Abstract
Extractive atmospheric pressure photoionization (EAPPI) mass spectrometry was designed for rapid qualitative and quantitative analysis of chemicals in complex matrices. In this method, an ultrasonic nebulization system was applied to sample extraction, nebulization, and vaporization. Mixed with a gaseous dopant, vaporized analytes were ionized through ambient photon-induced ion–molecule reactions, and were mass-analyzed by a high resolution time-of-flight mass spectrometer (TOF-MS). After careful optimization and testing with pure sample solution, EAPPI was successfully applied to the fast screening of capsules, soil, natural products, and viscous compounds. Analysis was completed within a few seconds without the need for preseparation. Moreover, the quantification capability of EAPPI for matrices was evaluated by analyzing six polycyclic aromatic hydrocarbons (PAHs) in soil. The correlation coefficients (R 2) for standard curves of all six PAHs were above 0.99, and the detection limits were in the range of 0.16–0.34 ng/mg. In addition, EAPPI could also be used to monitor organic chemical reactions in real time.

ᅟ
Similar content being viewed by others
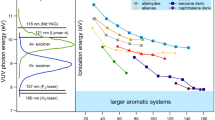
Explore related subjects
Discover the latest articles, news and stories from top researchers in related subjects.Avoid common mistakes on your manuscript.
Introduction
In recent years, the development of ambient ionization techniques for mass spectrometry has been driven by the urgent need for techniques for rapid detection of trace components in complex matrices [1–3]. Since the introduction of desorption electrospray ionization (DESI) [4] and direct analysis in real time (DART) [5] in 2004 and 2005, respectively, over 30 ambient ionization techniques have been developed. In most cases of ambient mass spectrometry, analytes are desorbed from surfaces and ionized by a variety of existing atmospheric pressure ionization methods, such as electrospray ionization (ESI), atmospheric pressure chemical ionization (APCI), atmospheric pressure photoionization (APPI) [6], and plasma based methods [7, 8]. Among these ionization methods, APPI has attracted increasing attention because of its favorable characteristics of soft ionization, no polarity discrimination, and reduced ion suppression compared with ESI [9].
There are currently three well-established ambient methods that have been derived from APPI, namely desorption APPI (DAPPI) [10], laser ablation APPI (LA APPI) [11], and solvent jet desorption capillary photoionization (DCPI) [12]. In DAPPI and solvent jet DCPI, a confined solvent vapor jet formed with the assistance of a relatively strong sheath gas flow is applied to desorb compounds from the surfaces of bulk substrates. Powdered samples should be compressed into a pellet or sprinkled on a double-sided tape for analysis [13]. In LA APPI, an expensive infrared laser [11] is used to ablate analytes from water-rich surfaces, and the ablated neutral compounds are intercepted and ionized by an orthogonal hot solvent vapor jet (e.g., toluene or anisole). These methods allow for fast qualitative characterization of the surfaces of tablets [10], biological tissues [11, 12], and environmental samples [14]. However, quantification of compounds within matrices is not possible because these techniques provide surface analysis only.
Ultrasonic nebulization has been applied as a sampling method for solutions in organic mass spectrometry [15]. Ultrasonic nebulization extraction (UNE) uses ultrasound to extract samples from matrices [16]. Recently, we applied UNE combined with low pressure photoionization (LPPI) to the analysis of compounds in solution and matrices [17]. LPPI can produce molecular ions and solvent adducts, which are complementary to the protonated ions of APPI. However, the operation of vacuum ultraviolet (VUV) lamp in the vacuum chamber is inconvenient, and the gas density in the ionization region is relatively low compared with that of APPI [18].
The aim of the present study was to combine UNE with APPI for direct analysis of real samples without chromatography separation. This method is called extractive atmospheric pressure photoionization (EAPPI), and is simpler, more convenient, and has better sensitivity than UNE-LPPI. In addition, it has the following three advantages over other existing photoionization-based techniques: (1) EAPPI can enable fast screening of components in matrices rather than just surface analysis; (2) EAPPI can realize rapid quantitative analysis in matrices with good correlation coefficients and detection limits; and (3) EAPPI can be used to monitor organic reactions in real time.
Experimental
Chemicals and Sample Preparation
Hydroxylamine hydrochloride, methyltestosterone, cholesterol, and 9,10-phenanthrenequinone were purchased from Sigma-Aldrich (St. Louis, MO USA). Fluorene, phenanthrene, acenaphthylene, acenaphthene, pyrene, and chrysene were obtained from TCI (Tokyo, Japan). All these chemicals (>99% purity) were used without further purification. High performance liquid chromatography grade toluene, anisole, methanol, acetonitrile, and water were obtained from Sinopharm Chemical Reagent Co. (Shanghai, China). Nitrogen (99.9%) for use as a carrier gas and refrigerated industrial grade liquid nitrogen (99.8%) for use as a drying gas were purchased from Nanjing Special Gas Factory Co., Ltd. (Nanjing, China). Unless stated otherwise, all the experiments were conducted with toluene as dopant.
A stock solution of 9,10-phenanthrenequinone (1 μg/mL) prepared in methanol/water (v:v = 3:1) was used for the parameter optimization of EAPPI. A stock solution of cholesterol (10 μg/mL) was also prepared in methanol/water (v:v = 3:1). Compound amino acid capsules were purchased from Shenzhen Wanhe Pharmaceutical Co. Ltd.d (Shenzhen, China). For analysis, a pellet weighing approximately 0.05 mg was taken from a capsule and placed in 4 mL of methanol/water (v:v = 3:1) in the nebulization cell. A sample of aromatic petroleum residue after hydrotreating was obtained from China University of Petroleum (Qingdao, China). The sample of aromatics was prepared from Lungu atmospheric residue after hydrotreating through classic saturates/aromatics/resins/asphaltenes fractionation. The details for hydrotreating are described in an earlier report [19]. Before analysis, a little viscous aromatic residue was added in acetonitrile in the nebulization cell. Tangerine (Citrus reticulata) and grapefruit (Citrus paradisi) fruits were obtained from a local supermarket. For analysis, a piece of tangerine peel (5 mg) or grapefruit peel (5 mg) cut from fresh fruit was added to the nebulization cell separately without any pretreatment.
A soil sample was collected from the University of Science and Technology of China West Campus (Hefei, China). The spiked soil samples were prepared as follows (Supplementary Figure S1). First, the soil sample was pulverized with a grinding rod and sieved through an 80-mesh sieve to obtain fine powder. The powder was freeze-dried, and then 40 mg of the freeze-dried powder was mixed with a series of stock solutions of six polycyclic aromatic hydrocarbons (PAHs) dissolved in acetonitrile. After the solvent volatilized at room temperature completely, the soil powder was put in 4 mL of methanol/water (v:v = 1:1) in the nebulization cell for subsequent mass analysis. When detecting PAHs in soil, toluene/anisole (v:v = 1:1) was used as the dopant because of its high ionization efficiency [20]. The soil samples were treated with UNE for 0.5 min before analysis. Each experiment was repeated three times.
Stock solutions of hydroxylamine hydrochloride (6 mg/mL) and methyltestosterone (1.6 μg/mL) were prepared in methanol/water (v:v = 3:1). For the oximation reaction, first 3 mL of hydroxylamine hydrochloride and then 3 mL of methyltestosterone were added to the nebulization cell. Mass spectra were acquired continually throughout this process. The flow rate of carrier gas 1 was 40 mL/min.
EAPPI Mass Spectrometer
A schematic view of the EAPPI MS is shown in Fig. 1. The ultrasonic nebulization system contained an ultrasonic nebulizer (model 402AI; Yuwell Medical Equipment and Supply Corp., Suzhou, China), a glass nebulization cell, a dopant bubbler, and a heated transfer tube. The working frequency for the transducer of the ultrasonic nebulizer was 1.7 MHz ± 10%. The upper part (height 35 mm) of the glass nebulization cell had an i.d. of 16 mm and lower part (height 10 mm) had an i.d. of 8 mm. The bottom of the cell was covered with a thin polyethylene membrane that was transparent to ultrasonic waves, which was held in place by an O-ring. When the ultrasonic nebulizer was turned on, the sample solution in the nebulization cell could be transformed into aerosols by ultrasonic waves propagated from the transducer. The formed aerosol would be driven by carrier gas 1 through the heated transfer tube (i.d. 6 mm, o.d. 9 mm, length 60 mm) and mixed with the gaseous dopant, which was introduced from the bubbler through another tube (i.d. 6 mm, o.d. 9 mm, length 180 mm) by carrier gas 2. The outlet of the tube was only 1.5 mm (i.d.), and the confined gaseous sample was introduced to the ionization region. The entire transfer tube was kept at a constant temperature of 320 °C. Generally, for the analysis of components in complex matrices (e.g., soil and peel), a solid–liquid mixture should be treated by UNE for 0.5 min.
(a) Schematic of the EAPPI mass spectrometer(not to scale) with the following parts: 1, ultrasonic nebulizer; 2, water; 3, transducer; 4, O-ring; 5, membrane; 6, nebulization cell; 7, sample solution; 8, dopant; 9, heated transfer tube; and 10, VUV lamp. (b) Relationship between signal intensity and the flow rates of carrier gases 1 and 2. (c) Relationship between signal intensity and the drying gas flow rate. (d) Relationship between signal intensity and the distance between the lamp and MS inlet. (e) Relationship between signal intensity and the capillary voltage. (f) Mass spectrum of 10 μg/mL cholesterol (MW = 386.35) obtained by EAPPI with the VUV lamp off. (g) Mass spectrum of 10 μg/mL cholesterol obtained by EAPPI with the VUV lamp on
An Agilent 6224 Accurate-Mass TOF MS (Agilent, Santa Clara, CA, USA) was used to acquire high resolution mass spectra. Dopant-assisted APPI was performed using a krypton DC discharge VUV lamp (model PKS 106; Heraeus, Cambridge, UK), which was mounted on a moving stage beside the MS inlet and positioned orthogonally to the sample plume. Another gas (N2, 250 mL/min) was used to purge the surface of the VUV lamp to protect the MgF2 window and prolong its operational life (not shown). The drying gas originally used for desolvation of the ESI source was heated to 325 °C. Generally, 15 consecutive full scans from m/z 20 to 1000 at 1 spectrum/s were acquired and averaged. The calibration, acquisition, and analysis were completed using Mass Hunter Workstation software.
Results and Discussion
Parameter Optimization of EAPPI
To improve the signal sensitivity, parameters that affect the overall performance of EAPPI were optimized using a 1 μg/mL 9,10-phenanthrenequinone solution. According to the results of our previous study [17], the volume of sample solution, the distance between the membrane and transducer, and the temperature of the water were kept at 4 mL, 13 mm, and 30 °C, respectively, during the optimization procedures.
Figure 1b shows the dependence of the signal intensity at m/z 209 on the flow rates of carrier gases 1 and 2. The optimal flow rates of carrier gases 1 and 2 were 80 and 60 mL/min, respectively. Higher flow rate of carrier gas 1 will slightly reduce ion formation because of increased absorption of VUV light by excess solvent [21, 22]. The heated drying gas is crucial to the sensitivity, and taking both the sensitivity and stability into account, the optimal flow rate of the drying gas was 5 L/min.
Another parameter affecting APPI and APPI-related experiments is the distance between the VUV lamp surface and the MS inlet axis. As shown in Fig. 1d, this distance (c in Fig. 1a) was carefully tuned to 6.5 mm. The signal intensity was significantly reduced at shorter distances (by ~60% when c was 5 mm). At longer distances, the ion intensity would also decrease by virtue of the adsorption of VUV light by air. The optimum distance between the lamp axis and MS inlet surface was 12 mm (a in Fig. 1a). To minimize potential contamination, the spray tip was directed off-axis to the MS inlet [23], and the horizontal and perpendicular distances between them were 22 and 3 mm (b and d in Fig. 1a), respectively.
The capillary voltage applied on the MS inlet that can affect the ions transmission efficiency [24] was also studied. As shown in Fig. 1e, the optimum voltage range for 9,10-phenanthrenequinone solution was found from 1000 to 3500 V, which is similar to that reported for dopant-assisted APPI [25]. No ions were detected at voltages below 1000 V.
After optimization of the EAPPI experimental parameters, the contribution of the VUV lamp to the production of ions was further examined with cholesterol (10 μg/mL), which has low polarity. No ion signal was detected (Fig. 1f) unless the VUV lamp was turned on (Fig. 1g), indicating that cholesterol was ionized through photon-induced reactions rather than thermospray ionization, which is one of the primary ionization mechanisms in APPI [26]. Here, the limit of detection (LOD) of pure cholesterol was 0.1 μg/mL (m/z = 385.35, S/N = 53), which is better than that obtained by reactive DESI for cholesterol derivatives (1 μg/mL) [27].
Direct Analysis of Chemicals in Complex Matrices
As demonstrated above, the application of EAPPI for the analysis of solvent sample can be well implemented. UNE could quickly extract chemicals from a matrix and supply the desolvated aerosols for mass analysis. The feasibility of EAPPI for rapid screening of chemicals in various matrices was then investigated.
A commercially available compound amino acid capsule containing eight standard amino acids and several soluble vitamins was tested using EAPPI. A background subtracted mass spectrum was obtained of the components in the pellet (Fig. 2), and this shows [M + H – H2O]+ for L-threonine and [M + H]+ ions for the other seven amino acids. The vitamins, B1, B3, B5, B6, and C, were also detected, but isomers like leucine and isoleucine were indistinguishable. Detailed assignments of the observed ions (mass error ≤ 5 ppm) in Fig. 2 are listed in Supplementary Table S1. No pretreatment was conducted. Thus, EAPPI is more favorable than gas chromatography (GC)-MS for the qualitative analysis of amino acids. According to the results of previous publications [28, 29], derivatization is mandatory for GC-based analysis of amino acids and a relatively longer time (>8 min) was needed than EAPPI analysis (within 1 min).
Figure 3 shows the EAPPI mass spectra of tangerine (Citrus reticulata) and grapefruit (Citrus paradise) peels after UNE treatment. Below m/z 200, the peels showed very similar signals, which could be assigned to volatile terpenes. For example, the ion at m/z 136.12 was tentatively identified as monoterpene hydrocarbons. Flavonoids that are biologically active [30] and show great potential as anticarcinogens [31] were found in both peels, with peaks detected at m/z = 343.12, 373.13, 403.14, and 433.15. Detailed assignments (mass error ≤5 ppm) are given in Supplementary Table S2. The peak at m/z = 465.14 (Fig. 3b) was assigned as hesperetin 7-O-glucoside based on previously reported flavonoid profiles for Citrus grandis [32]. This is the first time that hesperetin 7-O-glucoside has been detected as a major constituent in grapefruit peel. Other striking signals at m/z 581.18 and 611.19 (Fig. 3b) were assigned to narirutin/naringin and hesperidin/neohesperidin, respectively. These assignments agree with a previous report of high-performance liquid chromatography diode array detection ESI MS [33].
EAPPI was also applied to the analysis of a viscous matrix. The spectrum of heavy oil after hydrotreating (Fig. 4) shows that components in heavy oil with low polarity and volatility were fully nebulized via UNE and photoionized. Relatively low molecular weight components of 200–450 Da with a center around 320 Da and high molecular weight components located around 520 Da were observed, which was also observed with laser-induced acoustic desorption/tunable synchrotron vacuum ultraviolet photoionization mass spectrometry [19, 34]. Elucidation of the structures and identification of the compounds present in the highly complex fractions derived from the petroleum residue was not possible because of the resolution of our mass spectrometer. The mean molecular weight (M n) can be estimated using the following equation:
where N i and M i are the signal intensity and molecular weight, respectively. The mean molecular weight was calculated to be 548 Da, which was comparable to that obtained by laser-induced acoustic desorption/tunable synchrotron vacuum ultraviolet photoionization mass spectrometry [19].
Assessment of EAPPI-MS for Quantification
Quantitative analysis of chemicals in complex matrices is still challenging with ambient mass spectrometry. According to the literature [35–37], methods such as DESI and DAPPI, which use a high pressure sheath gas, are not suitable for direct quantitative analysis of compounds in samples in powder form. To date, only several ambient ionization methods, such as desorption APCI [37] and low temperature plasma (LTP) [38] were applied to the quantification of compounds in powder matrices (powdered milk), and LTP is reported to have a dynamic range of more than two orders of magnitude [38].
The quantitative performance of EAPPI for matrices was demonstrated by determining the LOD, linearity, and repeatability for six PAHs in soil. Owing to their mutagenic and carcinogenic properties, these PAHs have been classified as priority pollutants by the United States Environmental Protection Agency [39] and the European Union [40]. Existing GC-MS methods for the PAHs in matrices such as soils, sediments, and air particulates are well established [41, 42]. However, time-consuming pretreatment and separation steps are usually needed. By comparison, EAPPI-MS of PAHs in various matrices is rapid (within 1 min).
Table 1 lists the LODs of six PAHs in soil with toluene/anisole (v:v = 1:1) as dopant. The detection limits (range 0.16–0.34 ng/mg) were much lower than PAH levels in areas with anthropogenic pollution [43, 44]. The results were an obvious improvement over those obtained with photoionization-based DAPPI (10 ng/mg) [14] and UNE-LPPI (10 ng/mg) [17]. Relative standard deviation (RSD) values of each substance in soil were in the range 7–12%.
Standard curves (Fig. 5) were constructed for the six PAHs in soil by analyzing samples with different concentrations without adding internal standards. The correlation coefficients (R 2) were all above 0.99, indicating good linearity of the method for compounds in complex matrices. Complete bias and imprecision (<20%) data for all six PAHs spiked in soil are shown in Supplementary Table S3. All these results suggest that EAPPI offers a robust alternative to existing methods for rapid quantitative assay of chemicals in matrices, without the need for sophisticated sample pretreatment.
Monitoring of Chemical Reactions in Real Time
Reaction monitoring is important in the chemical and pharmaceutical industries. Numerous mass spectrometry-based methods, including ESI [45, 46], DESI [47], DART [48], extractive ESI [49], LTP [50], electrospray-assisted laser desorption/ionization [51], and ultrasonic-assisted spray ionization [52], have been used for online monitoring of chemical reactions in real time.
The application of EAPPI to organic reaction monitoring was investigated for oximation accelerated by ultrasound irradiation [52]. Oximation is widely used to synthesize oximes, which are valuable chemical intermediates [53]. Here, methyltestosterone and hydroxylamine hydrochloride were selected as the reactants with the presence of acid as catalyst, and the corresponding reaction mechanism is shown in Scheme 1.
The EAPPI mass spectra of methyltestosterone/hydroxylamine hydrochloride in methanol/water (v:v = 3:1) at different reaction time are displayed in Fig. 6a. Each mass spectrum was an average of 15 consecutive scans. Initially, [M + H]+ ions of methyltestosterone at m/z 303 and its dehydrated product at m/z 285 dominated the mass spectrum. As the reaction time increased, the intensities of these peaks decreased gradually and the signals of the protonated product at m/z 318 and its dehydrated product at m/z 300 increased. The integrated peak areas were plotted as a function of reaction time (Fig. 6b). The time-evolved profiles of reactant and product can be clearly found.
(a) Mass spectra obtained by EAPPI at different times during the reaction. (b) Traces of protonated methyltestosterone (m/z 303, circles) and protonated product (m/z 318, squares) detected by monitoring the individual averaged signal intensities (15 consecutive analyses) on addition of 3 mL of hydroxylamine hydrochloride solution (6 mg/mL) to 3 mL of methyltestosterone solution (1.6 μg/mL) in the nebulization cell. The hexagons in (b) represent the recorded signal traces of protonated methyltestosterone for continuous analysis of 6 mL of methyltestosterone solution (0.8 μg/mL) for 12 min (each point represents the averaged ion intensity over 15 s, RSD = 6%)
Approximately 1.6 mL of solution was consumed over the 12 min reaction. To ensure that the decrease in solution volume and sample amount did not affect the signal intensity, spectra of 6 mL of pure methyltestosterone solution were collected continually for 12 min (Fig. 6b). The profile of pure methyltestosterone over this time was nearly constant, and changing the solution volume from 4 to 6 mL did not affect the signal intensity remarkably (Supplementary Figure S2). The signal intensity of methyltestosterone in the presence of hydroxylamine hydrochloride at the beginning of the reaction was lower than that of pure methyltestosterone, which may be due to the presence of hydroxylamine hydrochloride leading to ion suppression.
Conclusions
The ambient ionization method EAPPI, which combines UNE and APPI, was applied to rapid qualitative and quantitative analysis of chemicals in various matrices and online monitoring of organic reactions. Pretreatment and chromatographic separation steps are not required with this method, and the extraction and ionization process can be completed within 1 min. Semi/non-volatile chemicals with different polarities in capsules and plant tissues were effectively extracted, nebulized, and successfully analyzed by EAPPI. Viscous aromatics separated from petroleum residue obtained after hydrotreating were analyzed and its mean molecular weight was obtained. The robust quantification performance of EAPPI for chemicals in matrices was shown by the good linear relationship obtained for the detection of six priority PAHs spiked in soil powder. Moreover, the feasibility of EAPPI for online, real time monitoring of organic reactions was demonstrated by monitoring oximation reactions.
EAPPI is a promising method for rapid qualification and quantification of chemicals in complex matrices, and is an alternative method for online monitoring of organic reactions. EAPPI could be applied in food, drugs, herbs, forensics, environmental analyses, and other areas.
References
Alberici, R.M., Simas, R.C., Sanvido, G.B., Romao, W., Lalli, P.M., Benassi, M., Cunha, I.B., Eberlin, M.N.: Ambient mass spectrometry: bringing MS into the "real world". Anal. Bioanal. Chem. 398, 265–294 (2010)
Chen, H., Hu, B., Zhang, X.: Principle and application of ambient mass spectrometry for direct analysis of complex samples. Chin. J. Anal. Chem. 38, 1069–1088 (2010)
Huang, M.Z., Yuan, C.H., Cheng, S.C., Cho, Y.T., Shiea, J.: Ambient ionization mass spectrometry. Annu. Rev. Anal. Chem. 3, 43–65 (2010)
Takats, Z., Wiseman, J.M., Gologan, B., Cooks, R.G.: Mass spectrometry sampling under ambient conditions with desorption electrospray ionization. Science 306, 471–473 (2004)
Cody, R.B., Laramée, J.A., Durst, H.D.: Versatile new ion source for the analysis of materials in open air under ambient conditions. Anal. Chem. 77, 2297–2302 (2005)
Robb, D.B., Covey, T.R., Bruins, A.P.: Atmospheric pressure photoionization: an ionization method for liquid chromatography-mass spectrometry. Anal. Chem. 72, 3653–3659 (2000)
Harper, J.D., Charipar, N.A., Mulligan, C.C., Zhang, X., Cooks, R.G., Ouyang, Z.: Low-temperature plasma probe for ambient desorption ionization. Anal. Chem. 80, 9097–9104 (2008)
Ding, X., Zhan, X., Yuan, X., Zhao, Z., Duan, Y.: Microfabricated glow discharge plasma (MFGDP) for ambient desorption/ionization mass spectrometry. Anal. Chem. 85, 9013–9020 (2013)
Theron, H.B., van der Merwe, M.J., Swart, K.J., van der Westhuizen, J.H.: Employing atmospheric pressure photoionization in liquid chromatography/tandem mass spectrometry to minimize ion suppression and matrix effects for the quantification of venlafaxine and O-desmethylvenlafaxine. Rapid Commun. Mass Spectrom. 21, 1680–1686 (2007)
Haapala, M., Pól, J., Saarela, V., Arvola, V., Kotiaho, T., Ketola, R.A., Franssila, S., Kauppila, T.J., Kostiainen, R.: Desorption atmospheric pressure photoionization. Anal. Chem. 79, 7867–7872 (2007)
Vaikkinen, A., Shrestha, B., Kauppila, T.J., Vertes, A., Kostiainen, R.: Infrared laser ablation atmospheric pressure photoionization mass spectrometry. Anal. Chem. 84, 1630–1636 (2012)
Haapala, M., Teppo, J., Ollikainen, E., Kiiski, I., Vaikkinen, A., Kauppila, T.J., Kostiainen, R.: Solvent jet desorption capillary photoionization-mass spectrometry. Anal. Chem. 87, 3280–3285 (2015)
Kauppila, T.J., Flink, A., Haapala, M., Laakkonen, U.M., Aalberg, L., Ketola, R.A., Kostiainen, R.: Desorption atmospheric pressure photoionization-mass spectrometry in routine analysis of confiscated drugs. Forensic Sci. Int. 210, 206–212 (2011)
Luosujärvi, L., Kanerva, S., Saarela, V., Franssila, S., Kostiainen, R., Kotiaho, T., Kauppila, T.J.: Environmental and food analysis by desorption atmospheric pressure photoionization-mass spectrometry. Rapid Commun. Mass Spectrom. 24, 1343–1350 (2010)
Shiea, J., Chang, D.Y., Lin, C.H., Jiang, S.J.: Generating multiply charged protein ions by ultrasonic nebulization/multiple channel-electrospray ionization mass spectrometry. Anal. Chem. 73, 4983–4987 (2001)
Wang, L., Wang, Z., Zhang, H., Li, X., Zhang, H.: Ultrasonic nebulization extraction coupled with headspace single drop microextraction and gas chromatography-mass spectrometry for analysis of the essential oil in Cuminum cyminum L. Anal. Chim. Acta 647, 72–77 (2009)
Liu, C., Zhu, Y., Zhou, Z., Yang, J., Qi, F., Pan, Y.: Ultrasonic nebulization extraction/low pressure photoionization mass spectrometry for direct analysis of chemicals in matrices. Anal. Chim. Acta 891, 203–210 (2015)
Marchi, I., Rudaz, S., Veuthey, J.L.: Atmospheric pressure photoionization for coupling liquid-chromatography to mass spectrometry: a review. Talanta 78, 1–18 (2009)
Chen, J., Jia, L., Zhao, L., Lu, X., Guo, W., Weng, J., Qi, F.: Analysis of petroleum aromatics by laser-induced acoustic desorption/tunable synchrotron vacuum ultraviolet photoionization mass spectrometry. Energy Fuels 27, 2010–2017 (2013)
Smith, D.R., Robb, D.B., Blades, M.W.: Comparison of dopants for charge exchange ionization of nonpolar polycyclic aromatic hydrocarbons with reversed-phase LC-APPI-MS. J. Am. Soc. Mass Spectrom. 20, 73–79 (2009)
Kauppila, T.J., Bruins, A.P., Kostiainen, R.: Effect of the solvent flow rate on the ionization efficiency in atmospheric pressure photoionization-mass spectrometry. J. Am. Soc. Mass Spectrom. 16, 1399–1407 (2005)
Robb, D.B., Blades, M.W.: Effects of solvent flow, dopant flow, and lamp current on dopant-assisted atmospheric pressure photoionization (DA-APPI) for LC-MS. ionization via proton transfer. J. Am. Soc. Mass Spectrom. 16, 1275–1290 (2005)
Robb, D.B., Blades, M.W.: An electropneumatic-heated nebulizer for enhancing spray ionization in PhotoSpray atmospheric pressure photoionization sources for liquid chromatography/mass spectrometry. Rapid Commun. Mass Spectrom. 23, 3394–3400 (2009)
Himmelsbach, M., Haunschmidt, M., Buchberger, W., Klampfl, C.W.: Microemulsion electrokinetic chromatography with on-line atmospheric pressure photoionization mass spectrometric detection. Anal. Chem. 79, 1564–1568 (2007)
Takino, M., Daishima, S., Nakahara, T.: Determination of perfluorooctane sulfonate in river water by liquid chromatography/atmospheric pressure photoionization mass spectrometry by automated on-line extraction using turbulent flow chromatography. Rapid Commun. Mass Spectrom. 17, 383–390 (2003)
Robb, D.B., Blades, M.W.: State-of-the-art in atmospheric pressure photoionization for LC/MS. Anal. Chim. Acta 627, 34–49 (2008)
Wu, C., Ifa, D.R., Manicke, N.E., Cooks, R.G.: Rapid, direct analysis of cholesterol by charge labeling in reactive desorption electrospray ionization. Anal. Chem. 81, 7618–7624 (2009)
Kaspar, H., Dettmer, K., Chan, Q., Daniels, S., Nimkar, S., Daviglus, M.L., Stamler, J., Elliott, P., Oefner, P.J.: Urinary amino acid analysis: a comparison of iTRAQ®-LC-MS/MS, GC-MS, and amino acid analyzer. J. Chromatogr. B 877, 1838–1846 (2009)
Krumpochova, P., Bruyneel, B., Molenaar, D., Koukou, A., Wuhrer, M., Niessen, W., Giera, M.: Amino acid analysis using chromatography–mass spectrometry: an inter platform comparison study. J. Pharm. Biomed. Anal. 114, 398–407 (2015)
Abeysinghe, D.C., Li, X., Sun, C., Zhang, W., Zhou, C., Chen, K.: Bioactive compounds and antioxidant capacities in different edible tissues of citrus fruit of four species. Food Chem. 104, 1338–1344 (2007)
Xiao, H., Yang, C.S., Li, S., Jin, H., Ho, C.T., Patel, T.: Monodemethylated polymethoxyflavones from sweet orange (Citrus sinensis) peel inhibit growth of human lung cancer cells by apoptosis. Mol. Nutr. Food Res. 53, 398–406 (2009)
Yu, E.A., Kim, G.S., Lee, J.E., Park, S., Yi, S., Lee, S.J., Kim, J.H., Jin, J.S., Abd El-Aty, A.M., Shim, J.H., Shin, S.C.: Flavonoid profiles of immature and mature fruit tissues of Citrus grandis Osbeck (Dangyuja) and overall contribution to the antioxidant effect. Biomed. Chromatogr. 29, 590–594 (2015)
Zhang, M., Duan, C., Zang, Y., Huang, Z., Liu, G.: The flavonoid composition of flavedo and juice from the pummelo cultivar (Citrus grandis (L.) Osbeck) and the grapefruit cultivar (Citrus paradisi) from China. Food Chem. 129, 1530–1536 (2011)
Jia, L., Weng, J., Zhou, Z., Qi, F., Guo, W., Zhao, L., Chen, J.: Note: Laser-induced acoustic desorptionsynchrotron vacuum ultraviolet photoionization mass spectrometry for analysis of fragile compounds and heavy oils. Rev. Sci. Instrum. 83, 026105 (2012)
Leuthold, L.A., Mandscheff, J.F., Fathi, M., Giroud, C., Augsburger, M., Varesio, E., Hopfgartner, G.: Desorption electrospray ionization mass spectrometry: direct toxicological screening and analysis of illicit Ecstasy tablets. Rapid Commun. Mass Spectrom. 20, 103–110 (2006)
Luosujärvi, L., Laakkonen, U.M., Kostiainen, R., Kotiaho, T., Kauppila, T.J.: Analysis of street market confiscated drugs by desorption atmospheric pressure photoionization and desorption electrospray ionization coupled with mass spectrometry. Rapid Commun. Mass Spectrom. 23, 1401–1404 (2009)
Yang, S., Ding, J., Zheng, J., Hu, B., Li, J., Chen, H., Zhou, Z., Qiao, X.: Detection of melamine in milk products by surface desorption atmospheric pressure chemical ionization mass spectrometry. Anal. Chem. 81, 2426–2436 (2009)
Huang, G.M., Ouyang, Z., Cooks, R.G.: High-throughput trace melamine analysis in complex mixtures. Chem. Commun. 556–558 (2009)
Priority Pollutant List. Available at: http://www.epa.gov/sites/production/files/2015–09/documents/ priority-pollutant-list-epa.pdf. Accessed xxxx
Wenzl, T., Simon, R., Anklam, E., Kleiner, J.: Analytical methods for polycyclic aromatic hydrocarbons (PAHs) in food and the environment needed for new food legislation in the European Union. Trends Anal. Chem. 25, 716–725 (2006)
Vaz, J.: Screening direct analysis of PAHS in atmospheric particulate matter with SPME. Talanta 60, 687–693 (2003)
Poster, D.L., Schantz, M.M., Sander, L.C., Wise, S.A.: Analysis of polycyclic aromatic hydrocarbons (PAHs) in environmental samples: a critical review of gas chromatographic (GC) methods. Anal. Bioanal. Chem. 386, 859–881 (2006)
Masih, A., Taneja, A.: Polycyclic aromatic hydrocarbons (PAHs) concentrations and related carcinogenic potencies in soil at a semi-arid region of India. Chemosphere 65, 449–456 (2006)
Cai, Q.Y., Mo, C.H., Wu, Q.T., Katsoyiannis, A., Zeng, Q.Y.: The status of soil contamination by semivolatile organic chemicals (SVOCs) in China: a review. Sci. Total Environ. 389, 209–224 (2008)
Yan, X., Sokol, E., Li, X., Li, G., Xu, S., Cooks, R.G.: On-line reaction monitoring and mechanistic studies by mass spectrometry: Negishi cross-coupling, hydrogenolysis, and reductive amination. Angew. Chem. Int. Ed. 53, 5931–5935 (2014)
Santos, L.S.: Online mechanistic investigations of catalyzed reactions by electrospray ionization mass spectrometry: a tool to intercept transient species in solution. Eur. J. Org. Chem. 2008, 235–253 (2008)
Xie, Y., He, L.F., Lin, S.C., Su, H.F., Xie, S.Y., Huang, R.B., Zheng, L.S.: Desorption electrospray ionization mass spectrometry for monitoring the kinetics of Baeyer-Villiger solid-state organic reactions. J. Am. Soc. Mass Spectrom. 20, 2087–2092 (2009)
Petucci, C., Diffendal, J., Kaufman, D., Mekonnen, B., Terefenko, G., Musselman, B.: Direct analysis in real time for reaction monitoring in drug discovery. Anal. Chem. 79, 5064–5070 (2007)
Zhu, L., Gamez, G., Chen, H.W., Huang, H.X., Chingin, K., Zenobi, R.: Real-time, on-line monitoring of organic chemical reactions using extractive electrospray ionization tandem mass spectrometry. Rapid Commun. Mass Spectrom. 22, 2993–2998 (2008)
Ma, X., Zhang, S., Lin, Z., Liu, Y., Xing, Z., Yang, C., Zhang, X.: Real-time monitoring of chemical reactions by mass spectrometry utilizing a low-temperature plasma probe. Analyst 134, 1863–1867 (2009)
Cheng, C., Yuan, C., Cheng, S., Huang, M., Chang, H., Cheng, T., Yeh, C., Shiea, J.: Electrospray-assisted laser desorption/ionization mass spectrometry for continuously monitoring the states of ongoing chemical reactions in organic or aqueous solution under ambient conditions. Anal. Chem. 80, 7699–7705 (2008)
Chen, T.-Y., Chao, C.-S., Mong, K.K.T., Chen, Y.-C.: Ultrasonication-assisted spray ionization mass spectrometry for on-line monitoring of organic reactions. Chem. Commun. 46, 8347–8349 (2010)
Allen, C.L., Williams, J.M.: Metal-catalysed approaches to amide bond formation. Chem. Soc. Rev. 40, 3405–3415 (2011)
Acknowledgments
This work was supported by grants from the Natural Science Foundation of China (no. 91545120 and no. U1432128) and Chinese Universities Scientific Fund. The authors thank Professor Wenyue Guo of China University of Petroleum for providing the sample of aromatic petroleum residue after hydrotreating.
Author information
Authors and Affiliations
Corresponding author
Electronic supplementary material
Below is the link to the electronic supplementary material.
ESM 1
(DOCX 737 kb)
Rights and permissions
About this article
Cite this article
Liu, C., Yang, J., Wang, J. et al. Extractive Atmospheric Pressure Photoionization (EAPPI) Mass Spectrometry: Rapid Analysis of Chemicals in Complex Matrices. J. Am. Soc. Mass Spectrom. 27, 1597–1605 (2016). https://doi.org/10.1007/s13361-016-1445-6
Received:
Revised:
Accepted:
Published:
Issue Date:
DOI: https://doi.org/10.1007/s13361-016-1445-6