Abstract
Some hot springs located in west of Turkey were investigated with respect to presence of thermophilic microorganisms. Based on the 16S rRNA gene sequence analysis, the 7 isolates are members of the genus Geobacillus, the other 7 isolates are members of the genus Brevibacillus. Based on recN sequence similarity analysis, isolate EG1 is a strain of G. thermodenitficans and isolate SG1 is a strain of one of Group 3 species. The species identity for the isolates SG13, SG16, SG17, EG6, and HIG12 are uncertain. Based on hypervariable regions similarity analysis of the 16S rDNA, isolates HIG17, HIG27 and HIG29 are strains of B. thermoruber, B. parabrevis and B. borstelensis, respectively. Isolate HIG22 and HIG28 are strains of B. agri, while HIG11 is a strain of B. gelatini. Also HIG19 could be regarded as an independent species Brevibacillus. The presence of xylanase and glucose isomerase activities, their optimum temperature and pH were also investigated. These results showed that SG16 has both xylanase and glucose isomerase activity, 4 strains have only xylanase activity, 4 strains have only glucose isomerase activity, remaining 6 strains have neither of these activities. The isolate SG16 has the highest temperature optima (80°C), HIG11, HIG19 and SG16 isolates have the highest pH optima (pH 9) of xylanase. Isolate SG16, SG1 and HIG12 have optimum glucose isomerase activities at 80°C, and isolates SG16, SG17 and HIG12 have the lowest pH of 6.5. Therefore, the results of the study indicated that these bacteria and their enzymes can constitute important reservoirs for biotechnological researches.
Similar content being viewed by others
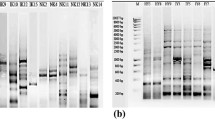
Avoid common mistakes on your manuscript.
INTRODUCTION
The taxonomy of thermophilic endospore-forming bacteria—and especially their identification—has generated considerable interest in the last decade. The importance of these bacteria has increased due to their potential as a source of thermostable enzymes, including xylanases, proteases, amylases, pullulanases, peroxidases, glucose isomerases, lipases, and DNA restriction enzymes (Ovando-Chacon et al., 2020; Taha et al., 2020).
Recently, interest in xylanase and glucose isomerase has increased significantly due to the potential applications in pulping and bleaching processes, in the food and feed industry, textile processes, the production ethanol from hemicellulose, the enzymatic saccharification of lignocellulosic materials, and waste treatment (Tsotetsi et al., 2020; Nam, 2022). Most of these processes are carried out at high temperatures, so that thermostable enzymes would give a great advantage (Mohammad et al., 2017).
Glucose isomerase is gaining importance in the food industry by catalyzing the reaction that converts glucose into fructose in the production of high fructose corn syrup (HFCS) (Jin et al., 2017; Rengasamy et al., 2020). Besides, glucose isomerase has received increased attention by industries for its potential application in the production of ethanol from hemicelluloses (Chanitnun and Pinphanichakarn, 2012). Given the high consumption of HFCS in beverages, many nutrition researchers have focused on the possible relationship between HFCS sweetened beverage consumption and obesity, diabetes or other related chronic diseases (Sinir et al., 2017). The major uses of HFCS are in the beverage, baking, canning and confectionery industries (Zargaraan et al., 2016). In the HFCS process, the final concentration of the fructose: glucose equilibrium is highly temperature dependent, favoring fructose at higher temperatures. Also, commercial application of glucose isomerase demands an acidic pH optimum to enable starch liquefaction and glucose isomerization to be carried out in a single step. Thus, attempts have been made to find novel microorganisms that can produce thermostable and acidic active glucose isomerase in order to increase the maximum attainable fructose level (Abdel-rassol et al., 2012; Neifar et al., 2019).
The use of xylanases is becoming increasingly important in different industrial processes. One major area is in the pulp and paper industry where xylanase can be used for the bleaching of kraft pulps (Canakci et al., 2007; Lyytikäinen and Backfolk, 2017). The use of xylanase in this industry is related to reduction of environmental pollution caused by the use of large amounts of chlorine and chlorinated compounds during pulp bleaching. Since most of processes in the paper and pulp industry are carried out at high temperature and at alkali pH, the use of alkaline xyalanases with higher temperature optima is considered to be advantageous, making the use of thermostable alkaline xylanases very attractive (Kacagan et al., 2008; Enez and Fincan, 2016).
Consequently, the use of thermostable alkaline xylanases and thermostable acidic active glucose isomerases is economically and technically very attractive. However, there are relatively few microorganisms that produce xylanases that are optimally active at elevated temperature or alkaline conditions (Mamo et al., 2006; Bhalla et al., 2015). Also glucose isomerases, which are optimally active and stable at acidic pH and at elevated temperature, are infrequent (Pedersen, 1993; Jin et al., 2021). Therefore, it is very important to isolate new microorganisms capable of producing acidic active thermostable glucose isomerase and alkali active thermostable xylanase (Inan et al., 2011a). Over the past decade, there has been a significant increase in interest in thermophilic endospore forming bacteria of the genus Bacillus, both because of their possible contamination of heated food products and because of their biotechnological importance as sources of thermostable enzymes and other products of industrial interest (Bergquist and Morgan, 1992; Zhang et al., 2020). Thus, thermophilic organisms are of special interest as a source of novel thermostable enzymes (Touzel et al., 2000; Suleiman et al., 2020). Thus, studying phylogenetic relations and diversity in the thermophilic organisms is not only a taxonomic concern, but also a necessity to fully exploit their biotechnological potential.
16S rRNA analyses are useful for comparing phenotypically close and still genetically different microorganisms and it is also known that analysis of 16S rRNA sequences may be insufficient to distinguish between some species (Vandamme et al., 1996; Inan et al., 2012). Consequently, systematics has come to rely increasingly on the comparison of DNA sequences, in particular 16S rRNA gene sequences, as a supplementary or alternative approach (Stackebrandt et al., 2002; Inan et al., 2011b). Recently, Zeigler (2005) identified over thirty genes that can be used in bacterial systematics and DNA repair and genetic recombination protein (recN) gene is one of the strong candidates among them for genome similarity prediction. The recN sequence identity scores can predict the whole genome sequence identity shared by two organisms to a high degree of accuracy. For the species studied, genome identity scores estimated by recN analysis showed a mean difference of only 4.4% in genomic alignments from those measured directly. For the genus Geobacillus, recN was clearly superior to 16S rRNA gene, with nearly an order of magnitude greater resolving power at the species-subspecies level. Therefore recN meets the requirements as a useful tool for assigning isolates to species within this genus.
Brevibacillus species give their poor reactivity in conventional identification tests so that it has been difficult to distinguish between species of the Brevibacillus genus by using these tests (Logan et al., 2002; Goto et al., 2004; Inan et al., 2016). However, more recently, sequencing of the 5' end hypervariable regions (HV) of the 16S rDNA is a useful marker for rapid and convenient identification and grouping of Brevibacillus species (Allan et al., 2005). In members of the genus Brevibacillus, the HV region sequence is highly conserved within a species and has diverged sufficiently different between species, enabling identification and grouping of Brevibacillus species by sequence comparisons of the HV region, as it has shown for the genera Bacillus, Paenibacillus, and Alicyclobacillus (Goto et al., 2000, 2002a, 2002b, 2004).
In this study, a molecular identification study was carried out on some thermophilic Geobacillus and Brevibacillus strains isolated from Kaynarca Hot Spring (İzmir), Karakoc Hot Spring (İzmir), Omerbeyli Hot Spring (Aydin), Camkoy Camur Hot Spring (Aydin), and Alangullu Hot Spring (Aydin) in Turkey. Thermophilic xylanase and glucose isomerase activity of these strains were investigated.
MATERIALS AND METHODS
Sampling and Isolation
In this study, thermophilic bacteria were isolated from mud and water samples taken from Izmir Kaynarca Hot Spring, Izmir Karakoc Hot Spring, Aydin Camkoy Camur Hot Spring, Aydin Omerbeyli Hot Spring and Aydin Alangullu Hot Spring in Turkey. The water temperature of these hot springs is between 70–130°C. The mud and water samples taken from of the hot springs were brought to laboratory under convenient conditions at 4°C and these samples were immediately used for enrichment in Luria-Bertani (LB) medium at 55°C. One-day-old enrichment cultures were repeatedly subcultured in 10 ml on Luria-Bertani liquid medium and streaked on agar plates to obtain separate colonies. The method used for isolation has been described previously (Inan et al., 2015). The purity of the isolates was assessed by using colony morphology and microscopy.
Phenotypic Features of Isolates
All phenotypic tests were made in duplicate and repeated if inconsistent results were first observed. The colony morphology of isolates was observed under a light microscope as described by Savas et al. (2009). The cell morphology and motility were examined by the light microscopy of native preparations. Gram staining was carried out by using the standard Gram’s reaction (Murray et al., 1994) and was confirmed by a nonstaining procedure using the KOH lysis test method (Gregersen, 1978). The formation of spores was tested by microscopic observations at different incubation periods, both in liquid cultures and in single colonies of isolates.
The presence of catalase and oxidase were investigated according to the methods described by Harley and Prescott (2002). The need of bacterial isolates for atmospheric O2 was detected after they were incubated in Brain Heart Infusion Agar media. Temperature and pH range for growth were determined following incubation of the strains on LB at different temperatures (30–80°C) and pH (5.0–10.0) with 5°C and one unit intervals, respectively. The NaCl requirement for growth was also tested in the LB medium containing 1.0–8.0% (w/v) NaCl. Growth was determined by visual observation after three days of incubation (Munster et al., 1986).
Biochemical characteristics were screened by API 20E® strips system (bioMérieux, France) according to the manufacturer’s instructions with some modifications. The number of bacteria was adjusted to one McFarland standard, and 200 μL of suspension was transferred into each well. Strips in incubation boxes were put into a plastic container filled into the bottom with sterilized water to minimize evaporation and then incubated at 55°C. The API strips were observed at 4, 8, 16, and 24 h. At each observation point, water was replenished when necessary.
PCR Amplification and DNA Sequencing
PCR amplification and cloning of 16S rRNA and recN gene sequences. The 16S rRNA genes were selectively amplified from purified genomic DNA by using oligonucleotide primers designed to anneal to conserved positions in the 5' and 3' regions of bacterial 16S rRNA genes. The forward primer, UNI16S-L (5'‑ATTCTAGAGTTTGATCATGGCTTCA), corresponded to positions 11 to 26 of Escherichia coli 16S rRNA gene and reverse primer, UNI16S-R (5'-ATGGTACCGTGTGACGGGCGGTGTTGTA), corresponded to the complement of positions 1411 to 1393 of Escherichia coli 16S rRNA (Brosius et al., 1978). PCR conditions were performed according to Beffa et al. (1996) and resulting PCR products were ligated into pGEM-T Easy vector.
The recN genes were selectively amplified from purified genomic DNA by using oligonucleotide primers designed to anneal to conserved positions in the 5' and 3' regions of Geobacillus spoIVB and ahrC genes (Zeigler, 2003). The forward primers, F1-2-(5'- CGA TTT GCG GCG ACG ATA C-3') and reverse primers, R1-1-(5'-TAC ACC ATG CAA AAA CGG TTA C-3') were used for amplification of the recN sequences. PCR conditions were carried out according to Beffa et al. (1996) and PCR products were cloned into plasmid using the pGEM-T Easy vector system.
16S rRNA and recN PCR products were ligated to the pGEM-T Easy vector according to the manufacturer’s instructions (Promega). The DNA sequences of 16S rRNA and recN genes were determined with a high throughput Applied Biosystems 3730XL sequencer by using 23 ABI 3730XLs sequencing kit (Macrogen, Netherlands). The sequences consisting of about 1600 nt of the recN gene and about 1400 nt of 16S rRNA gene were determined. These sequences were compared with those contained within GenBank (Benson et al., 1999) by using BLAST search (Altschul et al., 1990). Both the 16S rRNA and recN gene sequences of the species most closely related to our isolates were retrieved from the database.
HV Region
HV regions were corresponded to nucleotides 47–69 of Bacillus subtilis 16S rRNA 5'-GCYTAAYACATGCAAGTCGARCG-3', and 345–365 of Bacillus subtilis 16S rRNA, 5'-ACTGCTGCCTCCCGTAGGAGT-3'. HV regions of our Brevibacillus isolates derived from almost-complete 16S rRNA gene sequences of the strains. Also HV regions of Brevibacillus type strains were derived from 16S rRNA bacterial sequences available on the GenBank database. Multiple sequence alignment was performed with the CLUSTALW Software and the calculation of pairwise HV region sequence similarities were achieved using this software (Thompson et al., 1994).
Phylogenetic Analysis
16S rRNA, HV and recN genes were aligned using a multiple alignment software, CLUSTALW. Kimura’s two parameter model was used to calculate evolutionary distances (Kimura, 1980). Neigbour-joining method was used to perform the phylogenetic analyses (Sharma et al., 2008). Bootstrap analysis based on 1000 replicates was also carried out in order to obtain confidence levels for the branches (Felsenstein, 1985). The phylogenetic trees were constructed using the software MEGA7 (Kumar et al., 2016).
Xylanase Activity
Activity of extracellular crude xylanase produced from the isolates were determined according to Miller (1959). All isolates were grown in 1% xylan medium (w/v), at 55°C for 24 h and culture fluids were centrifuged at 13 000 rpm for 15 min at 4°C. The resulting supernatant was lyophilized and xylanase activity was measured with the dinitrosalicylic acid (DNS) reducing sugar assay with purified xylan from oat spelts as substrate.
The effect of pH on xylanase activity was determined by incubating 0.25 mL of crude enzyme and 0.75 mL of buffers in the pH range from 4.0 to 11.0, containing oat spelt xylan (1%). The pH stability was determined by verifying the remaining activity after incubating the crude enzymes for 36 hours at 4°C. Crude enzyme samples were incubated with different buffers in the pH range from 4 to 11.0, with 1.0 unit intervals.
The optimum temperature for xylanase activity was determined by assaying the crude enzyme extract at different temperatures (40–90°C), in its optimum pH, using DNS method (Miller, 1959).
Glucose Isomerase Activity
All isolates were grown to reach an optical density at 600 nm of about 0.6 and then was induced with 0.5% final D-xylose concentration at OD 0.6 (600 nm) for expression of glucose isomerase. After induction these isolates were grown for a further 4 h. Cells were harvested by centrifugation at 11 000 rpm for 5 min, resuspended and incubated in 50 mM Tris-HCl (pH 8.0) buffer, followed by sonication with Sartorius Labsonic M (80% amplitude, 0.6 cycles for 5 min) to release intracellular proteins. The sonicated extract was centrifuged at 14 800 rpm for 15 min to remove cell debris and obtain the cell-free extract, then assayed for glucose isomerase activity.
Cell extracts prepared by sonication and purified preparations were used as enzyme sources. Glucose isomerase activity was measured by incubating a reaction mixture that contained 10 mM MgSO4, 1 mM CoCl2, 0.1 M glucose, and the enzyme in 50 mM MOPS buffer (pH 7.0) at 85°C for 30 min in 100 μL reaction volume. The reaction was stopped by addition of 100 μL 0.5 M perchloric acid, 40 μL of 1.5% cysteine hydrochloride, 40 μL of 0.12% carbosol, respectively. 1.2 mL of 70% sulphuric acid was added and vortexed and then incubated at room temperature for 30 min. Absorbance was measured at 560 nm for fructose. The amount of fructose formed after the enzyme reaction was estimated by the cysteine-carbazole-sulfuric acid method (Dische and Borenfreund, 1951).
The effects of temperature on the glucose isomerase activities were determined spectrophotometrically using D-glucose as the substrate. The activity assay was performed at 25, 37, 45, 55, 60, 65, 70, 75, 80, 85, 90, 95 and 100°C for 30 min, and the results were expressed as relative activity (%) obtained at the optimum temperature.
The optimum pH of the enzyme was measured at 80°C and 560 nm by using the buffer solutions of different pH values and their relative activities (%) were measured. The following buffers (200 mM) were used: sodium acetate (pH 5.0–6.0), potassium phosphate (pH 6.0–7.0), Tris-HCl (pH 7.0–9.0) and Glisin-NaOH buffer (pH 9.0–10.0), and the results were expressed as relative activity (%) obtained at pH.
Nucleotide Sequence Accession Numbers
The nucleotide sequences of the 16S rRNA genes from isolates SG1, SG16, SG17, EG1, EG6, SG13, HIG11, HIG12, HIG17, HIG19, HIG22, HIG27, HIG28 and HIG29 determined in the present study have been submitted to GenBank under Accessions Numbers MN252553, MN252554, MN252555, MN252556, MN252557, MN252559, MN252560, MN252561, MN252562, MN252563, MN252564, MN252565, MN252566 and MN252567, respectively.
The nucleotide sequences of the recN genes from isolates SG1, SG13, SG16, SG17, EG1, EG6, and HIG12 determined in the present study have been submitted to GenBank under Accessions Numbers MN367795, MN367796, MN367797, MN367798, MN367799, MN367800 and MN367802, respectively.
RESULTS AND DISCUSSION
Environmental strains of thermophilic bacilli from some hot springs of Turkey were firstly isolated and then characterized on the basis of some morphologic and physiological properties, 16S rRNA, HV region and recN gene sequence analysis, thermostable xylanase and glucose isomerase production.
4 isolates from Omerbeyli hot spring (Aydin), 3 isolates from Alangullu hot spring (Aydin), 3 isolate from Camkoy Camur hot spring (Aydin), 2 isolates from Karakoc hot spring (Izmir) and 2 isolates from Kaynarca hot spring (Izmir), in total 14 isolates were obtained. The microorganisms with different colony formations and cell morphologies were subjected to conventional tests to characterize the isolates, and the results are shown in Table 1. These tests indicated that all of the isolates were Gram-positive, rod-shaped and motile and capable of endospore formation. Sub-terminal spores were observed in all eleven isolates, but central spores were also present in isolates EG1, SG1 and HIG17.
A total of 10 isolates were catalase-positive, 9 isolates were oxidase positive, while 6 isolates were oxidase- and catalase-positive. All isolates screened for temperature tolerance were able to grow between 35 and 50°C with variation among strains. It was noted that all isolates grow at 50°C within 24 h to 2 days. Salt was not required for growth since all the strains were able to grow in the unsupplemented medium. The isolates tolerated salt concentrations in medium supplemented with 1 to 3% NaCl (w/v). While none of the strains were able to grow in medium supplemented with 4% NaCl (w/v). In addition, the pH values tested ranged from 5.0 to 9.0. Almost all strains grew in pH ranging between 6.0 and 9.0.
The API 20E profiles of the isolates demonstrated biochemical diversity (Table 2). All strains were negative for ornithine decarboxylase, lysine decarboxylase and H2S production. As one can see, the utilization of carbohydrates varies; negative assimilation results were obtained for L-rhamnose and amygdalin overall strains. The carbohydrate assimilation test was positive for 10 strains on D-sorbitol, 6 strains for mannitol, and 2 strains for glucose, inositol and sucrose, respectively. Only 1 strain assimilate melibiose. The number of positive tests displayed by the isolates ranged from one to ten tests. 2 strains were positive only for one character.
A total of 1400 nucleotides of the 16S rRNA from 15 isolates were aligned and compared to sequences of related bacteria. The phylogenetic tree was constructed using the neighbour-joining method (Figs. 1, 2). Stackebrandt and Ebers (2006) reached the conclusion that strains belonging to the same genus that exhibit less than 98.7% 16S rRNA gene sequence similarity should be considered members of a different species. 16S rRNA sequence analysis showed that the 8 isolates showed ≥99% similarity to Geobacillus species, the other 7 isolates ≥99% similarity to Brevibacillus species. These similarities showed that the 8 isolates are members of the genus Geobacillus, the other 7 isolates are members of the genus Brevibacillus. In the literature, it is also known that the analysis of 16S rRNA sequences may be insufficient to distinguish between some closely related species. 16S rRNA gene sequence data is helpful for identification of thermophilic bacteria at genus level, but not at species. Thus, sequence data needs to be supported by other genomic methods which are expected to be more powerful and reliable approach to discriminate thermophilic bacteria even at strain levels.
Based on the results of 16S rRNA sequences, it was determined that the eight isolates were members of the genus Geobacillus. recN gene sequences of the eight isolates were determined. The recN sequences of eight isolates were aligned and compared to sequences of related bacteria. Neigbour-joining method was used to construct the phylogenetic tree (Fig. 3). Based on the analysis results, EG1 exhibits 99% similarity to the sequence of G. thermodenitficans; SG1 has 99% similarity to Group 3 (including G. thermoleoverans, G. kaustophilus, G. vulcani, G. lituanicus, G. stearothermophilus and G. thermocatenulotis); SG13, SG16 and SG17 show 91% similarity to the sequence of Group 3; EG7 exhibits 96% similarity to the G. tobeii; HIG12 and EG6 have 96% similarity to Group 3.
Zeigler (2003) suggested that recN sequence comparisons could accurately measure genome similarities for the Geobacillus genus and if the recN DNA sequences for two bacterial strains or isolates are less than 84% identical, we can be 95% confident that their genome sequences are less than 70% identical and that the bacteria belong to different species. If the recN DNA sequences are more than 96% identical, then by the same reasoning we can be 95% confident that the bacteria belong to the same species. If the recN sequences are between 84 and 96% identical, it is questionable whether the genome sequence identity is greater or less than 70%, making the species identity for these strains uncertain. Zeigler (2005) clustered this set of thermophilic Geobacillus strains into nine homology groups. Groups 1A, 2, 4A, 5, and 6A appear to correspond unambiguously to the species G. thermodenitrificans, G. stearothermophilus, G. thermoglucosidasius, G. toebii, and G. caldoxylosilyticus, respectively. Identification of the other four homology groups (1B, 3, 4B and 6B) with currently recognized species is somewhat more difficult, however. On the basis of a recN sequence similarity analysis, we suggest that isolate EG1 belongs to Group 1A and is a strain of G. thermodenitficans; isolates SG1 belongs to Group 3. Group 3 contains the type strains of G. kaustophilus, G. thermocatenulatus, G. thermoleovorans, G. vulcani and G. lituanicus. The group also includes B. caldotenax (96A4) and B. coldovelax (96A5). Since recN sequence of SG1 places these bacteria into group 3, to determine the species identities, further analysis is needed. Further analysis is also needed for these strains SG13, SG16, SG17, EG6, EG7 and HIG12 because their recN sequence have 91, 91, 91, 96, 96 and 96% similarity to the Geobacillus species discussed above respectively.
Based on the results of 16S rRNA sequences, it was determined that the seven isolates were members of the genus Brevibacillus. The HV region sequence is a useful marker for rapid and convenient identification and grouping of Brevibacillus species. HV sequences of the seven isolates were determined. The HV sequences of seven isolates were aligned and compared to sequences of related bacteria. Neigbour-joining method was used to construct the phylogenetic tree (Fig. 4). On the basis of the results of the analysis, HIG29 had 99% similarity to B. borstelensis; HIG17 and HIG27 exhibited 99% similarity to the B. thermoruber, B. formosus, respectively. HIG22 and HIG28 showed 99% similarity to the B. agri and B. formosus, respectively. HIG29, HIG17, HIG27, HIG22 and HIG28 resembled to the other type strains of Brevibacillus in range 94–99%.
HIG11 and HIG19 showed 99% sequence similarity to B. gelatini and these two isolates resembled to the other type strains of Brevibacillus in range 94–99%. And also HIG19 exhibited 97% similarity to HIG11. Also, this study demonstrated that the HV sequence (about 270 nt) of Brevibacillus species showed 94–99% similarity with each other. Goto et al. (2004) has determined that strains of each species of Brevibacillus clustered with their individual type strains. Thus, on the basis of HV similarity analysis, isolate HIG17, HIG27 and HIG29 are strains of B. thermoruber, B. formosus and B. borstelensis, respectively. HIG22 and HIG28 are strains of the B. agri. HIG11 is a strain of of Brevibacillus. Also HIG19 could be regarded as an independent species Brevibacillus. A DNA–DNA hybridization study in the future needs to be performed to confirm the identity and taxonomic group HIG11 and HIG19 strains isolated in our study.
So far, many attempts have been made to screen thermophilic and alcalophilic bacteria from natural sources (Yavuz et al., 2004; Mamao et al., 2006; Sharma et al., 2008; Kacagan et al., 2008; Inan et al., 2011a). The use of enzymes stable at a high pH and temperature especially contributes to technical and economical feasibilities of the hydrolysis process (Mamo et al., 1999).
Many studies have been reported on the isolation of xylanase-producing thermophilic bacteria. However, the optimum pH of the majority of xylanases from these thermophilic bacteria is neutrophilic. Although some strains have been reported to produce xylanase with good activity at pHs greater than 8.0, the optimum temperature for activity and stability is 50 to 55°C or below. Very few xylanases with an optimum temperature for activity exceeding 70°C at pH 9.0 or above have been reported so far (Gessesse, 1998). In the current study, xylanase was produced from thermophilic strains isolated from hot springs. As a result of the study, it was determined that only 5 of our strains (SG16, HIG11, HIG19, HIG22 and HIG28) have xylanase activity. Various pH affected the activity of extracellular xylanase from these strains. It was determined that the xylanases of strains HIG11, HIG19 and SG16 showed optimal pH at 9.0, whereas the xylanases of strains HIG22 and HIG28 represented optimal pH at 8.0, 8.0, and 7.5 respectively. Enzyme activity assay in various temperatures (40–90°C) were performed on afore mentioned optimum pH’s. The crude xylanase of strain SG16 had the highest temperature optima (80°C) among these xylanase while the others had optimal temperatures at 55–60°C. The optimum pH and temperature of crude xylanases of these strains were given in Figs. 5, 6.
The pH stability of these crude xylanases were determined between pH 4.0–11.0 at 4°C. The enzymes of HIG22, HIG28 and SG16 retained full activity in the pH range of 9.0–11.0 after 36 h. Xylanases of HIG11 and HIG19 maintained more than 70% of their activities at pH 11.0 after 36 h. The enzymes of HIG19 and HIG28 had 70% residual activity respectively at pH 8.0. However, these xylanases of HIG12 and SG16 retained full activity at pH 8.0 (data is not shown). Xylanases of all isolates were stable at alkaline pH, and the xylanase of SG16 was optimally active at alkaline pH and elevated temperatures.
The use of alkaline active xylanases provides direct enzymatic treatment of alkaline dough and does not require pH readjustment, which is costly and time-consuming steps. Alkaline xylanases, especially those that are operationally stable at higher temperatures, are more beneficial, saving cooling costs and time (Gessesse, 1998; Inan et al., 2011a). In this context, most of the xylanases of our isolates are expected to operate under biotechnologically desirable conditions such as high pH and temperature. By comparison, the properties of crude xylanases of our strains make them important candidates for biotechnological applications.
Glucose isomerase, in current processes for HFCS production, non-thermostable xylose (glucose) isomerases from mesophilic microorganisms producing 40–42% fructose syrup are used in immobilized enzyme reactors operating between 50 and 58°C, and with residence times of <1 h (Douglas and Jay, 1999). Therefore, an additional chromatographic step is necessary to obtain a 55% syrup concentration. But, isomeration at 95°C is enough to obtain 55% syrup without the additional concentration step (Silva et al., 2005) as equilibrium for the isomeration reaction is shifted to the production of fructose at high temperature. Also, commercial application of glucose isomerase demands an acidic pH optimum to enable starch liquefaction and glucose isomerization to be carried out in a single step. Because of advantageous of isomerization at low pH, acidic pHs are desirable for commercial applications of glucose isomerase. However, the optimum pH range of glucose isomerase is generally between pH 7.0 and 9.0 (Benson et al., 1999). Therefore, researchers have sought to isolate the microorganisms which are capable of producing more thermostable and acidic active enzyme than the one currently used in industry.
Glucose isomerase activities were detected in these strains examined and it was determined that only 6 (SG1, SG13, SG16, SG17 and HIG12) of these strains have glucose isomerase activity at the end of the study. The effect of temperature and pH on the glucose isomerase activity and stability were tested spectrophotometrically using D-glucose as substrate. These glucose isomerases of strains SG16, SG17 and HIG12 had optimal pH at 6.5, while the enzymes of strains SG1 and SG13 represented optimal pH at 7.0 and 7.5, respectively. The glucose isomerases of SG1 and SG13 showed optimum activity at neutral pH but the enzymes of others strains showed good activity at pH lower than 7.0 which is desirable for commercial applications of glucose isomerase.
To determine the effect of temperature on glucose isomerase activity, glucose isomerase activities was performed at pH 6.5 in a range between 25–100°C and the crude enzymes of strains SG13 and SG17 had the lowest temperature optima (75°C) among these enzymes while the others enzymes had optimal temperatures at 80°C. The optimum temperature and pH of glucose isomerases of these strains were given in Figs. 5, 6. Most of the commercial preparations of glucose isomerase have a temperature optimum of 60 to 65°C.
Glucose isomerase possessing a lower pH optimum, a higher temperature optimum reduces the formation of the coloured carbonyl compounds and may lead to lower costs of ion-exchange and carbon purification. Thus, acidic active glucose isomerase which is operationally active at higher temperatures is desirable for commercial applications of glucose isomerase. In this regard, our glucose isomerases could be a good candidate for biotechnology.
CONCLUSIONS
This study reports new isolates belonging to thermophilic Geobacillus and Brevibacillus species. Also, new strains which may be strains of a new species of Brevibacillus were determined in this study. 14 isolates were investigated in terms of their xylanase and glucose isomerase activities. As a result, we determined these isolates have xylaneses and glucose isomerase with better features compared to the enzymes of their reported relatives. We think that some of these enzymes may meet the needs of the industry.
To facilitate further studies with the enzymes, cloning and expression of the genes for both enzymes are in progress.
REFENRENCES
Abdel-rassol, T.M.A., Badr, S.A., and Omran, H.T., Glucose (xylose) isomerase production from thermotolerant and thermophilic bacteria, Afr. J. Biotechnol., 2012, vol. 11, pp. 15798–15801.
Allan, R.N., Lebbe, L., and Heyrman, J., Brevibacillus levickii sp. nov. and Aneurinibacillus terranovensis sp. nov., two novel thermoacidophiles isolated from geothermal soils of northern Victoria Land, Antarctica, Int. J. Syst. Evol. Microbiol., 2005, vol. 55, pp. 1039–1050.
Altschul, S.F., Gish, W., and Miller, W., Basic local alignment search tool, J. Mol. Biol., 1990, vol. 215, pp. 403–410.
Avcioglu, B., Eyupaoglu, B., and Bakir, U., Production and characterization of xylanases of a Bacillus strain isolated from soil, World. J. Microb. Biot., 2005, vol. 21, pp. 65–68.
Beffa, T., Blanc, M., and Lyon, P.F., Isolation of Thermus strains from hot compost (60 to 80°C), Appl. Environ. Microbiol., 1996, vol. 62, pp. 1723–1727.
Benson, D.A., Boguski, M.S., and Lipman, F.D.J., GenBank, Nucleic Acids Res., 1999, vol. 27, pp. 12–17.
Bergquist, P.L. and Morgan, H.W., The molecular genetics and biotechnological application of enzyme from extremely thermophilic eubacteria, in Molecular Biology and Biotechnology of Extremophiles, Herbert, R.A. and Sharp, R.J., Eds., Chapman and Hall, 1992.
Bhalla, A., Bischoff, K.M., and Sani, R.K., Highly thermostable xylanase production from a thermophilic Geobacillus sp. strain WSUCF1 utilizing lignocellulosic biomass, Front. Bioeng. Biotechnol., 2015, vol. 3, article 84.
Brosius, J., Palmer, M.L., and Kennedy, P.J., Complete nucleotide sequence of a 16S ribosomal RNA gene from Escherichia coli, Proc. Natl. Acad. Sci. U. S. A., 1978, vol. 75, pp. 4801–4805.
Canakci, S., Inan, K., and Kacagan, M., Evaluation of arabinofuranosidase and xylanase activities of Geobacillus spp. isolated from some hot springs in Turkey, J. Microbiol. Biotechnol., 2007, vol. 17, pp. 1262–1270.
Chanitnun, K. and Pinphanichakarn, P., Glucose(xylose) isomerase production by Streptomyces sp. Ch7 grown on agricultural residues, Braz. J. Microbiol., 2012, pp. 1084–1093.
Dische, Z. and Borenfreund, E., A new spectrophotometric method for the detection and determination of ketosugars and trioses, J. Biol. Chem., 1951, vol. 192, pp. 583–587.
Douglas, C.W. and Jay, K.S., Commodity scale production of sugars from starches, Curr. Opin. Microbiol., 1999, vol. 2, pp. 252–256.
Enez, B. and Fincan, S.A., Isolation, partial purification and characterization of thermostable xylanase from thermophilic Anoxybacillus sp. isolated from hot springs, Fresenius Environ. Bull., 2016, vol. 25, pp. 3038–3028.
Felsenstein, J., Confidence limits on phylogenies: an approach using the bootstrap, Evolution, 1985, vol. 39, pp. 783–791.
Gessesse, A., Purification and properties of two thermostable alkaline xylanases from an alkaliphilic Bacillus sp., Appl. Environ. Microbiol., 1998, vol. 64, pp. 3533–3535.
Goto, K., Fujita, R., and Kato, Y., Reclassification of Brevibacillus brevis strains NCIMB 13288 and DSM 6472 (=NRRL NRS-887) as Aneurinibacillus danicus sp. nov. and Brevibacillus limnophilus sp. nov., Int. J. Syst. Evol. Microbiol., 2004, vol. 54, pp. 419–427.
Goto, K., Kato, Y., and Asahara, M., Evaluation of the hypervaiable region in the 16S rDNA sequence as an index for rapid species identification in the genus Paenibacillus, J. Gen. Appl. Microbiol., 2002a, vol. 48, pp. 281–285.
Goto, K., Mochida, K., and Asahara, M., Application of the hypervariable region of the 16S rDNA sequence as an index for the rapid identification of species in the genus Alicyobacillus, J. Gen. Appl. Microbiol., 2002b, vol. 48, pp. 243–250.
Goto, K., Omura, T., and Yukihiko, H., Application of the partial 16S rDNA sequences as an index for rapid identification of species in the genus Bacillus, J. Gen. Appl. Microbiol., 2002, vol. 46, pp. 1–8.
Gregersen, T., Method for the distinction of Gram-negative from Gram-positive bacteria, Eur. J. Appl. Microbiol., 1978, vol. 5, pp. 123–127.
Harley, J.P. and Prescott, L.M., Laboratory Exercises in Microbiology, New York: McGraw-Hill, 2002.
Inan, K., Canakcı, S., and Belduz, A.O., Isolation and characterization of xylanolytic new strains of Anoxybacillus from some hot springs in Turkey, Turk. J. Biol., 2011a, vol. 35, pp. 529–542.
Inan, K., Bektas, Y., Canakci, S., and Belduz, A.O., Use of rpoB Sequences and rep-PCR for phylogenetic study of Anoxybacillus species, J. Microbiol., 2011b, vol. 49, pp. 782–790.
Inan, K., Canakcı, S., Belduz, A.O., and Sahin, F., Brevibacillus aydinogluensis sp. nov., a moderately thermophilic bacterium isolated from Karakoc hot spring in Turkey, Int. J. Syst. Evol. Microbiol., 2012, vol. 62, pp. 849–855.
Inan, K., Kacagan, M., Ozer, A., Belduz, A.O., Canakci, S., Algoriphagus trabzonensis sp. nov., isolated from freshwater, and emended description of Algoriphagus alkaliphilus, Int. J. Syst. Evol. Microbiol., 2015, vol. 65, pp. 2234–2240.
Inan, K., Ozer, A., Guler, H.I., Belduz, A.O., and Canakci, S., Brevibacillus gelatini sp. nov., isolated from a hot spring, Int. J. Syst. Evol. Microbiol., 2016, vol. 66, pp. 712–718.
Jin, L., Xu, Q., Liu, Z., Jia, D., Liao, C., Chen, D., and Zheng, Y., Immobilization of recombinant glucose isomerase for efficient production of high fructose corn syrup, Appl. Biochem. Biotechnol., 2017, vol. 183, pp. 293–306.
Jin, L.Q., Jin, Y.T., Zhang, J.W., Liu, Z.Q., and Zheng, Y.G., Enhanced catalytic efficiency and thermostability of glucose isomerase from Thermoanaerobacter ethanolicus via site-directed mutagenesis, Enzyme Microb. Technol., 2021, vol. 152, article 109931.
Kacagan, M., Canakci, S., and Sandalli, C., Characterization of a xylanase from a thermophilic strain of Anoxybacillus pushchinoensis A8, Biologia, 2008, vol. 63, pp. 599–606.
Kimura, M., A simple method for estimating evolutionary rates of base substitutions through comparative studies of nucleotide sequences, J. Mol. Evol., 1980, vol. 6, pp. 111–120.
Kumar, S., Stecher, G., and Tamura, K., MEGA7: molecular evolutionary genetics analysis version 7.0 for bigger datasets, Mol. Biol. Evol., 2016, vol. 33, pp. 1870–1874.
Logan, N.A., Forsyth, G., and Lebbe, L., Polyphasic identification of Bacillus and Brevibacillus strains from clinical, dairy and industrial specimens and proposal of Brevibacillus invocatus sp. nov., Int. J. Syst. Evol. Microbiol., 2002, vol. 52, pp. 953–966.
Lyytikäinen, K. and Backfolk, K., Refining of birch kraft pulp before or during xylanase treatment—effect on carbohydrate release and retention behaviour, Nordic Pulp Paper Res. J., 2017, vol. 32, pp. 87–96.
Mohammad, B.T., Daghistani, H.I.A., Jaouani, A., Abdel-Latif, S., and Kennes, C., Isolation and characterization of thermophilic bacteria from Jordanian hot springs: Bacillus licheniformis and Thermomonas hydrothermalis isolates as potential producers of thermostable enzymes, Int. J. Microbiol., 2017, vol. 2017, article ID 6943952.
Mamo, G., Gashe, B.A., and Gessesse, A., A highly thermostable amylase from a newly isolated thermophilic Bacillus sp. WN11, J. Appl. Microbiol., 1999, vol. 86, pp. 557–560.
Mamo, G., Hatti-Kaul, R., and Mattiasson, B., A thermostable alkaline active endo-β-1-4-xylanase from Bacillus halodurans S7: purification and characterization, Enzyme Microbiol. Technol., 2006, vol. 39, pp. 1492–1498.
Miller, G.L., Use of dinitrosalicyclic acid reagent for determination of reducing sugars, Anal. Chem., 1959, vol. 31, pp. 426–428.
Munster, M.J., Munster, A.P., Woodrow, J.R., and Sharp, R.J., Isolation and preliminary taxonomic studies of Thermus strains isolated from Yellowstone National Park, USA, Microbiology, 1986, vol. 132, pp. 1677–1683.
Murray, R.G.E., Doetsch, R.N., and Robinow, C.F., Determinative and cytological light microscopy, in Methods for General and Molecular Bacteriology, Gerhardt, P., Murray, R.G.E., Wood, W.A., and Krieg, N.R., Eds., Washington, DC: Am. Soc. Microbiol., 1994.
Nam, K.H., Glucose isomerase: functions, structures, and applications, Appl. Sci., 2022, vol. 12, p. 428.
Neifar, S., Hlima, H.B., Mhiri, S., Mezghani, M., Bouacem, K., Ibrahim, A.H., Jaouadi, B., Bouanane-Darenfed, A., and Bejar, S., A novel thermostable and efficient class II glucose isomerase from the thermophilic Caldicoprobacter algeriensis: biochemical characterization, molecular investigation, and application in high fructose syrup production, Int. J. Biol. Macromol., 2019, vol. 2020, article ID 1871934.
Pedersen, S., Industrial aspects of immobilized glucose isomerase, Bioprocess Technol., 1993, vol. 16, pp. 185–208.
Ovando-Chacon, S.L., Tacias-Pascacio, V.G., Ovando-Chacon, G.E., Rosales-Quintero, A., Rodriguez-Leon, A., Ruiz-Valdiviezo, V.M., and Servin-Martinez, A., Characterization of thermophilic microorganisms in the geothermal water flow of El Chichyn Volcano Crater Lake, Water, 2020, vol. 12, p. 2172.
Rengasamy, S., Subramanian, M.R., Perumal, V., Ganeshan, S.G., Khulaifi, M.M.A., AL-Shwaiman, H.A., Elgorban, A.M., Syed, A., and Thangaprakasam, U., Purification and kinetic behavior of glucose isomerase from Streptomyces lividans RSU26, Saudi J. Biol. Sci., 2020, vol. 27, pp. 1117–1123.
Savas, S., Adiguzel, A., Inan, K., Ozkan, H., Gulluce, M., Sahin, F., Molecular characterization of thermophilic bacteria isolated from Van City Ercis Town Hasanabdal hot spring, Rom. Biotechnol. Lett., 2009, vol. 14, pp. 4445–4454.
Sharma, A., Pandey, A., and Shouche, Y.S., Characterization and identification of Geobacillus spp. isolated from Soldhar hot spring site of Garhwal Himalaya, India, J. Basic. Microb., 2008, vol. 48, pp. 1–8.
Silva, E.A.B.D., Souza, A.A.U.D., and Souza, S.G.U.D., Simulated moving bed technology in the reactive process of glucose isomerization, Adsorption, 2005, vol. 11, pp. 847–851.
Sinir, G.O., Suna, S., Inan, S., Bagdas, D., Tamer, C.E., Copur, O.U., Sigirli, D., Sarandol, E., Sonmez, G., Ercan, I., Evrensel, T., Eren, E., Incedayi, B., Effects of long-term consumption of high fructose corn syrup containing peach nectar on body weight gain in Sprague Dawley rats, Food. Sci. Technol., 2017, vol. 37, pp. 337–343.
Stackebrandt, E. and Ebers, J., Taxonomic parameters revisited: tarnished gold standards, Microbiol. Today, 2006, vol. 33, pp. 152–155.
Stackebrandt, E., Wilhelm, F., and Garrity, G.M., Report of the ad hoc committee for the re-evaluation of the species definition in bacteriology, Int. J. Syst. Evol. Microbiol., 2002, vol. 52, pp. 1043–1047.
Suleiman, A.D., Abdul, N., Mohd, H., Mohd, F., and Yasid, N.A., Effect of cultural conditions on protease production by a thermophilic Geobacillus thermoglucosidasius SKF4 isolated from Sungai Klah hot spring Park, Malaysia, Molecules, 2020, vol. 25, pp. 1–14.
Taha, G.H., El-Shatoury, E.H., Tolba, S.T.M., and Ibrahim, M.K., Potential enzyme activity of thermophilic bacteria from hot spring in Egypt, African J. Biol. Sci., 2020, vol. 16, pp. 197–206.
Thompson, J.D., Higgins, D.G., and Gibson, T.J., CLUSTAL W: improving the sensitivity of progressive multiple sequence alignment through sequence weighting, position-specific gap penalties and weight matrix choice, Nucleic Acids Res., 1994, vol. 22, pp. 4673–4680.
Touzel, J.P., O’Donohue, M., and Debeire, P., Thermobacillus xylanilyticus gen. nov., sp. nov., a new aerobic thermophilic xylan-degrading bacterium isolated from farm soil, Int. J. Syst. Evol. Microbiol., 2000, vol. 50, pp. 315–320.
Tsotetsi, L., Prenaven, R., Modise S.J., and Monapathi, M., Isolation and identification of xylanase producing thermophilic bacteria from compost piles and optimization of xylanase production, J. Biotechnol. Res., 2020, vol. 11, pp. 122–132.
Vandamme, P., Plot, B., and Gillis, M., Polyphasic taxonomy, a consensus approach to bacterial systematic, Microbiol. Rev., 1996, vol. 60, pp. 407–438.
Yavuz, E., Gunes, H., and Harsa, S., Identification of extracellular enzyme producing thermophilic bacilli from Balcova (Agamemnon) geothermal site by ITS rDNA RFLP, J. Appl. Microbiol., 2004, vol. 97, pp. 810–817.
Zargaraan, A., Kamaliroosta, L., Yaghoubi, A.S., and Mirmoghtadaie, L., Effect of substitution of sugar by high fructose corn syrup on the physicochemical properties of bakery and dairy products: a review, Nutr. Food Sci. Res., 2016, vol. 3, pp. 3–11.
Zeigler, D.R., Gene sequences useful for predicting relatedness of whole genomes in bacteria, Int. J. Syst. Evol. Microbiol., 2003, vol. 53, pp. 1893–1900.
Zeigler, D.R., Application of a recN sequence similarity analysis to the identification of species within the bacterial genus Geobacillus, Int. J. Syst. Evol. Microbiol., 2005, vol. 55, pp. 1171–1179.
Zhang, X., Al-Dossary, A., Hussain, M., Setlo, P., Li, J., Applications of Bacillus subtilis spores in biotechnology and advanced materials, Appl. Environ. Microbiol., 2020, vol. 86, no. 17.
Author information
Authors and Affiliations
Corresponding author
Ethics declarations
The authors declare that they have no conflict of interest. This article does not contain any studies involving animals or human participants performed by any of the authors.
Rights and permissions
About this article
Cite this article
Kadriye İnan Bektas Isolation and Molecular Identification of Xylanase and Glucose-Isomerase Producer Geobacillus and Brevibacillus Strains from Hot Springs in Turkey. Biol Bull Russ Acad Sci 48 (Suppl 2), S34–S46 (2021). https://doi.org/10.1134/S1062359021150085
Received:
Revised:
Accepted:
Published:
Issue Date:
DOI: https://doi.org/10.1134/S1062359021150085