Abstract
Internal erosion refers to the mechanism of separation and movement of soil grains owing to water flow from a porous environment that may cause significant damage to the structures involved. In the present research, an environmentally friendly approach was used to improve the mechanical strength and erosion resistance of a poorly graded problematic sandy soil (SP) sample with a high degree of erodibility and negligible strength. For this purpose, polypropylene fibers (PP) (0, 0.35, 0.5 and 1%) were added to the studied sandy soil stabilized with different contents of clay (0, 5, 10 and 20%) and polyvinyl acetate (PVAc) resins (0, 1.2, 1.5 and 2%). The main goal of this study was to fully replace traditional stabilizers such as cement with durable and sustainable substitutes. The results revealed that the development in the strength of the samples by increasing the curing times was attributed to the time-dependent performance of PVAc content. Furthermore, the poorly graded sandy soil (PGSS) treated with PVAc and clay showed the highest mechanical strength equal to 2580 kPa after 28 days of curing. In terms of the Erosion Rate Index (ERI), the combination of 20% clay and 0.5% fiber along with 1.2% PVAc had the best performance, which was reported as 4.28 after 28 days. Among different samples reinforced by fiber, the highest ERI is related to the samples with 0.5% fibers at the 28-day curing time. The strength of the samples with clay inclusion was significantly higher than that of the samples with either PP fiber or PVAc inclusion, indicating the high potential of clay addition in developing the strength of the poorly graded sandy samples as confirmed by Scanning Electron Microscopy (SEM) analysis. SEM observations revealed that the treated sand sample became denser as the content of the amendments increased.
Similar content being viewed by others
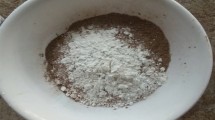
Avoid common mistakes on your manuscript.
Introduction
Constructing structures on problematic and poor soils, including poorly graded coastal soils, has many disadvantages (i.e., liquefaction and loss of shear strength) (Otoko 2014). In other words, poorly graded coastal sandy soils are known to have low compressive and shear strength and high settlement and erodibility potential (Al-Bared and Marto 2017), so they may cause serious problems and damage to different earth-hydraulic structures, such as the building foundation, irrigation canals, and roadbeds, during construction or exploitation of them and thereby leading to the structural failure or destruction and significant financial losses. Thus, reasonable preventive measures are required due to the existence of a vast area of sandy soils and the necessity of constructing civil and geo-environmental projects in such areas and sometimes non-cost-effective replacement of this type of soil (Bell 1993).
In order to overcome the problems associated with poorly graded sandy soil (PGSS), various soil improvement methods including soil stabilization and soil reinforcement have been widely used during these last decades (Maragos 1993; Bobrowsky and Marker 2018; Adabi et al. 2022). Lime, cement and gypsum are considered traditional stabilizers in the geotechnical engineering area. Irrespective of some advantages of these materials in increasing the soil strength and decreasing the soil erodibility (Mehenni et al. 2016; Salimi et al. 2018; Vakili et al. 2019, 2021), several drawbacks of these materials were also reported by researchers such as their harm to the environment (Kumari and Xiang 2019; Wang et al. 2022). For example, the production of each ton of cement releases approximately 1 ton of carbon dioxide gas into the atmosphere (Salimi and Ghorbani 2020). In addition, these traditional stabilizers bring brittle behavior or sudden failure for soil, which is undesirable (Kumar and Gupta 2016a; Jahandari et al. 2022).
Researchers have attempted new strategies to overcome the problems associated with traditional stabilizers. Many researchers added different types of fibers to cemented sandy soil (Liu et al. 2020a; Zhao et al. 2020). By following this approach, the failure pattern of soil typically changes from brittle behavior to a more ductile one (Hamidi and Hooresfand 2013). In addition, the reinforcement by different types of fiber strands such as polypropylene and palm fibers improves Unconfined Compressive Strength (UCS), California Bearing Ratio (CBR), peak and residual shear strength, mechanical properties, and stability and controls the erodibility and liquefaction potential of sandy soils (Wang et al. 2000; Marandi et al. 2008; Consoli et al. 2010; Hejazi et al. 2012; Liu et al. 2020b; Zhao et al. 2020). In the soil reinforcement method by fibers, fiber content, fiber length, curing time, curing method, and fiber distribution method, have been found as significant variable parameters controlling the soil behavior (Ghiassian and Poorebrahim 2005; Park 2009; Bozyiğit et al. 2017; Karakan 2018). It should be noted that better curing conditions can form better interlocking zones around the fiber strands, resulting in stronger bonding of the particles and improving fiber efficiency.
However, in the last decade, other researchers have attempted to replace traditional stabilizers like cement with environmentally friendly stabilizers such as nanosilica, lignosulfonate, polymers, various industrial wastes, and polyvinyl acetate (PVAc), partially or entirely (Yu et al. 2016). In all previous studies, the type of stabilizers, stabilizer contents, curing times, and curing methods significantly determine the soil characteristics (Anagnostopoulos 2015). Anagnostopoulos (2015) assessed the strength properties of epoxy resin-stabilized silty clay soil considering different curing times. The results of the study indicate that most of the epoxy resin-treated soil, even with the adverse influence of water content on the development of strength, appeared to have noticeable strength improvement after a curing period of 90 days. Some previous studies evaluated the effect of natural materials such as clayey soils and natural pozzolans on cement-treated sandy soils (MolaAbasi et al. 2020). It was found that these materials can play essential roles in stabilization procedures.
It should be noted that due to the size of its fine particles, clay can be placed between sand particles and fill the holes between sand grains, which can lead to the physical improvement of sandy soil. In such conditions, the performance of the fibers is much better due to the formation of better locking zones formed with the help of clay grains. In addition, clay particles can participate in chemical reactions due to their minerals and hence improve the properties of sandy soil. To cite an example, Wang et al. (2018) investigated the effects of coal-metakaolin (CMK) on the mechanical and microstructural properties of cemented sandy soil. The results showed that CMK can accelerate cement hydration, modify the pore structure, and improve the interfacial zone between soil particles and binder, due to its pozzolanic nature and fine particle size.
Recently, the investigators have attempted to examine the characteristics of the soil treated with environmentally friendly stabilizers and reinforced by polypropylene (PP) fibers. In these new researches, cement was completely removed from stabilization procedures and stabilization methods by environmentally friendly materials and reinforcement by various types of fibers have been taken into account simultaneously. A new method of soil improvement using both discrete PP fibers and PVAc was introduced by Masoumi et al. (2013). Based on the results from CBR tests on various specimens, it has been revealed that the combination of PP fiber and PVAc resin with suitable weight percentages had the optimum effect in increasing the CBR value in both saturated and dry soil specimens. Vakili et al. (2018) have also introduced a novel soil improvement technique using the lignosulfonate and PP fibers as stabilizer and reinforcement materials, respectively. They reported that the internal erosional and mechanical behavior of highly dispersive soil were significantly improved due to the simultaneous application of lignosulfonate and PP fiber. In other words, instead of cement, lignosulfonate or PVAc resin in the abovementioned studies was used as chemical binders to increase the adhesion between fiber strands and soil particles.
One of the advantages of PVAc compared to other additives is its easy production. This material has the property of forming an excellent membrane, which with its network structure can not only create the bonding and tensile forces between soil particles but also increase the friction angle and cohesion of soils. Furthermore, it is non-toxic and does not cause environmental pollution. It can efficiently be modified to achieve a certain level of performance (Bu et al. 2019; Sun et al. 2022).
As reported above, the main aim of the majority of the research done on PGSS was to investigate the mechanical strength of the samples. Therefore, less attention has been taken into consideration regarding the erosional behavior of PGSS after being stabilized or/and reinforced. Soil erosion means the continuous destruction of soil and its transfer or movement from one point to another through external factors such as water. It is a process by which fine-grained particles are locally moved into a coarse-grained layer, and the created holes can cause breakage and destruction. Piping and internal erosion cause many problems for earthen structures, especially earth dams. The gradual transport of soil particles, which in the developed state causes the creation of a waterway in the earthen structure, can provoke internal erosion in the earthen structures and ultimately lead to their destruction. To prevent possible construction problems caused by internal erosion, early identification of this type of soil and finding suitable solutions to deal with them are vital. Recently, some studies have been conducted on the effects of internal erosion on the mechanical and geotechnical characteristics of soils (Yang et al. 2018, 2020, 2021; Cai et al. 2022). It must be noted that PGSSs have been known to have not only negligible mechanical strength but also very low erosion resistance. Hence, in addition to examining the mechanical strength of the soil, its erosional behavior is also required to be tested using widely accepted experiments such as Hole Erosion Test (HET), firstly introduced by Wan and Fell (2004).
In this study, a new improvement strategy, involving the stabilization by natural clayey soil and PVAc resin and reinforcement by PP fiber was used to improve the mechanical strength and erosion resistance of poorly graded problematic sandy soils. Although the efficiency of the combined use of polymers with fibers in increasing the mechanical strength of PGSSs has been addressed in the literature (Liu et al. 2017, 2018; Bai et al. 2019), detailed studies on simultaneous applications of PVAc resins and PP fiber along with clay in mitigating the erodibility character of poorly graded problematic sandy soils are still lacking which has been tried to be addressed in this study. To this end, in the present study, the effects of different curing times and weight percentages of reinforcing elements and stabilizers on erosional behavior and mechanical properties of poorly graded coastal sandy soils were investigated.
Materials and methods
Materials
The sandy soil used in this study was collected from the site in Bandargah Village, located 5 km from the center of Bushehr, Bushehr Province, Iran and at 50° 54′ 54.61″ E and 28° 49′ 25.74″ N. Figure 1 (an aerial image) shows the geographic location of the soil. The provided sandy soil was initially subjected to particle size distribution (PSD) test by following ASTM D 422–63 (2007). The PSD curve of the soil is shown in Fig. 2. The studied soil with a coefficient of curvature (Cc) of 0.88 and a coefficient of uniformity (Cu) of 2.82 was classified as PGSSs (SP) based on the Unified Soil Classification System (USCS). Subsequently, following the ASTM D 4253 (2014) and ASTM D 4254 (2014), the maximum and minimum dry densities of the studied soil were measured as 1.75 and 1.47 g/cm3, respectively.
As a stabilizer, clayey soil was provided from Estahban, Iran. Note that the core material of Rodbal Dam was also supplied by the same area. The clayey soil was subjected to the PSD test as per ASTM D 422–63 (2007), and Atterberg limits test according to ASTM D 4318 (2005). Figure 2 shows the grading curve of used clay. In addition, the liquid limit, plastic limit and plasticity index of the clay were measured as 38, 12, and 26%, respectively (Table 1). Thus, the clayey soil was classified as low plasticity clay or CL based on USCS. The clayey soil was added to the sandy soil at 5, 10, and 20% by dry weight of the sandy soil. Furthermore, the Optimum Moisture Content (OMC) and the Maximum Dry Density (MDD) of clay are 20% and 1.64 g/cm3, respectively, as per ASTM D 698 (2012).
The 12 mm transparent PP fiber was used as a reinforcement material in this study. Selecting PP fiber with a length of 12 mm was in agreement with the previous studies where the most appropriate soil characteristics were obtained due to the inclusion of 12 mm fiber compared to other fiber lengths (Yilmaz et al. 2015; Kumar and Gupta 2016). The physical properties of the fibers used in the current research are given in Table 2. In this study, the PGSS samples were reinforced with various PP fiber content including 0.35, 0.5, and 1% of the dry weight of the soil. These contents for fibers have been selected based on the suggestions of past studies (Tang et al. 2007; Tran et al. 2018; Xiao et al. 2022), which stated that the optimum fiber content is in the range of 0 to 1%. It should be noted that fiber contents higher than 1% lead to the accumulation of fiber strands and result in practical difficulties.
As a stabilizer, white PVAc with a chemical formula of (C4H6O2) and unit weight of 1.05 g/cm3 was used in this study. PVAc is known as a non-flammable and non-toxic substance, it is not washed with water, contributes to soil stability and reduces soil erosion. Moreover, polymer molecules act as a bridge between soil particles and form linkages between them. Based on past studies (dos Santos et al. 2021; Cherifi et al. 2021), PVAc is a low-cost and environmentally friendly material due to its components. It is also used in various industries such as pharmaceuticals, cosmetics, food packaging, construction and textiles. The PVAc used in this study was liquid and its physical properties are given in Table 3, provided by the manufacturing company (Bell Co.). The PVAc was added to the PGSS samples at 1.2, 1.5 and 2% by dry weight of the sandy soil. The PVAc contents were determined by trial and error, which were within the range of PVAc contents in previous research (Homauoni and Yasrobi 2011).
Sample preparation and methodology
In this study, untreated soil was prepared at a relative density of 75%, based on which the density of the soil was obtained as 1.67 g/cm3. Choosing a relative density of 75% in testing programs can be considered appropriate because a relative density of between 70 to 85% is normally utilized in many construction projects in fields involving sandy soils (Memar et al. 2020). Then, this amount of density (i.e., 1.67 g/cm3) was considered constant during the test for all treated samples. In other words, to eliminate the effects caused by changes in density for different samples, a density of 1.67 g/cm3 was considered for preparing all samples, but the compression energy was distinct for them. This made it possible to determine the effect of additives on soil characteristics regardless of the effect of densities. Moreover, the water content used for samples without clay was equal to the amount of water required for compaction (equivalent to 5% of sand weight). But in the samples containing clay, the water content for sample preparation (Ww) was calculated from the following equation:
and
where Wsand and Wclay are the weight of sand and clay, respectively, \({{\rm W}}_{{\rm Opt }({\rm Clay})}\) is the weight of optimum water content for clay and \({{\rm OMC}}_{{\rm clay}}\) is equal to 20% for studied clay in this study.
To prepare modified sandy soil samples, different amounts of amendments were added to sandy soil according to Table 4. It should be mentioned that to prepare the samples containing PVAc, the required water for each composition was added to it and the final emulsion was added to the soil. To prepare samples containing PP fibers, the specified fibers were mixed with sandy soil in a dry state, and then the rest of the additives and water were added to it. All composites were mixed well to obtain a homogeneous mixture. After that, it was kept in closed plastic bags for 24 h in accordance with (Zhu et al. 2022) and then compressed in the UCS mold with a diameter of 40 mm and height of 87 mm in 3 layers with a dry density of 1.67 g/cm3. Note that each layer was compacted 25 times using a 1 cm diameter rod and then the top surface of the layer was scratched before compacting the next layer (Gerard et al. 2015). Subsequently, the compacted soil samples were extracted from the mold, wrapped in 3 layers of airtight plastic bags, and cured at the ambient temperature, i.e., 25 °C, for various time intervals including 1, 3, and 28 days (Vakili et al. 2022).
Unconfined compression test
In this study, the effects of clay content, PP content, PVAc content, and curing period on the mechanical characteristics of the PGSS samples were investigated by carrying out the UCS test as per ASTM D 2166 (2000). Note that each UCS test was repeated 3 times on the identical soil-additive samples and the results reported in the current research were the mean value of those 3 tests. Thus, a total of 108 samples, with the aforementioned weight percentages, were tested at three curing times. It must be noted that the UCS of the untreated sandy soil provided for the study was considered negligible because of its very low cohesion.
Hole Erosion Test (HET)
The hole erosion test (HET) was first performed by Wan and Fell (2004) in order to simulate piping erosion and also assess soil erosion parameters. They found that HET is a suitable approach to quantify the erosion rate in concentrated leaks in soil. The method of conducting this test is generally that first the studied soil is compressed inside a mold and then an axial hole is created along the soil sample. Then, by installing an upstream and downstream tank and creating a water level difference in them, a flow is established through this hole drilled in the sample. As the flow passes, the diameter of the hole gradually increases with time due to the erosion process. During the test, the flow rate and hydraulic head of the upstream and downstream of the sample are measured, and the final hole diameter is calculated at the end of the test. Wan and Fell (2004) showed that the erosion rate per unit surface area of the hole (\(\dot{{\varepsilon }_{t}}\)) is measured using the following equation:
where \({\rho }_{d}\) is the dry density of the soil (kg/m3) and \({\phi }_{t}\) is equal to the diameter of the hole at time t, which can be measured from Eqs. 2 (laminar flow conditions) and 3 (turbulent flow conditions) depending on the type of flow:
where \({Q}_{t}\) (m3/s) is the flow rate at time t, \({\rho }_{w}\) is the density of the eroding fluid (kg/m3), g is the acceleration due to gravity (9.8 m/s2), \({s}_{t}\) is the hydraulic gradient across the soil sample at time t and \({f}_{Lt}\) (kg/m2/s) and \({f}_{Tt}\) (kg/m3) are defined as friction factors relating shear stress to the mean flow velocity. Wan and Fell (2004) proposed an equation for the Erosion Rate Index (ERI) as follows:
where \({C}_{e}\), coefficient of soil erosion, is defined as the slope of the line of best fit in Eq. 1.
In this study, the effects of clay content, PP content, PVAc content, and curing time on the erosion rate of the PGSS samples were investigated by carrying out the HET based on the method designated by Wan and Fell (2004). After preparing the treated sandy soil specimens by the method described earlier, the soil specimens were compressed inside the mold of the HET device with a diameter of 100 mm and a height of 120 mm. Note that the soil samples were compacted in 3 layers with the compaction energy equivalent to reaching a density of 1.67 g/cm3. Thereafter, the mold of the HET device containing the compacted soil samples was covered by airtight plastic bags and the compacted soil sample was kept in the mold for 4 h. Next, the sample preparation was done by punching a hole with a diameter of 6 mm from its longitudinal direction. Subsequently, the mold of the HET device containing the prepared samples was put in airtight plastic bags and the prepared samples were cured at the ambient temperature for various curing periods including 1, 3 and 28 days. After completing the predetermined curing time, the mold was removed from the plastic bags and placed in the HET device. Note that the HET was done on each sample in 3 heads including 50, 200, and 400 mm. By performing the HET, the Erosion Rate Index (ERI) of different samples was calculated and finally, the qualitative classification of the samples in terms of erodibility was also done by following Table 5 as described by Wan and Fell (2004).
To check the repeatability and precision of the results, each HET test was repeated 2 times on the same soil samples and the average of the obtained results was reported as the final value. In other words, a total of 216 samples, with the aforementioned weight percentages, were tested at three curing times and heads.
Table 4 gives the influential parameters of the samples controlling the testing programs. In addition, to control the simultaneous effects of all three additives on the characteristics of the PGSS samples, the investigations were arranged to be possible to control the effects of only two additives, resulting in measuring the removal influence of the third additive on both the mechanical strength and erosion resistance. Finally, to find the improvement mechanisms followed in the current study, the microstructure of the untreated and treated sandy soil was analyzed by Scanning Electron Microscopy (SEM) test. To this end, the specified samples (i.e., I) poorly graded sand, sand treated with II) 1.2% PVAc and 20% clay, III) 1.2% PVAc and 0.5% PP fibers, IV) 20% clay and 0.5% PP fibers, and V) 1.2% PVAc, 20% clay and 0.5% PP fibers after 28 days of curing) were fractured to create freshly exposed surfaces for the SEM test. Images of the samples were magnified 3000 times using a scanning electron microscope modeled MIRA3-TESCAN by Beam Gostar Taban Co., Tehran, Iran.
Results and discussions
Effect of different weight percentages of PP fibers on the internal erosion and UCS of poorly graded sand
Figure 3 shows the variations of the UCS of the PGSS samples treated with constant 1.2% PVAc content, 20% clay content, and different PP fiber contents and curing times. The UCS of the PGSS sample increased from almost zero to about 539 kPa after a day of curing due to a treatment of the sample by clay and PVAc. Moreover, the UCS growth of the samples was significantly persisted due to fiber inclusion. The strength of the samples increased by increasing the fiber content from 0 to 0.5%, whereas the strength decreased owing to increasing the fiber content from 0.5 to 1%.
It is worth noting that this reduction in UCS in the sample containing excessive fiber can be attributed to the lubrication of the fibers (Lawer et al. 2021). This means that due to the excessive accumulation of fiber strands, they come into contact with each other and cause them to slide more easily on each other. In this case, the main interactions are fiber-to-fiber instead of fiber-to-soil, which causes a decrease in UCS (Jiang et al. 2022).
Furthermore, another factor that can be attributed to this decrease in the UCS of the samples is fiber entanglement, which occurs when too much fiber is added. As a result, the lack of sufficient links between strands and soil particles in some areas of the samples leads to a decrease in their strength (Vakili et al. 2018). Therefore, the highest compressive strength was obtained by adding 0.5% fibers to the soil and this was true for all three curing times.
In addition, the curing time plays a significant role in increasing the compressive strength of the sample due to the acceleration of chemical reactions and the formation of stronger interlocking zones, so that its value increases over time. It should be noted that the presence of clay particles as well as PVAc in the composition makes this sample very sensitive to time due to the ability of these additives to participate in the reactions with time. In the optimum state (adding 0.5% fiber), the compressive strength of the sample at the 28-day curing time was 1.53 and 2.15 times higher than its value at the 3-day and 1-day curing times, respectively. In general, the inclusion of PP fibers in the soil causes the fibers to be placed between the particles, which prevents the formation of tensile cracks during loading. In other words, the presence of fibers can increase the friction between particles and keep them together. Therefore, the presence of these interlocking areas around the fiber strands leads to a stronger bonding of the particles (Vakili et al. 2022).
To investigate the performance of PP fiber inclusion on the strength of the PGSS samples treated with clay and PVAc content over time, the new R ratio parameter was defined and calculated in this study, as given in Fig. 4. In this case, the R ratio was defined as the strength of the samples reinforced by PP fiber to that of the same samples without fiber. The highest R ratio was obtained for the sample with 0.5% PP fiber, however, the R ratio remained approximately constant for the samples reinforced either by 0.35 and 1% fiber. Thus, 0.5% fiber content can be considered as a threshold or fiber fixation point. The R ratio ranged between 1.06 and 2.21, reflecting the highest value of fiber inclusion in improving the mechanical strength of the samples when the added fiber content was optimum, i.e., 0.5% in this study. However, insignificant effects on UCS were recorded when the fiber content used in this study was either above or below the threshold. Decreasing the strength of the sample reinforced with the fiber content more than the threshold can be ascribed to the “lubricating” of the PP fibers and/or fiber entanglement, resulting in inadequate links between soil particles and fiber strands (Chen et al. 2015; Vakili et al. 2018).
In this case, the most important finding of the current study can be attributed to the variations of the R ratio with increasing the curing time. For any given PP fiber content, the R ratio value was more than 1 on the first day of curing, demonstrating the positive effects of fiber inclusion in improving the strength of the samples. However, the R ratio revealed a decreasing trend by increasing the curing times, reflecting that the curing time possesses an insignificant role in the case of samples reinforced by PP fiber. In other words, the fiber inclusion was found more effective than the curing time.
Figure 5 shows the variations of the erosion rate indices of the PGSS samples treated with constant 1.2% PVAc, 20% clay, and different weight percentages of PP fiber content after various curing times. The ERI of the PGSS sample increased from less than 2 for the untreated sample (soil alone) to 3.7 for the sample treated with 1.2% PVAc and 20% clay content after 1 day of curing. Thus, the qualitative classification of the sample in terms of erodibility changed from extremely rapid for the untreated sample to moderately rapid for the treated sample. After 28-day curing, the category of the sample upgraded to moderately slow in terms of erodibility, indicating an acceptable erosion resistance of the improved sample. Thus, the combination of clay and PVAc was found appropriate for improving the mechanical behavior of the PGSSs and increasing the erosion resistance of the samples to an acceptable level.
Fiber inclusion caused an increase in the ERI of the sample stabilized by clay and PVAc. As seen in Fig. 5, the ERI of the samples increased by increasing the fiber content up to 0.5%. However, the ERI decreased with increasing the fiber content from 0.5 to 1%. Therefore, the highest ERI and thus, the lowest erodibility was obtained by adding 0.5% fiber. The erodibility class of the sample treated with 0.5% fiber, 1.2% PVAc, and 20% clay was determined as moderately slow even after a day of curing. Although the qualitative classification of the mentioned sample was not changed and the ERI of the samples remained between 4 and 5 for the additional curing times, the results show that the erosion rate of the samples decreased over time.
Effect of different weight percentages of PVAc on the geotechnical properties of poorly graded sand
Figure 6 shows the variations of the UCS of the PGSS samples treated with constant 0.5% PP fiber, 20% clay, and different weight percentages of PVAc content and cured for various days. The UCS of the PGSS sample increased from almost zero for the untreated sample to about 485 kPa for the treated sample by 0.5% PP fiber and 20% clay content only after 1 day of curing. It must be noted that the UCS of the one-day cured PGSS samples treated with 1.2% PVAc and 20% clay content was determined as 539 kPa. Thus, the PGSS improved by a combination of PVAc and clay showed a better mechanical strength than the sample improved by a combination of PP fiber and clay.
Moreover, by increasing curing days, the strength increment rate of the samples treated with a combination of PVAc and clay was recorded as much higher than that of the samples improved by a combination of fiber and clay. To give evidence, the strength of the sample treated with clay and PVAc was determined as 1.11 times more than that of the sample treated with clay and fiber after 1 day of curing. However, after 28 days of curing, the strength of the clay-PVAc treated sample was 1.35 times higher than that of the clay-fiber treated sample. In addition, for any given clay content, i.e., 20%, the PVAc-treated soil performed better than PP fiber-reinforced soil.
Generally, the mechanism that acts on the soil by PVAc and improves its mechanical properties includes a series of chemical and physical reactions depending on the soil type. This type of additive can solidify the sand by forming new bonds with the surface of the sand particles. In sandy soils, the dominant process is physical bonds, and therefore electrostatic attractions between hydrogen atoms and atoms with strong electronegativity have the most significant effect during curing time. Furthermore, physical reactions between PVAc and sand particles create a superior network membrane structure with elasticity and glutinousness. This caused the pores between the sand particles to be filled. In this situation, the sand particles stick together well and the strength of the soil increases significantly due to the effect of reticulated membranes. On the other hand, in the samples containing clay, chemical reactions can have a greater impact on the properties of the soil. In this case, the thickness of the electric double layer becomes thinner when the cations on the surface of the soil particles are exchanged; thereby, the attraction between soil particles and aggregation increases (Bu et al. 2019; Huang et al. 2022).
As shown in Fig. 6, the UCS increased by increasing the PVAc content up to 1.2%, thereafter, it decreased due to the addition of more PVAc content. It should be noted that PVAc is used in the samples to increase the interfacial friction between the soil particles (Bu et al. 2019; Sun et al. 2022). So, the PVAc content higher than a certain threshold will not be suitable because it fills the gaps between the grains. Since the PVAc has lower strength than the soil grains, if the voids are filled with resin, it leads to a decrease in the strength of the samples (Masoumi et al. 2013). Thus, the highest strength was recorded for the sample stabilized by 1.2% PVAc. The pronounced potential of PVAc in increasing the mechanical strength of the samples can be linked to the enhanced bonding between soil particles due to filling the voids by this liquid stabilizer (Rezaeimalek et al. 2017).
The effects of PVAc content on the UCS growth of the samples improved by PP fiber and clay were also assessed by the R ratio over time. In this case, the R ratio was defined as the strength of the samples treated with PVAc content to the strength of the same samples without PVAc content. As given in Fig. 7, the highest and lowest R ratios were 0.96 and 2.86, respectively. Based on the results, the PVAc fixation point was determined as 1.2%. The addition of 2% PVAc content not only did not enhance the strength of the samples but also decreased UCS. Unlike the variation of the R ratio with increasing the curing time in the case of fiber reinforcement, the curing time plays a significant role in increasing the R ratio of the sample treated by PVAc, so that its value increases over time. Consequently, the strength development of the samples improved by clay, PVAc, and PP fiber with increasing the curing time can be linked to the existence of PVAc. In the optimum state (adding 1.2% PVAc), the compressive strength of the sample at the 28-day curing time was 1.52 and 2.155 times its value at the 3-day and 1-day curing times, respectively.
Figure 8 shows the variations of the erosion rate indices of the PGSS samples treated with constant 0.5% PP fiber, 20% clay, and different weight percentages of PVAc content and cured for various days. The ERI of the PGSS sample increased from less than 2 for the untreated sample to 4.09 for the sample treated with 0.5% PP fiber and 20% clay content after 1 day of curing. Thus, the qualitative classification of the sample in terms of erodibility changed from extremely rapid for the untreated sample to moderately slow for the treated sample. In terms of erosion resistance, the combination of clay and fiber performed better than the combination of clay and PVAc. Note that the classification of the clay-treated sample containing fiber was shifted into moderately slow only after 1 day of curing, while for the clay-treated sample containing PVAc, the required curing time to achieve the same classification was 28 days.
As shown in Fig. 8, the ERI of the samples increased with increasing the PVAc content up to 1.2%, after that, the trend reversed with increasing the PVAc content. Therefore, the highest ERI and thus, the highest erosion resistance was obtained for the sample stabilized by 1.2% PVAc content. In addition, the results also show that the ERI of the samples increased over time. The erosion rate indices of the sample stabilized by 1.2% PVAc were determined as 4.11, 4.15 and 4.28, respectively after 1, 3, and 28 days of curing.
Effect of different weight percentages of clay on the geotechnical properties of poorly graded sand
Figure 9 shows the variations of the UCS of the PGSS samples treated with constant 1.2% PVAc, 0.5% PP fiber, and different weight percentages of clay content and cured for various days. The UCS of the poorly graded sandy sample increased from almost zero to about 184 kPa after a day of curing due to the treatment of the sample with 1.2% PVAc and 0.5% PP fiber. Although the combination of PVAc and PP fiber increased the strength of the untreated sample even after a day of curing, the combination of PVAc and clay and the combination of PP fiber and clay performed a better role in increasing the mechanical strength of the samples than the combination of PVAc and PP fiber did, reflecting the significant effects of clay addition on the strength of the poorly graded sandy sample.
As shown in Fig. 9, the UCS of the samples increased gradually by increasing the clay content from 0 to 10%, thereafter, a significant increase in the strength was noticeable due to further increasing the clay content from 10 to 20%. To investigate the effects of clay treatment on the UCS growth of the samples improved by PVAc and PP fiber over time, the R ratio was also measured in this step of the study. The R ratio, in this case, was defined as the strength of the samples treated with clay content to that of the same samples without clay content. The range of the R ratio which was between 1.46 and 6.49 again indicated the high potential of clay addition in developing the strength of the poorly graded sandy samples.
In other words, the R ratio due to clay inclusion was significantly higher than the R ratio due to either PP fiber or PVAc inclusion. Although the difference between the strength of the samples treated with 20% clay was notable in comparison to the strength of the samples treated with 5 or 10% clay content, the variation of R ratio versus curing times for all these samples was governed by the same decreasing trend, as shown in Fig. 10.
Figure 11 shows the variations of the erosion rate indices of the PGSS samples treated with constant 1.2% PVAc, 0.5% PP fiber, and different weight percentages of clay content and cured for various days. The ERI of the PGSS sample increased from less than 2 for the untreated sample to 3.9 for the sample treated with 1.2% PVAc and 0.5% PP fiber content after 28-day curing. Thus, the qualitative classification of the sample in terms of erodibility altered from extremely rapid for the untreated sample to moderately rapid for the 28 days cured sample. The qualitative classification of the same sample after 1 day and 3 days of curing were determined as extremely rapid.
Although the ERI of the samples treated by the combination of fiber and PVAc increased by increasing curing days, this combination was found incapable of certain improving the sample from an erosion resistance viewpoint. It seems that the clay content plays a significant role in this case. In short curing time, for any given PVAc content, i.e., 1.2%, the ERI of the sample treated with clay content was about 2.16 times higher than that of the sample reinforced by fiber content. However, in a long curing time, the ERI of the sample treated with clay was about 1.035 times more than that of the sample reinforced by fiber. Therefore, the erosion resistance of both samples i.e., the sample treated by a combination of clay and PVAc and another one treated by fiber and PVAc was approximately measured equal, reflecting the significant performance of PVAc in long curing times.
As shown in Fig. 11, the ERI of the samples increased by increasing the clay content up to 20%. Therefore, the highest ERI and thus, the highest erosion resistance was obtained for the sample stabilized by 20% clay content. In addition, the results also show that the curing time has a different role in increasing the erosion resistance of the samples treated with various clay content, although the ERI of the samples increased over time. For low clay content, i.e., 5%, the ERI of the sample was determined as 1.9, therefore the sample appointed the extremely rapid classification in terms of erodibility. However, the ERI of the sample increased significantly to 4 after 28 days of curing and thus, its classification upgraded into moderately slow, reflecting the significant impact of long curing time in improving the sample against erosion when the clay content is low.
For the sample treated with 10% clay, the acceptable qualitative class in terms of erodibility, i.e., moderately slow, was achieved only after 3 days of curing. The erosion rate indices of the samples stabilized by 20% clay were determined as 4.11, 4.15, and 4.28, respectively after 1, 3, and 28 days of curing. It demonstrated that the erodibility class changed quickly from extremely rapid to moderately slow when the clay content is high.
Note that the soil classification based on USCS was also altered due to clay treatment. The initial classification of the untreated soil was SP. However, its class shifted to SP-SC due to the addition of 5–12% clay. By using 20% clay (> 12%) in the treatment of the PGSS samples, the new soil class, i.e., SC, was made. The results of the previous studies revealed that by increasing the clay content the erosion resistance of silty and sandy soils increased (Mehenni et al. 2016) and this trend was also found valid in this study for PGSS samples.
Microstructural analysis of the untreated and treated sandy soil
Figure 12 shows the SEM micrographs of various stabilized and reinforced samples after 28 days of curing at magnifications of 3000. As can be seen in Fig. 12a, natural sand had a discontinuous and open texture. The gaps between the particles have caused this soil with its weak structure to show no resistance. However, with the addition of chemical additives and PP fibers, soil structure has changed and become denser, especially in samples containing clay, PVAc, and PP fibers (Fig. 12e).
As can be seen, the compounds of clay and polyvinyl create a strong structure in the sand, and this enhances the behavior of the sample that can interlock the sand grains. Comparing the SEM results, it is clear that the samples containing clay have a much better system than other additives. Due to the size of the clay particles, they have been able to fill the gaps between the sand grains and participate well in the reactions (Shaikh 2021). In addition, the presence of clay in the sample has improved the bonding between particles and fibers.
It should be noted that the inclusion of PVAc in the compounds has improved the mechanical strength of the samples in the presence of clays. In other words, the adhesion between particles is evident in samples containing PVAc, which holds the particles well together, even with fiber strands. Furthermore, incorporating the randomly distributed fibers among the sand grains, they are connected to form a spatial network structure. By and large, the stabilized and reinforced sand sample became denser as the content of the amendments increased, making it a harder material to withstand more forces.
Conclusion
The effectiveness of using natural and modern stabilizers as environmentally friendly materials including clay, polyvinyl-acetate, and reinforcement fibers including PP fiber in soil stabilization was regularly investigated through laboratory tests to evaluate the compressive strength and erosion potential of soils. In the present study, a series of experiments have been carried out and it was attempted to investigate the effects of adding the abovementioned modern stabilizers on the soil compressive strength, erosional potential, and microstructures via considering different samples of a real site database depending on the performed laboratory tests. The most important results of the research are presented below.
-
Among different PP fiber contents, the greatest strength was related to the sandy soil reinforced with 0.5% fibers at the 28-day curing time, which increased by 2.12 times, as compared to the sample with no fibers.
-
Among different PVAc contents, in the optimum state, the greatest strength was related to the sandy soil stabilized with 1.2% PVAc at the 28-day curing time, which increased 2.86 times, as compared to the sample with no PVAc.
-
In all curing times, the PGSS improved by combining PVAc and clay and performed better in terms of mechanical strength than the sample improved by combining PP fiber and clay. On the other hand, in terms of erosion resistance, the combination of clay and fiber performed better than the combination of clay and PVAc did.
-
Among different clay contents, in the optimum state, the greatest strength was related to the sandy soil stabilized with 20% clay at the 28-day curing time, which increased 4.5 times, as compared to the sample with no clay.
-
Among different samples reinforced by fiber, the highest ERI is related to the samples with 0.5% fibers at the 28-day curing time, which increased by 6%, as compared to the sample with no fibers.
-
The highest ERI for PVAc treated samples is related to the samples with 1.2% PVAc at the 28-day curing time, which increased by 3%, as compared to the sample with no PVAc.
-
The highest ERI for clay treated samples is related to the samples with 20% clay at the 28-day curing time, which was 4.28 and increased by 10%, as compared to the sample with no clay.
-
Finally, the results obtained from SEM analysis showed that the stabilized and reinforced sand sample became denser as the content of the amendments increased, making it a harder material to withstand more forces.
Data availability
All data, models, and code generated or used during the study appear in the submitted article.
References
Adabi M, Darvishan E, Eyvazi G, Jahanbaksh Motlagh H (2022) Geoenvironmental Application of Novel Persian Gum Biopolymer in Sandy Soil Stabilization. Arabian Journal for Science and Engineering 2022 47:10 47:12915–12929. https://doi.org/10.1007/S13369-022-06645-4
Al-Bared MAM, Marto A (2017) A review on the geotechnical and engineering characteristics of marine clay and the modern methods of improvements. Malaysian J Fundam Appl Sci 13:825–831. https://doi.org/10.11113/mjfas.v13n4.921
Anagnostopoulos CA (2015) Strength properties of an epoxy resin and cement-stabilized silty clay soil. Appl Clay Sci 114:517–529. https://doi.org/10.1016/j.clay.2015.07.007
ASTM D (2000) 2166 (1985) Standard Test Method for for Unconfined Compressive Strength of Cohessive Soil. In: American society for testing and materials
ASTM D (2012) Standard test methods for laboratory compaction characteristics of soil using standard effort. ASTM D698
ASTM D4253–14 (2014) Standard Test Methods for Maximum Index Density and Unit Weight of Soils Using a Vibratory Table. American Society for Testing and Materials
ASTM D4254–14 (2014) Standard Test Methods for Minimum Index Density and Unit Weight of Soils and Calculation of Relative Density. American Society for Testing and Materials
ASTM D4318, ASTM D 4318–10 (2005) Standard Test Methods for Liquid Limit, Plastic Limit, and Plasticity Index of Soils. Report 04:1–14. https://doi.org/10.1520/D4318-10.
Bai Y, Liu J, Song Z et al (2019) Unconfined Compressive Properties of Composite Sand Stabilized with Organic Polymers and Natural Fibers. Polymers (basel) 11:1576
Bell F (1993) Engineering treatment of soils. CRC Press
Bobrowsky PT, Marker B (2018) Encyclopedia of Engineering Geology. Springer Cham
Bozyiğit I, Tanrinian N, Karakan E et al (2017) Dynamic behavior of a clayey sand reinforced with polypropylene fiber. Acta Phys Pol A 132:674–678
Bu F, Liu J, Bai Y et al (2019) Effects of the Preparation Conditions and Reinforcement Mechanism of Polyvinyl Acetate Soil Stabilizer. Polymers (basel) 11:506. https://doi.org/10.3390/polym11030506
Cai G dong, Chen L, Teng Y zong, et al (2022) Investigation on Drained Mechanical Properties of Sandy Soil after Internal Erosion with an Erosion-Stress Coupling Apparatus. KSCE Journal of Civil Engineering 1–15. https://doi.org/10.1007/s12205-022-0145-y
Chen M, Shen SL, Arulrajah A et al (2015) Laboratory evaluation on the effectiveness of polypropylene fibers on the strength of fiber-reinforced and cement-stabilized Shanghai soft clay. Geotext Geomembr 43:515–523. https://doi.org/10.1016/j.geotexmem.2015.05.004
Cherifi BI, Belbachir M, Rahmouni A (2021) Green anionic polymerization of vinyl acetate using Maghnite-Na+ (Algerian MMT): synthesis characterization and reactional mechanism. Discover Chemical Engineering 1:1–14. https://doi.org/10.1007/s43938-021-00005-8
Consoli NC, Arcari Bassani MA, Festugato L (2010) Effect of fiber-reinforcement on the strength of cemented soils. Geotext Geomembr 28:344–351. https://doi.org/10.1016/j.geotexmem.2010.01.005
dos Santos ACS, Henrique HM, Cardoso VL, Reis MHM (2021) Slow release fertilizer prepared with lignin and poly(vinyl acetate) bioblend. Int J Biol Macromol 185:543–550. https://doi.org/10.1016/j.ijbiomac.2021.06.169
Gerard P, Mahdad M, Robert McCormack A, François B (2015) A unified failure criterion for unstabilized rammed earth materials upon varying relative humidity conditions. Constr Build Mater 95:437–447. https://doi.org/10.1016/j.conbuildmat.2015.07.100
Ghiassian H, Poorebrahim GR (2005) Improvement of Strength Parameters of Sand Reinforced with Polymeric Fibers. Journal of Advanced Materials in Engineering (esteghlal) 23:113–131
Hamidi A, Hooresfand M (2013) Effect of fiber reinforcement on triaxial shear behavior of cement treated sand. Geotext Geomembr 36:1–9. https://doi.org/10.1016/j.geotexmem.2012.10.005
Hejazi SM, Sheikhzadeh M, Abtahi SM, Zadhoush A (2012) A simple review of soil reinforcement by using natural and synthetic fibers. Constr Build Mater 30:100–116
Homauoni ZJ, Yasrobi SS (2011) Stabilization of Dune Sand with Poly(Methyl Methacrylate) and Polyvinyl Acetate Using Dry and Wet Processing. Geotech Geol Eng 29:571–579. https://doi.org/10.1007/s10706-011-9404-2
Huang W, Geng X, Li J et al (2022) Molecular dynamics study on the adsorption and modification mechanism of polymeric sand-fixing agent. Polymers (basel) 14:3365. https://doi.org/10.3390/polym14163365
Jiang P, Chen L, Li N, et al (2022) Study on the mechanical properties of fiber-modified iron tailings stabilized by lime and fly ash based on energy analysis. Case Studies in Construction Materials 17:e01638. https://doi.org/10.1016/j.cscm.2022.e01638
Karakan E (2018) Factors Effecting the Shear Strength of Geotextile Reinforced Compacted Clays. Dokuz Eylül Üniversitesi Mühendislik Fakültesi Fen ve Mühendislik Dergisi 20:725–742. https://doi.org/10.21205/deufmd
Kumar A, Gupta D (2016) Behavior of cement-stabilized fiber-reinforced pond ash, rice husk ash-soil mixtures. Geotext Geomembr 44:466–474. https://doi.org/10.1016/j.geotexmem.2015.07.010
Kumari D, Xiang W-N (2019) Review on biologically based grout material to prevent soil liquefaction for ground improvement. Int J Geotech Eng 13:48–53
Lawer AK, Ampadu SIK, Owusu-Nimo F (2021) The effect of randomly distributed natural fibers on some geotechnical characteristics of a lateritic soil. SN Appl Sci 3:1–12. https://doi.org/10.1007/s42452-021-04634-0
Liu J, Chen Z, Song Z et al (2018) Tensile behavior of polyurethane organic polymer and polypropylene fiber-reinforced sand. Polymers (basel) 10:499
Liu J, Chen Z, Zeng Z et al (2020b) Influence of polyurethane polymer on the strength and mechanical behavior of sand-root composite. Fibers and Polymers 21:829–839
Liu J, Feng Q, Wang Y, et al (2017) The effect of polymer-fiber stabilization on the unconfined compressive strength and shear strength of sand. Advances in Materials Science and Engineering 2017:
Liu J, Bai Y, Song Z, et al (2020a) Stabilization of sand using different types of short fibers and organic polymer. Constr Build Mater 253:119164. https://doi.org/10.1016/j.conbuildmat.2020.119164
Maragos JE (1993) Impact of coastal construction on coral reefs in the US affiliated pacific Islands. Coast Manag 21:235–269
Marandi SM, Bagheripour MH, Rahgozar R, Zare H (2008) Strength and ductility of randomly distributed palm fibers reinforced silty-sand soils. Am J Appl Sci 5:209–220
Masoumi E, Forooshani SMA, Nian FA (2013) Problematic soft soil improvement with both polypropylene fiber and polyvinyl acetate resin. Geotech Geol Eng 31:143–149
Mehenni A, Cuisinier O, Masrouri F (2016) Impact of lime, cement, and clay treatments on the internal erosion of compacted soils. J Mater Civ Eng 28:4016071
Memar M, Zomorodian SMA, Vakili AH (2020) Effect of pile cross-section shape on pile group behaviour under lateral loading in sand. International Journal of Physical Modelling in Geotechnics 20:308–319
MolaAbasi H, Semsani SN, Saberian M, et al (2020) Evaluation of the long-term performance of stabilized sandy soil using binary mixtures: A micro-and macro-level approach. J Clean Prod 122209
Otoko GR (2014) A review of the stabilization of problematic soils. International Journal of Engineering and Technology Research 2:1–6
Park S-S (2009) Effect of fiber reinforcement and distribution on unconfined compressive strength of fiber-reinforced cemented sand. Geotext Geomembr 27:162–166
Rezaeimalek S, Nasouri A, Huang J et al (2017) Comparison of short-term and long-term performances for polymer-stabilized sand and clay. Journal of Traffic and Transportation Engineering (english Edition) 4:145–155
Salimi M, Ghorbani A (2020) Mechanical and compressibility characteristics of a soft clay stabilized by slag-based mixtures and geopolymers. Appl Clay Sci 184:105390. https://doi.org/10.1016/j.clay.2019.105390
Salimi M, Ilkhani M, Vakili AH (2018) Stabilization treatment of Na-montmorillonite with binary mixtures of lime and steelmaking slag. International Journal of Geotechnical Engineering 1–7. https://doi.org/10.1080/19386362.2018.1439294
Shaikh ND (2021) Effect of Kaolinite Clay and Different Sand Gradation Mixture on Compaction Parameters. In: Lecture Notes in Civil Engineering. Springer Science and Business Media Deutschland GmbH, pp 495–507
Standard A (2007) D422–63 (2007) Standard test method for particle-size analysis of soils. ASTM International, West Conshohocken Doi 10:1520
Sun X, Miao L, Wang H et al (2022) Bio-cementation for the mitigation of surface erosion in loess slopes based on simulation experiment. J Soils Sediments 22:1804–1818. https://doi.org/10.1007/s11368-022-03190-3
Tang C, Shi B, Gao W et al (2007) Strength and mechanical behavior of short polypropylene fiber reinforced and cement stabilized clayey soil. Geotext Geomembr 25:194–202. https://doi.org/10.1016/j.geotexmem.2006.11.002
Tran KQ, Satomi T, Takahashi H (2018) Effect of waste cornsilk fiber reinforcement on mechanical properties of soft soils. Transportation Geotechnics 16:76–84. https://doi.org/10.1016/j.trgeo.2018.07.003
Vakili AH, Salimi M, Shamsi M (2021) Application of the dynamic cone penetrometer test for determining the geotechnical characteristics of marl soils treated by lime. Heliyon 7:e08062
Vakili AH, Salimi M, Lu Y et al (2022) Strength and post-freeze-thaw behavior of a marl soil modified by lignosulfonate and polypropylene fiber: an environmentally friendly approach. Constr Build Mater 332:127364
Vakili AH, Ghasemi J, bin Selamat MR, et al (2018) Internal erosional behaviour of dispersive clay stabilized with lignosulfonate and reinforced with polypropylene fiber. Constr Build Mater 193:405–415. https://doi.org/10.1016/j.conbuildmat.2018.10.213
Vakili AH, Selamat MR Bin, Salimi M, Gararei SG (2019) Evaluation of pozzolanic Portland cement as geotechnical stabilizer of a dispersive clay. International Journal of Geotechnical Engineering 1–8
Wan CF, Fell R (2004) Investigation of rate of erosion of soils in embankment dams. J Geotech Geoenviron Eng 130:373–380
Wang L, Li X, Cheng Y, Bai X (2018) Effects of coal-metakaolin on the properties of cemented sandy soil and its mechanisms. Constr Build Mater 166:592–600
Wang Y, Frost JD, Murray J (2000) Utilization of recycled fiber for soil stabilization. In: Proceedings of the Fiber Society Meeting. pp 17–19
Wang Z, Wang Q, Zhao W, et al (2022) Influence of carlin-type gold mine tailings addition on the synthesis temperature, alkali-resistant performance, and hydration mechanism of Portland cement. Constr Build Mater 359:129458. https://doi.org/10.1016/j.conbuildmat.2022.129458
Xiao Y, Tong L, Che H, et al (2022) Experimental studies on compressive and tensile strength of cement-stabilized soil reinforced with rice husks and polypropylene fibers. Constr Build Mater 344:128242. https://doi.org/10.1016/j.conbuildmat.2022.128242
Yang J, Yin Z, Laouafa F, Hicher P (2020) Three-dimensional hydromechanical modeling of internal erosion in dike-on-foundation. Int J Numer Anal Methods Geomech 44:1200–1218. https://doi.org/10.1002/nag.3057
Yang Y, Xu C, Indiketiya S (2018) Laboratory Dissolution Experiments of Internal Erosion in Sandy Soil: Underground Cavities and Piping. In: Proceedings of GeoShanghai 2018 International Conference: Multi-physics Processes in Soil Mechanics and Advances in Geotechnical Testing. Springer Singapore, pp 244–251
Yang Y, Wu-wei M, Min Y (2021) Triaxial Experiments on Sandy Soil Subjected to Internal Erosion. Journal of Yangtze River Scientific Research Institute 38:114. https://doi.org/10.11988/CKYYB.20200034
Yilmaz E, Belem T, Benzaazoua M (2015) Specimen size effect on strength behavior of cemented paste backfills subjected to different placement conditions. Eng Geol 185:52–62
Yu H, Huang X, Ning J et al (2016) Improving performance of soil stabilizer by scientific combining of industrial wastes. Geomech Eng 10:247–256
Zhao Y, Xiao Z, Fan C, et al (2020) Comparative mechanical behaviors of four fiber-reinforced sand cemented by microbially induced carbonate precipitation. Bulletin of Engineering Geology and the Environment 1–12
Zhu X, Liu J, Xue J, et al (2022) Effect of Curing Condition on the Compressive Mechanical Behavior of Clayey Soil Stabilized with Liquid Polymer. Int J Polym Sci 2022:. https://doi.org/10.1155/2022/9031369
Acknowledgements
The authors would like to thank Mr. Mohammad Shamsi for their constructive help in revising the paper.
Funding
The authors declare that no funds, grants, or other support were received during the preparation of this manuscript.
Author information
Authors and Affiliations
Contributions
Amir Hossein Vakili: Conceptualization, Validation, Investigation, Writing - Review & Editing, Visualization, Formal analysis, Supervision. Shahram Rastegar: Formal analysis, Methodology, Writing - Original Draft, Investigation. Hossein Golkarfard: Resources, Methodology, Investigation, Writing - Original Draft. Mahdi Salimi: Formal analysis, Methodology, Investigation, Writing - Review & Editing. Zahra Izadneshan: Writing - Original Draft, Investigation.
Corresponding author
Ethics declarations
Conflcit of interest
The authors have no relevant financial or non-financial interests to disclose.
Disclosure statement
No potential conflict of interest was reported by the authors.
Additional information
Publisher's Note
Springer Nature remains neutral with regard to jurisdictional claims in published maps and institutional affiliations.
Rights and permissions
Springer Nature or its licensor (e.g. a society or other partner) holds exclusive rights to this article under a publishing agreement with the author(s) or other rightsholder(s); author self-archiving of the accepted manuscript version of this article is solely governed by the terms of such publishing agreement and applicable law.
About this article
Cite this article
Vakili, A.H., Rastegar, S., Golkarfard, H. et al. Effect of polypropylene fibers on internal erosional behavior of poorly graded sandy soil stabilized with the binary mixtures of clay and polyvinyl acetate. Environ Earth Sci 82, 294 (2023). https://doi.org/10.1007/s12665-023-10961-9
Received:
Accepted:
Published:
DOI: https://doi.org/10.1007/s12665-023-10961-9