blots (a) and densitometry (b) for PDI protein level in HRECs treated for 24 h with vehicle (control), 15-HETE (0.1 μmol/l) or 15-HETE + NADPH oxidase inhibitor (apocynin, 30 μmol/l). Data represent individual data points with means ± SEM. Representative western blots and densitometry for NOX2 protein (c, d) or P47phox protein (e, f) in HRECs treated for 24 h with vehicle, 15-HETE (0.1 μmol/l) or 15-HETE + ER stress inhibitor (PBA, 30 μmol/l). Data represent individual data points with means ± SEM. (g) Superoxide measurement using DHE staining in HRECs treated with vehicle (white circles), 15-HETE (0.1 μmol/l) (black squares), 15-HETE (0.1 μmol/l) + VAS2870 (NADPH oxidase inhibitor, 10 μmol/l) (white triangles) or 15-HETE (0.1 μmol/l) + PBA (30 μmol/l) (black triangles). Data represent individual data points with means ± SEM
This proof of concept was then extended to be tested in vivo using Nox2−/− mice. Non-diabetic Nox2−/− mice were injected intravitreally with 12-HETE (0.1 μmol/l). One week after the injection, retinas were collected and both q-RT-PCR and western blotting were performed to screen the changes in retinal levels of ER stress genes and proteins, respectively. Intravitreal injection of 12-HETE in Nox2−/− mice induced increases in RNA levels of Xbp1 (p < 0.001), Eif2α (p < 0.05), Atf6 (p < 0.01), Pdi (p < 001), Chop (p < 0.05) and calnexin (p = 0.09) (Fig. 5a). Furthermore, 12-HETE significantly upregulated protein levels of retinal PDI (Fig. 5b, c) (p = 0.03 vs vehicle-injected) and ATF4 (Fig. 5b, d) (p = 0.02 vs vehicle-injected) in Nox2−/− mice and WT mice compared with the vehicle-injected group. Taken together, our in vivo and in vitro data suggest that NADPH oxidase does not have a regulatory role in 12/15-LO-mediated ER stress in the retina or retinal endothelial cells.
Nox2 deletion has no effect on 12-HETE-induced ER stress in vivo. (a) q-RT-PCR analysis of relative mRNA expression of ER stress markers in retinas isolated from Nox2−/− mice that had received intravitreal injection of vehicle (white circles) or 12-HETE (0.1 μmol/l) (black squares). Data represent individual data points with means ± SEM. Representative western blots (b) and densitometry of ER stress proteins PDI (c) and ATF4 (d) in retinas isolated from WT or Nox2−/− mice after intravitreal injection of 12-HETE (0.1 μmol/l). Data represent individual data points with means ± SEM. *p < 0.05, **p < 0.01, ***p < 0.001 vs vehicle in (c) and (d), or as shown by brackets in (a)
15-HETE induces intracellular calcium release
To find out a possible mechanism by which 12/15-LO induces ER stress, we were interested in testing whether 12/15-LO-derived lipid products dysregulate calcium homeostasis, a known regulator of ER stress in HRECs [23]. To this end, HRECs were loaded with a Ca2+-detecting dye followed by adding vehicle (ESM Video 1) or 15-HETE (0.1 μmol/l) (ESM Video 2). Real-time imaging showed a marked increase in the level of cytosolic calcium after adding 15-HETE compared with the control group. The ability of 15-HETE to induce intracellular calcium release may underscore a possible mechanism for 12/15-LO-induced ER stress (Fig. 6).
15-HETE induces intracellular Ca2+ release in HRECs. (a) Real-time imaging of intracellular calcium in HRECs treated with vehicle or 15-HETE (0.1 μmol/l). HRECs were loaded with a calcium-detecting dye, and then treated with vehicle or 15-HETE under the inverted confocal microscope. Treatment of HRECs with 15-HETE caused a significant increase in the fluorescence intensity, suggesting increased intracellular cytosolic Ca2+ levels. (b) Quantification of normalised calcium fluorescence in HRECs treated with vehicle (blue line) or 15-HETE (0.1 μmol/l) (red line) over 30 s from the beginning of the treatment. Data represent means ± SEM. (c) Statistical analysis of the averaged normalised fluorescence intensity of at least three independent experiments shows that 15-HETE treatment significantly increases HRECs’ intracellular cytosolic calcium levels compared with the vehicle-treated HRECs (*p < 0.05). Data represent individual data points with means ± SEM; scale bars, 200 μm
Inhibition of ER stress attenuates 15-HETE-induced VEGFR2 phosphorylation
We measured VEGF levels in the supernatant of HRECs treated with 15-HETE or vehicle. 15-HETE had no effect on VEGF release from HRECs when compared with that of the vehicle-treated group (n = 4, p > 0.05) (Fig. 7a). We previously showed that inhibition of NADPH oxidase reduced VEGFR2 phosphorylation in retinal endothelial cells treated with 12/15-LO-derived metabolites [13]. To investigate whether ER stress inhibition elicits a similar effect, HRECs were treated with 15-HETE for 5 min in the presence or absence of PBA (30 μmol/l), then immunoprecipitation was used to detect VEGFR2 phosphorylation (Fig. 7b). 15-HETE induced VEGFR2-phosphorylation (p < 0.05), which was significantly reduced by the ER stress inhibitor (PBA) and the NADPH oxidase inhibitor (apocynin) (n = 4, p < 0.05) (Fig. 7c). These data suggest that ER stress could play a role in 12/15-LO-induced retinal microvascular complications via activation of VEGFR2 signalling.
ER stress inhibition attenuates 15-HETE-induced VEGFR2 phosphorylation. (a) ELISA of VEGF levels in supernatant of HRECs treated with vehicle or 15-HETE for 24 h showing no significant difference in the VEGF levels. Data represent individual data points with means ± SEM. (b) Immunoprecipitation (IP) of VEGFR2 in HRECs treated with 15-HETE in the presence or absence of PBA or apocynin for 5 min, followed by immunoblotting (IB) of phosphotyrosine (pTyr). Normalisation was done by re-blotting the membrane for VEGFR2. (c) Densitometry analysis shows a significant increase in VEGFR2 phosphorylation by 15-HETE compared with the control. ER stress inhibitor (PBA) and NADPH oxidase inhibitor (apocynin) reduced the effect of 15-HETE on VEGFR2 phosphorylation. Data represent individual data points with means ± SEM; *p < 0.05 vs vehicle
Discussion
Our previous studies established 12/15-LO as a potential player in the development of microvascular dysfunction in diabetic retinopathy via activating NADPH oxidase and VEGFR2 signalling [13, 15]. The current study explored the role of ER stress in mediating the effect of 12/15-LO lipid metabolites on retinal endothelial dysfunction during diabetic retinopathy. Our major findings include: (1) deletion of 12/15-Lo significantly attenuated the diabetes-induced ER stress in mouse retina; (2) 15-HETE, the major product of 12/15-LO in humans, induced ER stress in HRECs; (3) inhibition of ER stress reduced 15-HETE-induced leucocyte adhesion; (4) inhibition of NADPH oxidase or deletion of its catalytic subunit NOX2 did not reduce 12/15-LO-induced ER stress; (5) inhibition of ER stress reduced NADPH oxidase activity and expression of its catalytic and regulatory subunits NOX2 and P47phox, respectively; (6) 15-HETE induced an increase in intracellular calcium levels in HRECs; (7) inhibition of ER stress reduced 15-HETE-induced VEGFR2 phosphorylation.
Accumulated evidence demonstrated that ER stress is a crucial player in the development of retinal microvascular dysfunction in diabetic retinopathy [19, 20]. However, the implication of ER stress in 12/15-LO-mediated retinal endothelial cell activation has not been investigated yet. The current study portrays a mechanistic axis that links hyperglycaemia to retinal endothelial cell dysfunction. This axis starts from hyperglycaemia-induced activation of 12/15-LO, followed by ER stress and NADPH oxidase activation, and ends with increased VEGFR2 phosphorylation. Consistent with previous reports, our in vivo experiments showed that diabetes induces ER stress in mouse retina [16]. Our RNA data from the 12/15-Lo−/− mice showed a significant reduction in ER stress markers in diabetic 12/15-Lo−/− retina vs diabetic WT mouse retina. Moreover, intravitreal injection of 12-HETE, the main bioactive lipid product of 12/15-LO in rodents, into the eyes of non-diabetic WT mice activated retinal ER stress, suggesting ER stress as an important player in mediating the pro-inflammatory and permeability effects of 12/15-LO in the retina in diabetes. Although our diabetes model was type 1, another study utilised 12/15-Lo−/− mice placed on a high-fat diet (as a model for pre-type-2 diabetes) [21] showed a significant reduction in ER stress markers as well, compared with WT mice fed a normal diet. Interestingly, ER stress markers that changed with a high-fat diet were different among different tissues, indicating the complexity and variability of the ER stress response across different tissues.
We also used an in vitro model utilising HRECs treated with 15-HETE and we detected activation of the ER stress response by 15-HETE. Lipoxygenases catalyse oxygen insertion into polyunsaturated fatty acids. There are different LO analogues such as 5-LO, 12-LO and 15-LO. The different numbers indicate the carbon at which the oxygen is added. Interestingly, a previous study reported that deletion of 5- or 12/15-Lo dampens diabetes-induced leucostasis after 3 months of diabetes. However, 5-Lo deletion only reduced capillary degeneration after 9 months of diabetes, suggesting a differential role for each enzyme at different stages of diabetic retinopathy [11]. Our data showed that ER stress inhibition significantly reduced 15-HETE-induced leucostasis in HRECs, suggesting the early involvement of ER stress in the 12/15-LO-induced inflammatory reaction.
Others have reported the presence of interaction between ER and oxidative stresses in endothelial cell dysfunction. Recently, mRNA-sequencing data showed ER stress-induced downregulation of endogenous antioxidant genes [29]. Also, ER stress induces endothelial dysfunction via NADPH oxidase. This notion has been supported by studies done both in vitro utilising primary coronary endothelial cells and in vivo using p47phox−/− mice injected with tunicamycin [30]. We previously reported that 12/15-LO induced retinal microvascular complications in an NADPH-oxidase-dependent mechanism [13, 15]. In the current study, we looked at the relationship between ER stress and NADPH oxidase in retinal endothelial cell dysfunction induced by 12/15-LO-derived metabolites. 12/15-LO-derived bioactive lipids induced ER stress despite the deletion or inhibition of NADPH oxidase, suggesting that either ER stress and NADPH oxidase are working independently, or ER stress is an upstream effector of NADPH oxidase. To uncover which of these two possibilities is involved, we measured superoxide production and the expression of NADPH oxidase subunits, NOX2 and P47phox in HRECs after 15-HETE treatment in the presence or absence of a ER stress inhibitor. ER stress inhibition significantly reduced 15-HETE-induced superoxide production and expression of NOX2, and P47phox in HRECs subjected to 15-HETE treatment. These findings suggest that ER stress is an upstream effector of NADPH oxidase in the signalling pathway induced by activated retinal 12/15-LO in diabetes.
PDI has been suggested as an organiser of NADPH oxidase activation in leucocytes [31], vascular smooth muscles and endothelial cells [32, 33]. 12/15-LO-derived lipid metabolites increased levels of PDI in HRECs and mouse retina, which was not abrogated by either inhibition or deletion of NOX2. Moreover, diabetes-induced PDI upregulation was reduced in diabetic 12/15-LO−/− mouse retina. Interestingly, it was shown that PDI is associated with NADPH oxidase and acts as a redox-dependent enzyme complex regulator with a supportive effect on the activity of NADPH oxidase [31]. Thus, the ability of 12/15-LO-derived bioactive lipids to upregulate PDI both in vitro and in vivo suggests a mechanistic link between 12/15-LO-induced ER stress and NADPH oxidase activation during diabetic retinopathy.
It was reported that disruption of both lipid metabolism and calcium homeostasis causes activation of ER stress in the liver [23]. Moreover, thapsigargin, a widely used drug for ER stress induction, is an inhibitor of the sarco/endoplasmic reticulum Ca2+ ATPase (SERCA), leading to elevated intracellular cytosolic Ca2+ levels and induction of ER stress [34]. In obesity, it was reported that lipids inhibit SERCA and cause disturbance of Ca2+ homeostasis with subsequent activation of ER stress [23], which might be a possible mechanism of 12/15-LO-induced ER stress. In addition, the role of NOX2 in ER stress-oxidative stress interplay is Ca2+ dependent [35, 36].
We reported in this study the 12/15-LO-induced upregulation of ATF4. Interestingly, ATF4 is not only involved in ER stress-induced oxidative stress [29], but in addition, ATF4 binds to the promoter of human VEGF and upregulates both VEGF and cytokine production in retinas of diabetic rodents. Recently, ATF4 was reported to have a critical role in the regulation of the inflammatory response in brain and retinal endothelial cells [18, 37, 38]. The role of ER stress in diabetic retinopathy has been also linked to activation of VEGF signalling [17, 38, 39]. The link between ER stress and VEGF is supported by other reports that ER stress inhibition reduces VEGF expression in retinas of diabetic mice [16]. On the other hand, VEGF activates ER stress signalling in endothelial cells independent of the accumulation of misfolded proteins within the ER lumen [40], suggesting that both ER stress and VEGF have positive feedback. Taking together the accumulated evidence of ER stress–VEGF crosstalk, and our previous study [13], which demonstrated activation of VEGFR2 in retinal endothelial cells by 12/15-LO-derived metabolites, we examined whether ER stress is involved in activating VEGF signalling by 12/15-LO metabolites. Consistent with our previous report [13], our data showed that, while 15-HETE has no effect on VEGF expression in HRECs, it significantly activated VEGFR2. This effect was significantly attenuated by PBA and apocynin, the ER stress and NADPH oxidase inhibitors, respectively. These data support the potential role of both ER stress and NADPH oxidase in mediating the effect of 15-HETE on retinal endothelial function via activating VEGF signalling.
The ubiquitin–proteasome system (UPS) is an important member of the protein quality control machinery [41, 42]. UPS dysfunction is implicated in ER stress-induced apoptosis [43], oxidative stress induction [44, 45], disturbed calcium homeostasis [46] and retinal diseases including diabetic retinopathy [47]. Recently, defective UPS was reported to cause ER stress in diabetic retina [48]. However, other studies showed that ER stress itself can cause defective UPS [49]. The discrepancy noticed in RNA and protein levels of ER stress markers can be explained in part through disturbed UPS. Studying the impact of 12/15-LO on UPS may provide new insights into the mechanism of 12/15-LO-induced ER stress.
In summary, our previous and current data suggest that 12/15-LO is implicated in retinal microvascular dysfunction in diabetic retinopathy via activation of the ER stress/NADPH oxidase/VEGFR2 signalling pathway, and that disruption of Ca2+ homeostasis could be an essential step in initiating this signalling pathway (Fig. 8). Thus, the 12/15-LO/ER stress signalling loop may represent an early player that could be targeted to prevent progression of microvascular dysfunction in diabetic retinopathy.
A schematic summary. Hyperglycaemia increases the activity of 12/15-LO enzyme with a subsequent increase of its bioactive lipid products, 12- and 15-HETE, in retinal endothelial cells. 12/15-LO lipid metabolites increase endothelial intracellular calcium levels that may activate ER stress and, in turn, NADPH oxidase. These intracellular activated pathways lead to increased phosphorylation of VEGFR2 and subsequent retinal endothelial dysfunction
Data availability
All the data are available on request from the authors.
Abbreviations
- ATF:
-
Activating transcription factor
- DHE:
-
Dihydroethidium
- eIF2α:
-
Eukaryotic initiation factor 2α
- ER:
-
Endoplasmic reticulum
- GAPDH:
-
Glyceraldehyde-3-phosphate dehydrogenase
- HETE:
-
Hydroxyeicosatetraenoic acid
- HREC:
-
Human retinal endothelial cell
- IRE1:
-
Inositol-requiring enzyme 1
- LO:
-
Lipoxygenase
- NOX:
-
NADPH oxidase
- PBA:
-
4-Phenylbutyric acid
- PDI:
-
Protein disulfide isomerase
- PERK:
-
RNA-dependent protein kinase-like ER-regulated kinase
- q-RT-PCR:
-
Quantitative real-time PCR
- STZ:
-
Streptozotocin
- UPR:
-
Unfolded protein response
- UPS:
-
Ubiquitin–proteasome system
- VEGF:
-
Vascular endothelial growth factor
- VEGFR:
-
VEGF receptor
- WT:
-
Wild-type
- XBP1:
-
X-box binding protein 1
References
Congdon NG, Friedman DS, Lietman T (2003) Important causes of visual impairment in the world today. JAMA 290:2057–2060
Leal EC, Manivannan A, Hosoya K et al (2007) Inducible nitric oxide synthase isoform is a key mediator of leukostasis and blood-retinal barrier breakdown in diabetic retinopathy. Invest Ophthalmol Vis Sci 48:5257–5265
Cheung N, Mitchell P, Wong TY (2010) Diabetic retinopathy. Lancet 376:124–136
Miyamoto K, Khosrof S, Bursell SE et al (1999) Prevention of leukostasis and vascular leakage in streptozotocin-induced diabetic retinopathy via intercellular adhesion molecule-1 inhibition. Proc Natl Acad Sci U S A 96:10836–10841
Bai N, Tang S, Ma J, Luo Y, Lin S (2003) Increased expression of intercellular adhesion molecule-1, vascular cellular adhesion molecule-1 and leukocyte common antigen in diabetic rat retina. Yan Ke Xue Bao 19:176–183
Lupo G, Motta C, Giurdanella G et al (2013) Role of phospholipases A2 in diabetic retinopathy: in vitro and in vivo studies. Biochem Pharmacol 86:1603–1613
Horn T, Adel S, Schumann R et al (2015) Evolutionary aspects of lipoxygenases and genetic diversity of human leukotriene signaling. Prog Lipid Res 57:13–39
Li J, Rao J, Liu Y et al (2013) 15-Lipoxygenase promotes chronic hypoxia-induced pulmonary artery inflammation via positive interaction with nuclear factor-kappaB. Arterioscler Thromb Vasc Biol 33:971–979
Kuhn H, O'Donnell VB (2006) Inflammation and immune regulation by 12/15-lipoxygenases. Prog Lipid Res 45:334–356
Pallast S, Arai K, Pekcec A et al (2010) Increased nuclear apoptosis-inducing factor after transient focal ischemia: a 12/15-lipoxygenase-dependent organelle damage pathway. J Cereb Blood Flow Metab 30:1157–1167
Gubitosi-Klug RA, Talahalli R, Du Y, Nadler JL, Kern TS (2008) 5-Lipoxygenase, but not 12/15-lipoxygenase, contributes to degeneration of retinal capillaries in a mouse model of diabetic retinopathy. Diabetes 57:1387–1393
Al-Shabrawey M, Mussell R, Kahook K et al (2011) Increased expression and activity of 12-lipoxygenase in oxygen-induced ischemic retinopathy and proliferative diabetic retinopathy: implications in retinal neovascularization. Diabetes 60:614–624
Othman A, Ahmad S, Megyerdi S et al (2013) 12/15-Lipoxygenase-derived lipid metabolites induce retinal endothelial cell barrier dysfunction: contribution of NADPH oxidase. PLoS One 8:e57254
Ibrahim AS, Saleh H, El-Shafey M et al (2017) Targeting of 12/15-lipoxygenase in retinal endothelial cells, but not in monocytes/macrophages, attenuates high glucose-induced retinal leukostasis. Biochim Biophys Acta 1862:636–645
Ibrahim AS, Elshafey S, Sellak H et al (2015) A lipidomic screen of hyperglycemia-treated HRECs links 12/15-lipoxygenase to microvascular dysfunction during diabetic retinopathy via NADPH oxidase. J Lipid Res 56:599–611
Li J, Wang JJ, Yu Q, Wang M, Zhang SX (2009) Endoplasmic reticulum stress is implicated in retinal inflammation and diabetic retinopathy. FEBS Lett 583:1521–1527
Zhong Y, Li J, Chen Y, Wang JJ, Ratan R, Zhang SX (2012) Activation of endoplasmic reticulum stress by hyperglycemia is essential for Müller cell-derived inflammatory cytokine production in diabetes. Diabetes 61:492–504
Wang Y, Gao S, Zhu Y, Shen X (2017) Elevated activating transcription factor 4 and glucose-regulated 78 Kda protein levels correlate with inflammatory cytokines in the aqueous humor and vitreous of proliferative diabetic retinopathy. Curr Eye Res: 1–7
Ma JH, Wang JJ, Zhang SX (2014) The unfolded protein response and diabetic retinopathy. J Diabetes Res 2014:160140
Zhang SX, Ma JH, Bhatta M, Fliesler SJ, Wang JJ (2015) The unfolded protein response in retinal vascular diseases: implications and therapeutic potential beyond protein folding. Prog Retin Eye Res 45:111–131
Cole BK, Kuhn NS, Green-Mitchell SM et al (2012) 12/15-Lipoxygenase signaling in the endoplasmic reticulum stress response. Am J Physiol Endocrinol Metab 302:E654–E665
Cao SS, Kaufman RJ (2014) Endoplasmic reticulum stress and oxidative stress in cell fate decision and human disease. Antioxid Redox Signal 21:396–413
Fu S, Yang L, Li P et al (2011) Aberrant lipid metabolism disrupts calcium homeostasis causing liver endoplasmic reticulum stress in obesity. Nature 473:528–531
Al-Shabrawey M, Bartoli M, El-Remessy AB et al (2008) Role of NADPH oxidase and Stat3 in statin-mediated protection against diabetic retinopathy. Invest Ophthalmol Vis Sci 49:3231–3238
Ibrahim AS, El-Remessy AB, Matragoon S et al (2011) Retinal microglial activation and inflammation induced by amadori-glycated albumin in a rat model of diabetes. Diabetes 60:1122–1133
Ibrahim AS, Tawfik AM, Hussein KA et al (2015) Pigment epithelium-derived factor inhibits retinal microvascular dysfunction induced by 12/15-lipoxygenase-derived eicosanoids. Biochim Biophys Acta 1851:290–298
Funk CD, Chen XS, Johnson EN, Zhao L (2002) Lipoxygenase genes and their targeted disruption. Prostaglandins Other Lipid Mediat 68–69: 303–312
Kuhn H (2004) Lipoxygenases in the cardiovascular system. Circ Res 94:1527–1529
Han J, Back SH, Hur J et al (2013) ER-stress-induced transcriptional regulation increases protein synthesis leading to cell death. Nat Cell Biol 15:481–490
Galan M, Kassan M, Kadowitz PJ, Trebak M, Belmadani S, Matrougui K (2014) Mechanism of endoplasmic reticulum stress-induced vascular endothelial dysfunction. Biochim Biophys Acta 1843:1063–1075
de Paes AAM, Verissimo-Filho S, Guimaraes LL et al (2011) Protein disulfide isomerase redox-dependent association with p47(phox): evidence for an organizer role in leukocyte NADPH oxidase activation. J Leukoc Biol 90:799–810
Laurindo FR, Fernandes DC, Amanso AM, Lopes LR, Santos CX (2008) Novel role of protein disulfide isomerase in the regulation of NADPH oxidase activity: pathophysiological implications in vascular diseases. Antioxid Redox Signal 10:1101–1113
Janiszewski M, Lopes LR, Carmo AO et al (2005) Regulation of NAD(P)H oxidase by associated protein disulfide isomerase in vascular smooth muscle cells. J Biol Chem 280:40813–40819
Rogers TB, Inesi G, Wade R, Lederer WJ (1995) Use of thapsigargin to study Ca2+ homeostasis in cardiac cells. Biosci Rep 15:341–349
Li G, Scull C, Ozcan L, Tabas I (2010) NADPH oxidase links endoplasmic reticulum stress, oxidative stress, and PKR activation to induce apoptosis. J Cell Biol 191:1113–1125
Santos CX, Tanaka LY, Wosniak J, Laurindo FR (2009) Mechanisms and implications of reactive oxygen species generation during the unfolded protein response: roles of endoplasmic reticulum oxidoreductases, mitochondrial electron transport, and NADPH oxidase. Antioxid Redox Signal 11:2409–2427
Huang H, Jing G, Wang JJ, Sheibani N, Zhang SX (2015) ATF4 is a novel regulator of MCP-1 in microvascular endothelial cells. J Inflamm 12:31
Chen Y, Wang JJ, Li J et al (2012) Activating transcription factor 4 mediates hyperglycaemia-induced endothelial inflammation and retinal vascular leakage through activation of STAT3 in a mouse model of type 1 diabetes. Diabetologia 55:2533–2545
Zhong Y, Wang JJ, Zhang SX (2012) Intermittent but not constant high glucose induces ER stress and inflammation in human retinal pericytes. Adv Exp Med Biol 723:285–292
Karali E, Bellou S, Stellas D, Klinakis A, Murphy C, Fotsis T (2014) VEGF signals through ATF6 and PERK to promote endothelial cell survival and angiogenesis in the absence of ER stress. Mol Cell 54:559–572
Shang F, Taylor A (2004) Function of the ubiquitin proteolytic pathway in the eye. Exp Eye Res 78:1–14
Ye Y (2005) The role of the ubiquitin-proteasome system in ER quality control. Essays Biochem 41:99–112
Casas S, Gomis R, Gribble FM, Altirriba J, Knuutila S, Novials A (2007) Impairment of the ubiquitin-proteasome pathway is a downstream endoplasmic reticulum stress response induced by extracellular human islet amyloid polypeptide and contributes to pancreatic beta-cell apoptosis. Diabetes 56:2284–2294
Ishii T, Sakurai T, Usami H, Uchida K (2005) Oxidative modification of proteasome: identification of an oxidation-sensitive subunit in 26 S proteasome. Biochemistry 44:13893–13901
Shang F, Taylor A (2012) Roles for the ubiquitin-proteasome pathway in protein quality control and signaling in the retina: implications in the pathogenesis of age-related macular degeneration. Mol Asp Med 33:446–466
Liu K, Lyu L, Chin D et al (2015) Altered ubiquitin causes perturbed calcium homeostasis, hyperactivation of calpain, dysregulated differentiation, and cataract. Proc Natl Acad Sci U S A 112:1071–1076
Campello L, Esteve-Rudd J, Cuenca N, Martin-Nieto J (2013) The ubiquitin-proteasome system in retinal health and disease. Mol Neurobiol 47:790–810
Shruthi K, Reddy SS, Reddy GB (2017) Ubiquitin-proteasome system and ER stress in the retina of diabetic rats. Arch Biochem Biophys 627:10–20
Menendez-Benito V, Verhoef LG, Masucci MG, Dantuma NP (2005) Endoplasmic reticulum stress compromises the ubiquitin-proteasome system. Hum Mol Genet 14:2787–2799
Acknowledgements
Authors would like to thank M. E. McGee-Lawrence (Department of Cellular Biology and Anatomy, Medical College of Georgia, Augusta University) for assistance with live calcium imaging.
Contribution statement
KE, ASI, NE and MA conceived and designed the study; KE, ASI, HS, NE, SE and KH contributed to data acquisition; KE, ASI, NE and SE analysed the data; all authors interpreted the data, drafted the article, revised it and approved the final version. MA is the guarantor of this work.
Funding
This study was funded by the National Eye Institute (National Institutes of Health) Grant 5R01EY023315 (MA) and Egyptian Cultural and Educational Bureau – Washington DC (Egyptian Ministry of Higher Education) (KE).
Author information
Authors and Affiliations
Corresponding author
Ethics declarations
The authors declare that there is no duality of interest associated with this manuscript.
Electronic supplementary material
Rights and permissions
About this article
Cite this article
Elmasry, K., Ibrahim, A.S., Saleh, H. et al. Role of endoplasmic reticulum stress in 12/15-lipoxygenase-induced retinal microvascular dysfunction in a mouse model of diabetic retinopathy. Diabetologia 61, 1220–1232 (2018). https://doi.org/10.1007/s00125-018-4560-z
Received:
Accepted:
Published:
Issue Date:
DOI: https://doi.org/10.1007/s00125-018-4560-z