Abstract
The technique that enables simultaneous evaluation of water potential in the substomatal cavity of the intact leaf (ψwа) and rate of its photosynthetic CO2/H2O gas exchange was applied to a halophyte Suaeda altissima (L.) Pall. Formation of a water-potential gradient in a whole plant, rate of CO2 uptake, rate of transpiration, and concentrations of Na+ and Cl– ions in the plant organs were determined under conditions of chloride–sodium salinization of a nutrient solution. It was found that the salinization decreases not only biological productivity of the plants but also their capacity to accumulate Cl– in the amounts equivalent to Na+ accumulation. High salinity also diminished the gradient of water potential between a nutrient solution and the apoplast of the cells in the leaf substomatal cavity due to the increase in ψwа and, respectively, decrease in the water stream from roots to leaves and the rate of CO2/H2O gas exchange of the leaf. It was shown that the decrease in the water potential in the interface between liquid and gaseous phases in the apoplast of the substomatal cavity (expressed in the ψwа value) plays an essential role in the regulation of water uptake under salinization conditions. It is supposed that the NaCl-induced increase in ψwа is a consequence of the suppression of photosynthesis and a resultant osmolyte shortage together with the decrease in plant productivity due to the stress impact exerted by NaCl.
Similar content being viewed by others
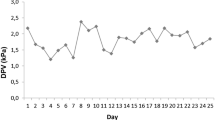
Explore related subjects
Discover the latest articles, news and stories from top researchers in related subjects.Avoid common mistakes on your manuscript.
INTRODUCTION
Soil salinization is one of the most harmful environmental factors that plants face. More than 800 million hectares and at least 20% of world’s irrigated areas are more or less salinized [1]. Salt stress entails both osmotic and toxic impacts on the plants and represses the essential vital processes and physiological functions—photosynthesis, mineral nutrition, and growth. Ultimately, it restricts the harvest. Global heating and intensifying of regional aridization lead to augmentation of the salinized areas [2]. Therefore, investigating the mechanisms of salinity tolerance and adaptations to this factor is traditionally in focus of plant physiology.
Water balance of plants and their tolerance to salt and osmotic stresses depend of water potential upward gradient in the soil solution–root–shoot–atmosphere system. The water potential decrease is considerably contributed by osmolytes at particular stages of water transport. These metabolites are low-molecular weight organic compounds fulfilling both osmoregulation and protection of biopolymers [3]. Osmolytes diminish the water potential not only in the intracellular compartments but, apparently, in the apoplast as well [4].
A novel method for the quantitative determination of water potential of mesophyll cells’ apoplast in the leaf’s substomatal cavity (ψwа) has been recently advanced [4]. This technique, simultaneously with ψwа, enables measuring photosynthetic CO2 gas exchange and transpiration in the intact leaf. By means of this approach, it was revealed that water potential sharply decreases at the interface between the aqueous and gaseous phases of the mesophyll cells’ apoplast in the leaf’s substomatal cavity [4]. The application of this method to pine needles also showed the decrease in ψwа under conditions of salt stress and water deficit [5]. The data obtained on maize seedlings supports the suggestion that the water stress-induced accumulation of organic osmolytes in the leaf cells decreases ψwа, while the osmolyte depletion increases this index [6]. We hypothesized that the osmolytes, which are synthesized in the cells, are transported by a gradient of their concentration to the apoplast and, thus, decrease its water potential to some extent. The present study continues a series of works dealing with the physiological role of ψwа regulation under stress.
Herein we studied the halophyte Suaeda altissima to reveal how the chloride–sodium salinization of its hydroponic substrate influences the plant growth, water potential of the apoplast in substomatal cavity of the leaf (ψwа), gradient formation of this potential in the whole plant, and CO2/H2O gas exchange of the intact leaves. We attempted this study to validate the suggestion that the ψwа value indicates not only biological productivity of Suaeda altissima but also its capacity to withstand stress impacted by salinization.
MATERIALS AND METHODS
Plant objects. Seeds were collected from the plants of Suaeda altissima (L.) Pall. inhabiting on shores of the Elton salt lake (Volgograd region, Russia). The seeds were germinated in wet sand at room temperature. After 3 weeks, the seedlings were transplanted to 3-L glass vessels (four plants per vessel) onto aerated nutrient solution (NS) [7]. The plants grew in a controlled growth chamber at 24ºC in water culture under illumination with Dna3 250-W high-pressure lamps (Reflux, Russia) at light intensity of 500 µmol/(m2 s) and 16/8 h (day/night) photoperiod. The nutrient solution was refreshed every week over the first 3 weeks. When the plants achieved the 42-day age, sodium chloride was added to NS in a stepwise manner with 50- or 100-mM increment to attain the final concentrations of 0, 250, or 750 mM. These counterparts were designated, respectively, as the control, treatment I, or treatment II. Thereafter, NS was no more changed, and the plants grew until the 60-day age after which they were analyzed.
Osmotic pressure of the solutions was measured cryoscopically with an OSMoMaT 030-D-RS osmometer (Gonotec, Germany) according to the manufacturer’s instructions.
Determination of Na+ and Cl– in plant organs. The organs were dried at 90°C and were ground to a powder state. The ions were extracted from the samples by 2-min boiling in distilled water followed by filtration of the extract. Concentration of Na+ was assayed in the filtrates with an FPA-2-01 flame photometer (OAO ZOMZ, Russia). Concentration of Cl– was evaluated by the extract titration with mercury ions, according to State standard R 4245-72 using a TopBuret semiautomatic titrator (Eppendorf, Germany).
Stationary photosynthetic CO2/H2O gas exchange of the intact S. altissima leaves were examined with a LI-820 infrared gas analyzer (Li-Cor, United States) in an open flow-through system at the atmospheric CO2 concentration and PAR intensity of 200 µmol/(m2 s) [8]. Here, the intact leaf attached to the plant was mounted in a clip chamber at room temperature and was illuminated by a KL 1500 LCD light source (Schott, Germany) equipped with a 150-W halogen lamp (Philips, the Netherlands) through a fiber-optic light guide. The leaf was allowed to adapt to the conditions of the clip chamber for 15–20 min to attain a stationary regime of photosynthetic CO2 gas exchange. The leaf transpiration was derived from the difference between gas humidity in the inlet and outlet of the leaf chamber. In the inlet gas flow, the constant humidity was maintained at 25°C with a LI-610 humidifier (Li-Cor). In the outlet of the leaf chamber, the air humidity was measured with a HMP50 psychrometric sensor (Vaisala Intercap, Finland). Atmospheric air, previously accumulated in a 30-L polyethylene gasholder, was used as injected gas. The gas flow rate (100 mL/min) enabled continuous 5-h operation of the system. The gas-mixing unit allowed the stable maintenance of CO2 concentration at 380 ppm in the air flow at the entrance to the leaf chamber.
Determination of water potential in the apoplastic gas–water interface on the surface of mesophyll cells lining the substomatal cavity. According to the theory of general thermodynamic interaction of statistical physics [9, p. 274], the equilibrium pressure of water vapor above the surface of water solution is related to the water chemical potential ψ by the formula [10, p. 15]
where e is the equilibrium vapor pressure above the aqueous solution; eo is a pressure of saturated water vapor above the surface of pure water (ψo = 0) at the absolute temperature of T; R is the universal gas constant (8.31441 J/(mol K)); T is absolute temperature (K); and V is molar volume of water (18 × 10–6 m3/mol). Therefore,
The physical dimension of water potential is seen from the formula (2) and is expressed in J/m3 or Pa.
The relative humidity (RH) is defined by the formula
where eo is the pressure of saturated water vapor (Pa) and e is the actual pressure of water vapor (Pa).
The value of air humidity at which transpiration ceases was obtained by a linear approximation of the experimentally obtained transpiration rate as a function of RH [4]. The water potential can be calculated from equations (2) and (3) using instrumentally determined RH values in the substomatal cavity at a given leaf temperature.
Statistics. All the experiments were carried out in three biological replications. Means and their SEs are reported. Different letters designate means, which significantly differ from each other (at P < 0.05, two-way ANOVA).
RESULTS
We investigated the role and the regulation of water potential of mesophyll cells’ apoplast in substomatal cavity of the S. altissima leaf under conditions of salt stress at two enhanced NaCl concentrations in NS (see above). In the control NS, free of exogenous salt, only traces of NaCl were present as impurities to other reagents.
Plant Growth and Water Content in the Organs
Sodium chloride, introduced to NS, stimulated plant growth at 250 mM and retarded it at 750 mM. In treatment II, the retardation manifested as more than twofold decrease in shoot dry biomass per plant as compared with the control and fivefold decrease in comparison with treatment I (Table I). The differences in root dry biomass between the treatments were qualitatively similar but more pronounced. Thus, in treatment II, the root dry biomass was six times lower than in treatment I and three times lower than in the control plants.
Water content in the roots was approximately the same, nearly 90% of total root mass in all cases (Table I). In the shoots, this index considerably differed in the differently treated plants, namely, 78% in the control, 82% in treatment I, and 91% in treatment II.
Na+ and Cl– Content in the Organs
Roots and leaves of S. altissima were found to accumulate both Na+ and Cl– regardless of the ambient NaCl concentrations (Fig. 1). There were Na+ and Cl–, respectively, at 14 and 46 mmol/kg fr wt in the roots (Fig. 1a) and at 79 and 107 mmol/kg fr wt in the shoots (Fig. 1b), even in the control. With the higher salinity of the nutrient solution, higher ionic content was found in the organs. In the plants grown in the maximally salted medium (treatment II), Na+ and Cl– were, respectively, present at 478 and 605 mmol/kg fr wt in the roots (Fig. 1a) and at 797 and 535 mmol/kg fr wt in the shoots (Fig. 1b). Therefore, with the increased NaCl concentration in the NS, either roots or leaves accumulated more amounts of both ions. The difference between Na+ cation and Cl– anion in their consumption by the plant was also found (Fig. 1). At the equal initial ambient concentrations of Na+ and Cl–, the S. altissima roots contained noticeably more amounts of Cl– than Na+ in all the experimental treatments (Fig. 1a). By contrast, the leaves of all but control plants contained more sodium than chloride (Fig. 1b). In either roots or leaves, the total content of Na+ exceeded that of Cl–. In this regard, organic anions appear to be the most probable candidates for the role of compensators of the surplus positive charges of sodium ions in the leaves. From the data of Table 1 and Figs. 1a and 1b, the capacity of the roots and leaves to absorb the ions was calculated and normalized to one plant (Figs. 1c, 1d). The plants were revealed to consume the maximal amounts of Na+ and Cl– if the ambient salt concentration was optimal for growth (250 mM). At the higher NaCl concentration, 750 mM, one plant consumed lesser amounts of the ions than when at 250 mM, although the accumulation, if normalized to unit of fresh biomass, increased with the increased outer salt concentration and attained the maximum at 750 mM NaCl in NS (Figs. 1a, 1b). This means that the inhibition of the plant growth rate, i.e., decrease in root and shoot biomass, was a limiting factor of the salt accumulation under the salt stress.
Gradient of Water Potential in the Whole Plant and Photosynthetic CO2 /H2O Gas Exchange in the Leaves
Water moves from the soil to the root and, further, to the above-ground organs by a gradient of water potential [12, p. 81–139]. To evaluate this gradient in the whole-plant system, we measured the water (osmotic) potential of the NS and the water potential of the substomatal cavity of the S. altissima leaves (ψwa) at the end of the experiment, i.e., after 60-day cultivation of the plants (Figs. 2a, 2b). It was found that the values of water (osmotic) potential of nutrient solutions significantly differed depending on a particular experimental counterpart (Fig. 2a). The differences were accounted for by both different concentrations of NaCl added to NS and different rates of ion consumption by the growing plants. Significantly lower values of water potential of NS in treatments I and II than in the control were caused by large amounts of the introduced NaCl. In the NS of treatment II, the osmotic potential was the least, –2.34 МPa. In treatment I, it was –0.54 МPa, i.e., four times larger. In the control NS, the osmotic potential was –0.026 МPa, which was as much as two orders higher than in treatment II. However, such differences between the water potentials of nutrient solutions in the different experimental counterparts (Fig. 2a) did not significantly affect their gradients in the NS–root–shoot system, because the decrease in water potential of the apoplast in the substomatal cavity of the leaf (ψwa) brought about the overwhelming contribution to this gradient (Fig. 2b). Even the minimal water (osmotic) potential of NS in treatment II was almost tenfold higher than the water potential of the substomatal cavity of the leaf in this treatment (–22 МPa). In the control plants and those of treatment I, the water (osmotic) potential of NS further exceeded the water potential of the substomatal cavity of the leaf (ψwa) (Figs. 2a, 2b). Therefore, it is the water potential of the substomatal cavity, which is considerably lower than the osmotic (water) potential of NS, that physically enables water transport through xylem from roots to leaves in all experimental treatments. Implementation of this physical capability was instrumentally evaluated by leaf transpiration rate (Fig. 3a). In treatment II (with the maximally salted NS), the transpiration rate was approximately half of that in the control or treatment I. The rate of the photosynthetic assimilation of carbon dioxide correlated with the transpiration of the leaves in different experimental counterparts (Fig. 3b). The values of the CO2/H2O gas exchange rates were the same as in the control in the leaves of treatment I but twofold lower than the control in treatment II (Fig. 3).
DISCUSSION
Water transport from roots to above-ground organs requires maintaining the correspondingly directed gradient of water potential. The gradient, in particular, depends on concentrations of substances being higher in the shoots than in the roots. In our experiments, the concentrations of Na+ and Cl– ions in the S. altissima organs increased with the increase in the NaCl concentration in the nutrient solution. Here, the Na+ level was higher in the leaves than in the roots; this meets the requirement of an ascending water flow. However, the Cl– concentration gradient was inversely directed, i.e., the leaves were significantly less enriched with this ion than the roots. Taking a higher osmotic pressure (osmolarity) in the leaves than in roots of S. altissima [11] into account, together with an electrical neutrality of intracellular solutions [12, p. 81–139], we can assume that the lack in charge of cellular Cl– is compensated by organic anions. The latter ions not only maintain electrical neutrality of the intracellular media, when the cells assimilate Na+ more intensively than Cl–, but also contribute to the gradient of osmotic potential and, consequently, the gradient of water potential between the root and shoot. Salination of the nutrient solution was accompanied by not only the salt accumulation in the roots and leaves per unit of their fresh biomass (Fig. 1) but also the marked suppression of increase in biomass (Table 1).
By the end of the experiment, the nutrient solutions significantly differed in respect to their water (osmotic) potentials in different counterparts (Fig. 2a). The water potential of the leaf apoplast (ψwа) was approximately one third lower in treatment II (with the maximal salinity) than in the control or treatment I (Fig. 2b). As a consequence, the gradient of water potential between nutrient solution and leaf apoplast in the substomatal cavity (motive power of an upward water transport) was significantly lower in treatmment II than in the control and treatment I. These differences agree with the lower rates of the transpiration and photosynthetic CO2 assimilation in treatment II (Fig. 3a) in comparison with the control and treatment I (Fig. 3b). The water flow equals the product of water-potential gradient and water conductivity [12, p. 81–139]. The fact that the ratios between the photosynthetic gas exchange, leaf transpiration, and gradients of water potentials coincided in the different experimental counterparts allows for drawing the conclusion on a stomatal limitation of a water flow through the leaves and general disturbance of water supply of the S. altissima plants under conditions of salinization. One may suggest that restriction in a water flow in the salt-stressed plant primarily interrupts the donor-acceptor interactions between underground and above-ground parts of the plant. According to literature [13], the possibility of nonstomatal limitation of CO2 gas exchange under light could not be excluded in a general case. However, under the particular conditions of our experiments and the precision of the measurements, the found fitness between decrease in the CO2 gas exchange and decrease in the transpiration in the illuminated leaf (Fig. 3) allows neglecting a contribution of the dark respiration to the stomatal limitation of CO2/H2O gas exchange.
As was shown earlier [3], such photosynthetic products as reducing sugars may behave as osmolytes involved in the reduction of decrease in ψwа. However, the lower level of Cl– in comparison with Na+ in the S. altissima leaves points to possible biosynthesis of organic anions compensating surplus positive charges of sodium ions. The organic anions also appear to contribute to the maintenance of water-potential gradient in the whole plant of this species.
Our data on the CO2 gas exchange indicate that photosynthesis of the plants that were subjected to the solution of the highest salinity (variant II) was half of that in the control or treatment I (Fig. 3b). Such a decrease in photosynthesis rate should significantly restrict the formation of organic osmolytes in treatment II in comparison with other treatments. As a consequence, the apoplastic water potential in the substomatal cavity (ψwа) should increase. In fact, the estimated value of ψwа was approximately one third higher in treatment II than in the control or treatment I (Fig 2b). Therefore, although the cells of S. altissima accumulated salt, which decreased their water potential, the motive power of water consumption by the plants also decreased due to suppression of the photosynthetic gas exchange and decrease in intracellular content of organic osmolytes under conditions of salinization (Figs. 2b, 3). As an outcome of a stomatal limitation of water transport from roots to leaves, the simultaneous reduction in photosynthetic CO2 gas exchange, and decrease in assimilation capacity of the plants, the biomass accumulation was slowed down (Table 1). The results demonstrate that the level of water potential of the apoplast in substomatal cavity of the leaves is indicative of not only bioproductivity but also the strength of the salt stress impact on the halophyte S. altissima.
Another question is addressed to the nature of processes decreasing the water potential of the apoplast in the substomatal cavity. Our earlier study [4] revealed that the sharp fall in the water potential in the leaf apoplast at the interface between aqueous and gaseous phases is universal since it occurs in both halophytes and glycophytes. Presumably, the clue to unravel this mechanism involves capillary forces in the apoplast in combination with diminishing the apoplast’s osmotic potential afforded by a transfer of organic osmolytes from the cells into the cell wall capillars.
REFERENCES
FAO land and plant nutrition management service, 2008. http://www.fao.org/ag/agI/agII/spush3.
Munns, R. and Tester, M., Mechanisms of salinity tolerance, Annu. Rev. Plant Biol., 2008, vol. 59, p. 651. https://doi.org/10.1146/annurev.arplant.59.032607.092911
Ahmad, P. and Sharma, S., Salt stress and phyto-biochemical responses of plants, Plant Soil Environ., 2008, vol. 54, p. 89.
Voronin, P.Yu., Rakhmankulova, Z.F., Shuyskaya, E.V., Maevskaya, S.N., Nikolaeva, M.K., Maksimov, A.P., Maximov, T.Ch., Myasoedov, N.A., Balnokin, Yu.V., Rymar, V.P., Valdayskih, V.V., and Kuznetsov, Vl.V., New method for quantitative determination of water potential of mesophyll cells’ apoplast in substomatal cavity of the leaf, Russ. J. Plant Physiol., 2017, vol. 64, p. 452. https://doi.org/10.1134/S1021443717020133
Voronin, P.Yu., Rakhmankulova, Z.F., Tarnopolskaya, E.E., and Kuznetsov, Vl.V., Closure of stomata in water-stressed pine needles results from the decreased water potential of the mesophyll apoplast in the substomatal cavity, Russ. J. Plant Physiol., 2018, vol. 65, p. 518. https://doi.org/10.1134/S1021443718030081
Voronin, P.Yu., Maevskaya, S.N., and Nikolaeva, M.K., Physiological and molecular responses of maize (Zea mays L.) plants to drought and rehydration, Photosynthetica, 2019, vol. 57, p. 850. https://doi.org/10.32615/ps.2019.101
Robinson, S.R. and Downton, W.J.S., Potassium, sodium and chlorid ion concentration in leaves and isolated chloroplasts of the halophyte Suaeda australis R. Br., Aust. J. Plant Physiol., 1985, vol. 12, p. 471.
Voronin, P.Yu., Experimental installation for measurements of chlorophyll fluorescence, CO2 exchange, and transpiration of a detached leaf of a detached leaf, Russ. J. Plant Physiol., 2014, vol. 61, p. 269. https://doi.org/10.1134/S1021443714020174
Reif, F., Fundamentals of Statistical and Thermal Physics, New York: McGraw-Hill, 1965.
Laisk, A.Kh., Kinetika fotosinteza i fotodykhaniya C 3 ‑rastenii (Photosynthesis and Photorespiration Kinetics of C3 Plants), Moscow: Nauka, 1977.
Balnokin, Yu.V., Kotov, A.A., Myasoedov, N.A., Khailova, G.F., Kurkova, E.B., Lun’kov, R.V., and Kotova, L.M., Involvement of long-distance Na+ transport in maintaining water potential gradient in the medium-root-leaf system of a halophyte Suaeda altissima, Russ. J. Plant Physiol., 2005, vol. 52, p. 489.
Nobel, P.S., Physicochemical and Environmental Plant Physiology, San Diego: Academic, 1999.
Pan, T., Liu, M., Kreslavski, V.D., Zharmukhamedov, S.K., Nie, Ch., Yu, M., Kuznetsov, Vl.V., Allakhverdiev, S.I., and Shabala, S., Non-stomatal limitation of photosynthesis by soil salinity, Crit. Rev. Environ. Sci. Technol., 2020, vol. 50. https://doi.org/10.1080/10643389.2020.1735231
Funding
This work was supported by State Task no. AAAA-A19-119041690035-9.
Author information
Authors and Affiliations
Corresponding author
Ethics declarations
Conflict of interests. The authors declare that they have no conflicts of interest.
Statement on the welfare of humans or animals. This article does not contain any studies involving animals performed by any of the authors.
Additional information
Translated by A. Aver’yanov
Abbreviations: ψwа—water potential of apoplast in substomatal cavity of the leaf; NS—nutrient solution.
Rights and permissions
About this article
Cite this article
Voronin, P.Y., Myasoedov, N.A., Khalilova, L.A. et al. Water Potential of the Apoplast in Substomatal Cavity of the Suaeda altissima (L.) Pall. Leaf under Salt Stress. Russ J Plant Physiol 68, 519–525 (2021). https://doi.org/10.1134/S1021443721030171
Received:
Revised:
Accepted:
Published:
Issue Date:
DOI: https://doi.org/10.1134/S1021443721030171